Introduction
When the oocytes in antral follicles (AF) acquire developmental competence, they can be used for in vitro embryo production in large domesticated animals, but their number on the surface of ovaries is limited. In contrast with AFs, there are huge numbers of oocytes in premature follicles in the ovaries, and they are a potential resource for embryo production. Although live offspring have been obtained from immature oocytes derived from advanced secondary stage follicles in cows, sheep, and goats (Gutierrez et al., Reference Gutierrez, Ralph, Telfer, Wilmut and Webb2000, Arunakumari et al., Reference Arunakumari, Shanmugasundaram and Rao2010; Magalhães et al., Reference Magalhães, Duarte, Araújo, Brito, Soares, Lima, Lopes, Campello, Rodrigues and Figueiredo2011; Luz et al., Reference Luz, Araújo, Duarte, Celestino, Silva, Magalhães-Padilha, Chaves, Brito, Almeida, Campello, Feltrin, Bertolini, Santos and Figueiredo2012), the developmental efficiency of oocytes grown in vitro is still low.
Oocyte growth is supported by complex autocrine and paracrine interactions with the surrounding granulosa cells (Orisaka et al., Reference Orisaka, Tajima, Tsang and Kotsuji2009; Binelli & Murphy, Reference Binelli and Murphy2010). Owing to the lower ability of oocytes to take up glucose, the surrounding cells take up glucose and provide energy substrates to oocytes via gap junctional communication (Downs & Utecht, Reference Downs and Utecht1999; Dalton et al., Reference Dalton, Szabadkai and Carroll2014; Gu et al., Reference Gu, Liu, Gu, C, Moley and Wang2014). Adenosine triphosphate (ATP) is important for oocyte growth, accumulating transcripts and protein, and epigenetic modification of histone and DNA, as well as meiotic maturation (Gu et al., Reference Gu, Liu, Gu, C, Moley and Wang2014). Most ATP is derived from mitochondrial oxidative phosphorylation, and the presence of surrounding cells plays a key role in maintaining ATP levels in oocytes (Dalton et al., Reference Dalton, Szabadkai and Carroll2014). Furthermore, histone acetylation levels in H4K12 increase during the growth of bovine oocytes (Maalouf et al., Reference Maalouf, Alberio and Campbell2008; Racedo et al., Reference Racedo, Wrenzycki, Lepikhov, Salamone, Walter and Niemann2009) and the acetylation level changes depending on maternal conditions including advanced maternal age (Manosalva & González, Reference Manosalva and González2009). Acetylation of cellular protein depends on acetyl-CoA, which originates from citrate and pyruvate, as reported by Morrish et al. (Reference Morrish, Noonan, Perez-Olsen, Gafken, Fitzgibbon, Kelleher, Van Gilst and Hockenbery2010), who also reported that the acetyl residues of H4K16 in cells are derived from glucose. From these reports it can be speculated that energy capacity in oocytes depends on the number of granulosa cells surrounding the oocytes, and that energy efficiency reflects ATP and acetylation levels in the oocytes. However, no direct relationship between the number of granulosa cells and ATP and the acetylation level of H4K12 in oocytes grown in vitro has been reported.
The proliferation of granulosa cells increases with the development of follicles from early antral follicles (EAFs) to AFs. In pigs, the oocytes in EAFs and AFs are associated with 8000 and 1,390,000 granulosa cells, respectively. However, by culturing the porcine oocytes and granulosa cell complexes (OGCs) derived from EAFs, the number of granulosa cells in the OGCs increased but did not reach one-tenth of the level contained in the AFs (Oi et al., Reference Oi, Tasaki, Munakata, Shirasuna, Kuwayama and Iwata2015). This finding suggests that the small number of granulosa cells in OGCs grown in vitro is a limiting factor for inefficient growth of oocytes in vitro. We have recently reported that when granulosa cells are co-incubated with denuded porcine oocytes, these two types of cells form OGCs, and the oocytes developed in the reconstructed OGCs acquire the ability to develop to the blastocyst stage (Oi et al., Reference Oi, Tasaki, Munakata, Shirasuna, Kuwayama and Iwata2015). Based on these reports, we hypothesised that the addition of granulosa cells to the culture condition (GC-add culture system) supports the development of OGCs and the growth of oocytes, and that the use of this culture system makes it possible to examine the relationship between ATP and the acetylation of oocytes grown in vitro and the number of granulosa cells.
In the present study, we collected OGCs from EAFs on the surface of cow ovaries, and cultured the OGCs with additional granulosa cells collected from other cohort OGCs. We then examined the effect of the GC-add culture system on oocyte growth, ATP content, and histone acetylation.
Materials and methods
Reagents and media
All drugs were purchased from Nacalai Tesque INC (Kyoto, Japan) unless otherwise stated. TCM199 medium (Gibco BRL, Paisley, UK) used for the in vitro culture (IVC) of OGCs was supplemented with 5.56 mM glucose (final concentration 11.2 mM), 0.1 mM pyruvic acid, 1 µg/ml estradiol-17β (E2), 0.02 milli-arbitrary units (mAU)/ml follicle-stimulating hormone (FSH; Kawasaki Mitaka Pharmaceutical Co., Kawasaki, Japan), 4% polyvinylpyrrolidone (PVP) (360K), 4 mM hypoxanthine, and 10% fetal calf serum (FCS; 5703H, ICN, Costa Mesa, CA, USA). The TCM 199 medium used for the in vitro maturation (IVM) of oocytes contained 5% FCS.
Collection of ovaries and oocytes from EAFs
Ovaries were collected from Japanese black cows aged 28–50 months at a slaughterhouse and transported to the laboratory in phosphate-buffered saline (PBS) containing antibiotics, at 30°C within 4 h. The ovaries were wiped with 70% ethanol. The ovarian surface was then sliced and EAFs (400–700 μm in diameter) were collected. OGCs were retrieved from the EAFs using an 18G needle connected to a 1 ml syringe.
Culture of OGCs and in vitro maturation and fertilization
OGCs were washed in culture medium, individually transferred to wells containing 200 μl of medium (96-well plate, Becton, Dickinson and Company, Franklin Lakes, NJ, USA), and cultured for 16 days. Half of the medium was replaced with fresh medium at 4-day intervals, and antrum formation (Fig. 1) was examined. After the culture period, the OGCs that had formed an antrum were subjected to IVM for 23 h. In vitro fertilization was conducted as reported previously (Iwata et al., Reference Iwata, Goto, Tanaka, Sakaguchi, Kimura, Kuwayama and Monji2011). To determine the rate of maturation and fertilization, zygotes were fixed in 4% paraformaldehyde, permeabilized in 0.2% Triton X-100, and mounted on glass slides using an antifade reagent containing 4′,6-diamidino-2-phenylindole (DAPI) (ProLong Gold Antifade Reagent with DAPI; Invitrogen, OR, USA). Oocytes with two polar bodies and pronuclei were defined as normally fertilized oocytes.
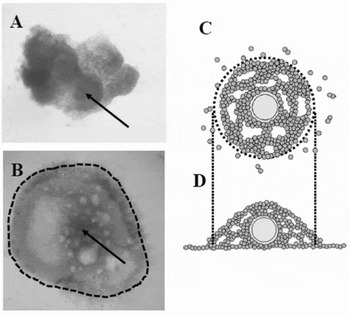
Figure 1 Representative pictures and images of oocyte–granulosa cell complexes (OGCs) cultured in vitro. OGCs were collected from early antral follicles (EAFs) (A); and cultured for 16 days, after which OGCs forming pseudo-antrum cavities were selected (B, picture; C, upper view; D, side view). Dotted lines indicate OGCs used for experiments. Arrows in (A) and (B) indicates the oocyte.
Preparation of granulosa cell mass (GC mass) from EAFs and addition of the GCs mass to OGCs
The OGCs were retrieved from EAFs and the oocytes were removed from the OGCs using a fine- pulled Pasteur pipette to obtain GC mass from which an equal area of GC mass (x-axis, 250 µm; y-axis, 250 µm; approximately half of GC mass collected from an OGC) was stripped. The OGCs were co-incubated with the GC mass (termed the GC-add culture system henceforth) and OGCs without GC supplementation (termed as control henceforth) (Fig. 2). The amount of GCs to be added to the OGCs was determined in preliminary experiments in which the addition of a large GC mass (x-axis, 500 µm; y-axis, 250 µm) resulted in low antrum formation. During the co-incubation of OGCs with the GC mass, the supplemented GC mass was merged with the OGCs and contributed to OGC development (Fig. 3).

Figure 2 Oocyte–granulosa cell complexes (OGCs) were collected from early antral follicles (EAFs), and the oocytes were removed from the OGCs to obtain granulosa cell mass. The granulosa cell mass was processed to make up an equal volume using a microscope equipped with scales. The granulosa cells were added to the OGCs and co-incubated for 16 days (GC-add culture system). OGCs without additional granulosa cells were referred to as the control culture system.

Figure 3 Location of granulosa cell (GC) mass added to oocyte granulosa cell complexes (OGCs). GC mass was collected from the OGCs in the early antral follicles (EAFs) and was stained with Cell Tracker™ (Molecular Proves: C10094) prior to co-incubation. After 7 days of culture, the OGCs were examined under a fluorescence microscope (Keyence, Tokyo, Japan) to observe the location of the GC mass. (A) Bright field. (B) Arrow indicates GC added to the OGCs.
Measurement of oocyte diameter
At the end of the culture period, oocytes were denuded from the surrounding cells using a narrow-pulled glass pipette and transferred to TCM199 medium containing 3 mg/ml bovine serum albumin (BSA) covered by mineral oil. The x-axis and y-axis of the diameter were measured using a digital microscope (Keyence, Tokyo, Japan), and the average of the two values was regarded as the diameter of the oocyte.
Measurement of granulosa cell number
At the end of the culture period, OGCs forming the antrum (Fig. 1 B) were selected and oocytes were removed from the OGCs using a fine narrow-pulled glass pipette. The remaining GC complexes were then transferred to the Accumax cell dissociation solution (Innovative Cell Technologies, Inc., San Diego, CA, USA) and pipetted vigorously. The cell number was calculated by the volume of the cellular suspension and the concentration was determined using a haemocytometer.
Measurement of the mitochondrial DNA (mtDNA) copy number of the oocytes
Oocytes grown in vitro were collected from OGCs forming the antrum and were lysed in 6 μl lysis buffer (20 mM Tris, 0.4 mg/ml proteinase K, 0.9% Nonidet-P40, and 0.9% Tween 20) at 55°C for 30 min followed by 95°C for 5 min. The mtDNA copy number was then determined by real-time polymerase chain reaction (PCR) using ND-5 region-specific primer sets, as described previously (Iwata et al. Reference Iwata, Goto, Tanaka, Sakaguchi, Kimura, Kuwayama and Monji2011; Endo et al. Reference Endo, Kimura, Kuwayama, Monji and Iwata2012).
Detection of acetylated H4K12 by fluorescence immunostaining
Oocytes grown in vitro were collected from OGCs forming the antrum, and were subjected to immuno-staining, which was carried out as described previously (Sato et al., Reference Sato, Itami, Tasaki, Takeo, Kuwayama and Iwata2014). The primary and secondary antibodies used for this procedure were rabbit polyclonal anti-H4K12 (1:500; Novus International Saint Charles, MO, USA) and fluorescein-conjugated goat anti-rabbit IgG (1:1000; Cell Signaling Technology Inc., Beverly, MA, USA), respectively. The oocytes were mounted on glass slides using an antifade reagent containing DAPI (ProLong Gold Antifade Reagent with DAPI; Invitrogen, OR, USA). We attempted to ensure that the equatorial diameter of oocytes was consistent using a stereomicroscope equipped with a scale. The expression of acetylated H4K12 was observed using a fluorescence digital microscope (BZ-8000; Keyence, Tokyo, Japan: for green fluorescence, sensitivity ISO 200, exposure 1/6 s; and for blue fluorescence, sensitivity ISO 200, exposure 1/2 s). Fluorescence images of the oocytes were captured, and the fluorescence intensity of the germinal vesicle was measured using Image J software (ImageJ, US National Institutes of Health, Bethesda, MD, USA).
ATP measurement
The oocytes were denuded of the surrounding GCs and the ATP content of the individual denuded oocytes was measured. The ATP levels were determined by measuring luminescence generated from the ATP-dependent luciferin–luciferase reaction, as described previously (Sato et al., Reference Sato, Itami, Tasaki, Takeo, Kuwayama and Iwata2014).
Experimental design
Experiment 1
We determined the effects of the GC-add culture system on antrum formation of the OGCs and the diameter, meiotic maturation rate, and fertilization rate of the oocytes following IVM. The OGCs collected from the EAFs were cultured for 16 days and the number of OGCs forming an antrum was determined. Moreover, the diameter of the oocytes enclosed in the OGCs was measured. In addition, the OGCs were subjected to in vitro maturation and fertilization. The number of oocytes that reached the M2 stage and the number of oocytes that had been normally fertilized were determined. Ten OGCs were cultured and the process was repeated 17 times. In the first seven trials, the diameters of the oocytes grown in vitro were measured; in the next five trials, the number of oocytes that reached the M2 stage was determined; and in the last five trials, the number of oocytes that had been normally fertilized was determined.
Experiment 2
We examined the effect of the GC-add culture system on ATP content in the oocytes grown in vitro and the granulosa cell number of the OGCs. The OGCs were collected from EAFs and cultured for 16 days, after which the OGCs that formed an antrum were selected; the ATP content in the oocytes and the number of GCs in the OGCs were determined. In total, 40 OGCs were cultured. The granulosa cell number and ATP content of 16 oocytes (control) and 22 oocytes (GC-add culture system) along with the corresponding GCs were determined.
Experiment 3
Because the ATP content in the oocytes grown in the GC-add culture system was higher than that in the control culture system, the mtDNA copy number in the oocytes grown in vitro was compared between the two culture systems. The OGCs derived from EAFs were divided into two groups, and cultured for 16 days in GC-add or control culture systems, after which the oocytes were collected from the OGCs forming an antrum and the mtDNA copy number in the oocyte was compared between the two oocytes groups.
Experiment 4
The OGCs derived from EAFs were divided into two groups, and cultured for 16 days in GC-add or control culture systems, after which the acetylation level of H4K12 in the oocytes was compared between the two culture systems. Approximately 15 control OGCs and 15 GC-add OGCs were cultured, and the oocytes were collected from the OGCs forming an antrum; the experiments were repeated on 3 sequential days using differential ovary series. In total, 50 OGCs were used and oocytes grown in vitro were fixed and pooled in PBS. In total, 23 and 38 oocytes from the control and GC-add culture systems, respectively, were subjected to immunostaining against acetylated H4K12 (Ace-H4K12) in which the average fluorescence intensity of the oocytes derived from the control culture system was defined as 1.0.
Statistical analysis
The rate of antrum formation, the oocyte diameter, the number of GCs, the ATP content, the mtDNA number, and the acetylation level of the oocytes were compared using Student's two-tailed t-test. Maturation and fertilization rates were examined using the chi-squared test. The rate of antrum formation was arcsine transformed prior to analysis. The correlation between the GC number and ATP was determined using SPSS software (version, 17.0, Chicago, IL, USA). A P-value of less than 0.05 was considered to indicate statistical significance.
Results
Experiment 1
The GCs formed a pseudo-antrum during the in vitro culture period (Fig. 1), and the rate of antrum formation was higher in the GC-add culture system than in the control group (P < 0.05). The diameter of the oocytes grown in the GC-add culture system was 119.6 μm, which was significantly greater than that in the control culture system (116.7 µm, Table 1). The number of oocytes that reached the M2 stage and the number that had been normally fertilized were higher in the GC-add group compared with the control, but the difference was not significant.
Table 1 Comparison of oocytes grown in vitro between GC-add and control culture systems

Experiment 2
The total number of granulosa cells constituting the OGCs developed in the GC-add culture system was 24,091, which was significantly greater than in the control groups (17,375, P < 0.05). Furthermore, the oocytes grown in the GC-add culture system contained high ATP content compared with those in the control culture system (2.83 versus 2.37 pM, P < 0.05, Table 2). Figure 4 shows the relationship between the GC number and the ATP content in the oocytes. Significantly positive correlation was observed (r = 0.44, P < 0.01) between the two factors.
Table 2 Effect of GC-add culture system on ATP content of oocytes grown in vitro and granulosa cell number of OGCs

Data are presented as mean ± standard error of the mean (SEM). a , bP < 0.05.

Figure 4 Correlation between the number of granulosa cells and ATP content in oocytes grown in vitro. Oocyte–granulosa cell complexes (OGCs) were cultured with or without additional granulosa cell mass for 16 days, and then the ATP content in the oocytes was determined. Significantly positive correlation was observed between ATP content in the oocytes and the number of granulosa cells (r = 0.44, P < 0.01). White circles: control culture system; black circles: GC-add culture system.
The dots representing the GC-add culture system shifted to the upper right of those for the control culture system.
Experiment 3
The mtDNA copy numbers in the oocytes grown in vitro in the GC-add culture and control culture systems were 183,346 and 211,763 per oocyte, respectively. The values did not differ significantly (Fig. 5).
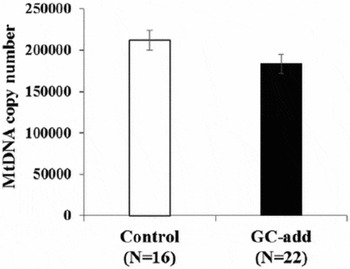
Figure 5 Mitochondrial DNA (mtDNA) number in oocytes developed in the granulosa cell (GC)-add culture system or the control culture system. Oocyte–granulosa cell complexes (OGCs) derived from early antral follicles (EAFs) were cultured for 16 days and the mtDNA copy number in the oocytes grown in vitro was determined. y-axis: mtDNA copy number; white bar: oocytes grown under the control culture system; black bar: oocytes grown under the GC-add culture system; bar: standard error of the mean (SEM).
Experiment 4
Acetylated H4K12 was detected in the germinal vesicle (Fig. 6 A, B) and the level of acetylation was higher in oocytes developed in the GC-add culture system than in those developed in the control culture system (Fig. 6 C).

Figure 6 Acetylation of H4K12 in oocytes grown in vitro. Oocyte–granulosa cell complexes (OGCs) derived from early antral follicles (EAFs) were cultured for 16 days and the acetylation level of oocytes grown in vitro was determined by immunostaining. (A, B) Picture of oocytes grown under the control culture system and the GC-add culture system, respectively. (C) Comparison of fluorescence intensity of oocytes stained against Ace-H4K12. White bar: oocytes grown under the control culture system. Black bar: oocytes grown under the GC addition culture system, a , bP < 0.05. Bar: standard error of the mean (SEM).
Discussion
The present study showed that the co-incubation of OGCs with additional GCs improves the development of OGCs with both increased antrum formation and number of granulosa cells. Addition of GC mass to the culture medium increases the diameter, ATP content, and acetylation level of H4K12 in the oocytes grown in vitro.
Oocyte growth requires sufficient energy to complete many crucial cellular events including protein and transcript accumulation and epigenetic modification. Moreover, oocyte energy homeostasis is supported by bidirectional communication with the surrounding somatic cells (Albertini et al., Reference Albertini, Combelles, Benecchi and Carabatsos2001; Matzuk et al., Reference Matzuk, Burns, Viveiros and Eppig2002). Owing to the lower glucose-uptake capacity of oocytes, GCs take up glucose and transport the metabolites to oocytes through gap junctions. Because the total number of GCs is much lower in OGCs developed in vitro than in those developed in vivo, increasing the number of surrounding cells is a potential way to improve oocyte development. Some options to increase the number of GC include stimulation of cellular proliferation by cytokines, artificial addition of granulosa cells, and improvement of culture conditions so that they are more suitable for cellular proliferation. To examine the effect of GC number on oocytes, we selected to employ artificial addition of granulosa cells, because cytokines and modification of culture conditions profoundly affect both granulosa cells and oocytes, and may hinder the evaluation of the intrinsic effect of cellular number on oocytes. The present study shows that the addition of granulosa cells to the culture medium increased the development of OGCs with high antrum formation rate and granulosa cell number at the end of the culture period, and the diameter of the oocytes enclosed in the OGCs was high for the GC-add culture system (Table 1). These results indicate that the GC number is closely related to antrum formation and oocyte growth in vitro. In addition, we examined the hypothesis that the number of GCs affects ATP and acetylation levels in oocytes, and found a significantly positive relationship between the number of granulosa cells and the ATP content in oocytes grown in vitro (Fig. 4). Furthermore, we discovered that the GC-add culture system increased ATP content in oocytes (Table 2). The notion that ATP in oocytes originates from mitochondria is supported by Itami et al. (Reference Itami, Shiratsuki, Shirasuna, Kuwayama and Iwata2015), who reported that the treatment of oocytes with the mitochondrial un-coupler CCCP causes the ATP content to plummet in oocytes. Thus, we determined whether the GC-add culture system affects mitochondrial number, and found that the mtDNA copy number was similar between the two culture systems. This result suggests that high ATP content in oocytes grown in the GC-add culture system is due to higher mitochondrial activity and not due to the mitochondrial number in the oocytes. The acetylation level of histone H4K12 is reportedly high at the germinal vesicle stage in bovine oocytes (Racedo et al., Reference Racedo, Wrenzycki, Lepikhov, Salamone, Walter and Niemann2009) and the acetylation level in oocytes increases as oocytes grow in mice (Kageyama et al., Reference Kageyama, Liu, Kaneko, Ooga, Nagata and Aoki2007). Moreover, acetyl-coA derived from glucose has been used for human pluripotent stem cell acetylation (Moussaieff et al., Reference Moussaieff, Rouleau, Kitsberg, Cohen, Levy, Barasch, Nemirovski, Shen-Orr, Laevsky, Amit, Bomze, Elena-Herrmann, Scherf, Nissim-Rafinia, Kempa, Itskovitz-Eldor, Meshorer, Aberdam and Nahmias2015). The present study showed that the acetylation level of oocytes grown with a greater number of GCs was high compared with that of oocytes developed in the control culture system. Therefore, it can be deduced that a sufficient energy substrate derived from surrounding GCs resulted in the high ATP content and histone acetylation in oocytes grown in vitro. To the best of our knowledge, our study is the first to demonstrate a relationship between ATP and acetylation levels in oocytes and the number of GCs surrounding the oocytes grown in vitro. Interestingly, in our additional experiments, we collected oocytes from the AFs (3–6 mm in diameter) of young (23–27 months old) and older (>120 months old) cows, and found that the average number of GCs per antral follicle was significantly lower in the older than in the young cows (younger cows: N = 8, 242,278 ± 30,573; older cows: N = 8, 105,701 ± 23,281; P < 0.01, Fig. S1). Furthermore, as reported in mice (Manosalva & González, Reference Manosalva and González2009), we found that the acetylation level of H4K12 in oocytes was lower in older cows than in younger cows (N = 74, 0.85 ± 0.03 versus N = 73, 1.0 ± 0.03, respectively; P < 0.01, Fig. S1). These results suggest that lower GC numbers stem from poor maternal conditions including ageing, which cause insufficient energy conditions for oocytes. Whether this in turn affects chromatin configuration and the epigenetics of the oocytes, remains to be elucidated by further studies.
In conclusion, our study shows that ATP content and acetylation levels in oocytes grown in vitro are profoundly affected by the number GCs.
Acknowledgements
We thank the Kanagawa Meet Center for providing the ovaries.
Financial support
This work was supported by a Grant-in-Aid for Scientific Research C (KAKENHI, grant number: 16K07996) from the Japan society for the Promotion of Science.
Statement of interest
The authors declare that there are no conflicts of interest.
Ethical standards
The ovaries collected at a slaughterhouse were discarded before use, thus this study met with the approval of the ethical committee for animal experiments of the Tokyo University of Agriculture because an ethics statement for our paper was not required.
Supporting information
Additional supporting information is available in the online version of this article at the publisher's website.
Supplementary material
To view supplementary material for this article, please visit http://dx.doi.org/10.1017/S0967199416000198