Introduction
The family Echinochasmidae Odhner 1910 comprises numerous species that parasitize mammals, birds and, less commonly, reptiles in the mature stage (Tkach et al., Reference Tkach, Kudlai and Kostadinova2016). Many of these trematodes are cosmopolitan. For most of them, the taxonomic status is determined based only on the morphology of adult individuals and is not genetically confirmed. There are data in the GenBank with established species affiliation for six Echinochasmus and three Stephanoprora representatives. This leads to certain difficulties with solving the problems of taxonomy and phylogenetic relationships in the Echinochasmidae system for these worms (Tkach et al., Reference Tkach, Kudlai and Kostadinova2016; Besprozvannykh et al., Reference Besprozvannykh, Rozhkovan and Ermolenko2017). In the Russian southern Far East, 13 Echinochasmus and one species of Stephanoprora were recorded. Of these, Stephanoprora chasanensis Besprozvannykh, Rozhkovan, Ermolenko, 2017 and five species of Echinochasmus from this region revealed a natural infection of the first intermediate hosts, and their life cycles were completed in a laboratory (Besprozvannykh, Reference Besprozvannykh1989, Reference Besprozvannykh1991, Reference Besprozvannykh2009, Reference Besprozvannykh2011). In addition, genetic data were obtained for Echinochasmus milvi Yamaguti, Reference Yamaguti1939 and S. chasanensis (Besprozvannykh et al., Reference Besprozvannykh, Rozhkovan and Ermolenko2017).
In the present work, during parasitological studies of freshwater prosobranch molluscs of the family Semisulcospiridae in the Razdolnaya River (Primorsky Region, Russia), we found snails that emitted two types of long-tailed cercariae, which were morphologically similar to Echinochasmidae. Subsequent experimental completion of their life cycles, as well as the study of developmental stages, showed that one of the cercariae belongs to a new species of Stephanoprora, and others are Echinochasmus suifunensis Besprozvannykh, Reference Besprozvannykh1991. Previously, adult E. suifunensis worms have already been obtained from studies of the life cycle of trematodes in the Russian southern Far East (Besprozvannykh, Reference Besprozvannykh1991). For both species, molecular data were obtained for adult worms. In addition, new genetic data were provided for adult worms of E. milvi. To analyse the phylogenetic relationships of Echinochasmidae representatives, we used markers of nuclear (ITS1, ITS2 rDNA regions and 28S rRNA gene) and mitochondrial (cox1 gene) DNA.
Materials and methods
Life cycle and morphology of worms
Long-tailed cercariae of two morphologically different species were isolated from two snails, Parajuga subtegulata Prozorova et Starobogatov (Semisulcospiridae), collected in the Razdolnaya River (Primorsky Region, Russia). Cercariae of one of these species were also found in snails Parajuga amurensis (Gerstfeldt) from the Ussuri River. To determine the second intermediate host, the infected snails of Parajuga emitting cercariae were placed separately in containers (1000 mL volume) together with ten specimens of the freshwater fish Rhodeus sericeus sericeus (Pallas, 1776) in each. After 8 h of exposure, fish from both experiments were placed separately in two aquaria. The fish used in the experiments were caught in an artificial pond. Fifty fish from this pond were previously dissected to confirm the absence of trematode metacercariae. Two fish from each aquarium were dissected on the fourth day to establish the level of infection. Before infection of the definitive host, the remaining fish were dissected on the 25th day after the beginning of the experiment. Metacercariae were found only on the gills of all fish. Fish gills from both experiments were then fed separately to two laboratory chickens. Adult worms were found in the small intestines of the chickens 8 days later. The experiments were carried out at room temperature, from 18 to 22°C.
Measurements of rediae and metacercariae were taken on live specimens. Cercariae were measured after fixation with 4% hot formalin. Adult worms recovered from experimental chickens were fixed with 70% ethanol and then placed in 96% ethanol. Whole mounts were made by staining specimens with alum carmine, dehydrating the worms in a graded ethanol series and clearing in clove oil. Clove oil treatments were followed by mounting specimens in Canada balsam under a coverslip on a glass slide. All measurements are given in micrometres (μm).
In addition to the sexually mature worms obtained in the experiment, the study used trematode slides of E. milvi (No. 20 – Tr, Besprozvannykh, 1989), and E. suifunensis (holotype No. 26 – Tr, paratypes; Nos. 27, 28 – Tr, Besprozvannykh, 1991) from the Zoological Museum (Federal Scientific Center of the East Asia Terrestrial Biodiversity, Far East Branch of the Russian Academy of Sciences, Vladivostok, Russia).
DNA extraction, amplification and sequencing
Using the HotSHOT method (Truett et al., Reference Truett, Heeger, Mynatt, Truett, Walker and Warman2000), DNA samples were extracted from adult worms: four samples of Stephanoprora amurensis sp. nov. and two samples of E. suifunensis from the Razdolnaya River obtained in the experiment, as well as two E. milvi samples from the Komissarovka River obtained by Besprozvannykh et al. (Reference Besprozvannykh, Rozhkovan and Ermolenko2017); these were deposited in the Zoological Museum of Federal Scientific Center of the East Asia Terrestrial Biodiversity, Far East Branch of the Russian Academy of Sciences.
Information about the primers for amplification and sequencing of nuclear markers (ITS1, ITS2 rDNA regions and 28S rRNA gene), the composition of the reaction mixture and the polymerase chain reaction cycling conditions can be found in Tatonova et al. (Reference Tatonova, Besprozvannykh, Katugina, Solodovnik and Nguyen2020). The amplification and sequencing of the partial cox1 gene were performed using the following primers: JB3 (5′-TTT TTT GGG CAT CCT GAG GTT TAT-3′, forward) and JB4.5 (5′-TAA AGA AAG AAC ATA ATG AAA ATG-3′, reverse) (Bowles et al., Reference Bowles, Hope, Tiu, Liu and McManus1993) for the genus Echinochasmus, and CO1-Fw (5′-GGG CAT CCT GAG GTT TAT G-3′, forward) and CO1-Rv (5′-AAC AAA TCA TGA TGC AAA AGG TA-3′, reverse) for S. amurensis sp. nov. (Katokhin et al., Reference Katokhin, Shekhovtsov, Konkow, Yurlova, Serbina and Vodianitskai2008). The annealing temperature was 50 and 55°C for the first and second primer pairs, respectively.
Genetic analysis
The nucleotide sequences were manually assembled in MEGA version 5.03 (Tamura et al., Reference Tamura, Peterson, Peterson, Stecher, Nei and Kumar2011). The p-distances between species were also analysed using the same program. Phylogenetic reconstructions used aligned sequences of 427 and 1151 bp from ITS2 and 28S, respectively, based on the Bayesian inference (BI) method in MrBayes version 3.1.2 (Ronquist and Huelsenbeck, Reference Ronquist and Huelsenbeck2003). The list of samples used in the study is presented in Table 1. According to the Akaike criteria in Modeltest version 3.7 (Darriba et al., Reference Darriba, Taboada, Doallo and Posada2012), TVM + G and GTR + I + G were the optimal models for determining genetic distances for the ITS2 region and 28S rDNA sequences, respectively. The BI analysis was performed using 400 000 and 1 200 000 generations of the Markov chain Monte Carlo test for the ITS2 region and 28S rRNA gene, respectively. This number of generations was sufficient as the s.d. value was <0.01. A total of 25% samples were excluded to construct the consensus trees. The chain was sampled every 100th generation. The sequences of the ITS1 rDNA region and the cox1 mtDNA gene were also used to identify differences between the related species E. suifunensis and E. milvi.
Table 1. List of analysed sequences

Results
Stephanoprora amurensis sp. nov.
Host: Gallus gallus dom. (experimental host).
Site: small intestine.
Intensity of infection: 12 specimens.
First intermediate host: Parajuga subtegulata Prozorova et Starobogatov.
Other first intermediate host: Parajuga amurensis (Gerstfeldt).
Second intermediate host: Rhodeus sericeus sericeus (experimental host).
Site: gills.
Type locality: the Razdolnaya River, Primorsky Region, southern Far East, Russia (43°20′N, 131°47′E).
Other locality: the Ussuri River (right tributary of the Amur River), Primorsky Region, southern Far East, Russia; 45°15′N, 133°30′E.
Type-deposition: holotype No. 143-Tr, paratype No. 144-147-Tr. This material is held in the parasitological collection of the Zoological Museum (Federal Scientific Center of the East Asia Terrestrial Biodiversity, Far East Branch of the Russian Academy of Sciences, Vladivostok, Russia); e-mail: petrova@biosoil.ru. Deposited: 2018.22.09.
Etymology: The species' name refers to the Amur River, in the basin of which the parasite was first discovered.
Adult worm (based on seven specimens; Fig. 1A–C; Table 2)
Body elongated, spined from anterior end to level of posterior testis. Most densely spines from anterior end of body to level of middle of ventral sucker. Oral sucker subterminal. Head-collar with 22 spines, arranged in single row interrupted dorsally. Prepharynx short, pharynx oval, oesophagus longer than prepharynx. Intestinal bifurcation just anterior to ventral sucker. Caeca narrow, reach level of posterior end of vitellarium. Ventral sucker in anterior third of body. Testes two, tandem, elongate, ovoid, in middle third of body. Distance between testes present or absent. Cirrus-sac oval, at median line of body and partly dorsal to ventral sucker. Internal seminal vesicle bipartite. Genital pore between oesophageal bifurcation and anterior margin of ventral sucker. Ovary round or transversely-oval, on median line of body anterior to anterior testis. Uterine seminal receptacle and Mehlis' gland between ovary and anterior testis. Uterus short, in space between caeca, posterior margin of ventral sucker and anterior margin of anterior testis. Vitelline fields of transversely-oval follicles, between middle of anterior testis and posterior end of body, uniting posterior to testes. Vitelline reservoir on median line of body between ovary and anterior testis. Eggs operculated, light yellow. Excretory bladder Y-shaped.

Fig. 1. Stephanoprora amurensis sp. nov.: (A, B) adult worm; (C) head-collar; (D) redia; (E, F) cercaria; (G) body of cercaria and (H) metacercaria.
Table 2. Measurements (μm) of adult worms of the family Echinochasmidae

a Bw/Bl, body width as a percentage of body length; Fo/Bl, length of the forebody as a percentage of body length; Pt/Bl, post-testicular field length as a percentage of body length.
Redia (based on ten specimens; Fig. 1D)
Body sac-shaped, 0.410–0.730 × 0.145–0.156, grey, with collar and lateral appendages. Pharynx 0.056–0.072 × 0.045–0.061. Caeca long, terminate at some distance from posterior extremity of body. Intestinal contents dark grey. Birth pore posterior to collar, 0.13–0.24 from anterior end of body. Redia contains few mature cercariae and several immature cercariae at various stages of development.
Cercaria (based on ten specimens; Fig. 1E–G; Table 3)
Body without spines. Oral sucker, with ten cuticular plates in single row. Prepharynx and pharynx present, oesophagus long, caeca short, terminate at posterior margin of ventral sucker. Intestinal bifurcation anterior to ventral sucker. Ventral sucker at 0.132–0.180 from anterior end of body. Internal edge of ventral sucker with 32 cuticular plates. Undifferentiated genital primordium dorsal to ventral sucker, at level of its posterior margin. Cells of cystogenous glands in two lateral and two median rows. Lateral rows between anterior margin of pharynx and level of middle of ventral sucker, median rows between middle of pharynx and anterior margin of ventral sucker. Two drop-like cells of another type of glands on both sides from prepharynx and by five cells on both sides from oesophagus. Gland ducts located at level of oesophagus open at anterior end of body. Excretory bladder bipartite, with single caudal appendage. Caudal appendage twisted in initial part of tail or elongated, reaches middle of its length. Collecting channels of excretory system with 10–11 granules. Tail large, light grey, contains few small vacuole-like inclusions.
Table 3. Measurements (μm) of cercariae and metacercariae of the family Echinochasmidae

Metacercaria (based on five specimens; Fig. 1H; Table 3)
Cyst oval, with thin wall. Body surface with few small spines. Head collar with 22 spines. Prepharynx short, pharynx spherical, caeca terminate blindly at level of excretory bladder. Oral and ventral suckers equal in size. Collecting channels of excretory system contain small granules.
Genetic data
The analysed lengths of nucleotide sequences were 437, 422, 1145 and 720 bp for nuclear ribosomal (complete ITS1 and partial ITS2, 28S) and mitochondrial markers (partial cox1), respectively. The sequences were identical for all specimens of S. amurensis sp. nov. At position 397 of the complete ITS1 region, intragenomic variability (T ↔ C) was detected for all samples.
Remark
The above-described mature worms of a new species, according to morphological parameters, including the number of collar spines (22), corresponded to the diagnostic features of the genus Stephanoprora (with the exception of the species Stephanoprora ornata Odhner, 1902, which has 26 spines). In addition, the parasite belonging to the genus was confirmed by the similarity of the morphology of its cercaria with the cercariae of Stephanoprora denticulata (Rudolphi, 1802), Stephanoprora uruguayense Holcman-Spector et Olague, 1989, Stephanoprora aylacostoma Ostrowski de Nunez et Quintana 2008 and S. chasanensis (Køie, Reference Køie1986; Ostrowski de Núñez, Reference Ostrowski de Núñez2007; Ostrowski de Núñez and Quintana, Reference Ostrowski de Núñez and Quintana2008; Besprozvannykh et al., Reference Besprozvannykh, Rozhkovan and Ermolenko2017). Among Stephanoprora, for which the life cycles were studied, S. denticulata, S. uruguayense and S. chasanensis circulate with the participation of Truncatelloidea Gray, 1840 molluscs. In contrast, Stephanoprora in our material uses the Cerithioidea Fleming, 1822 molluscs as the first intermediate hosts, like S. aylacostoma from Argentina. Between mature individuals of S. amurensis sp. nov. and S. aylacostoma, there were differences in the size of the head spines, the width of the cirrus-sac, the maximum size of the ventral sucker and testes (Table 2), as well as the presence of a space between the vitelline fields in the posterior part of the body for S. aylacostoma. At the cercaria stage, they differed in the size of the ventral sucker and number of granules in the excretory system, while those at the metacercaria stage had only a different pharynx width (Table 3).
To date, in the Russian southern Far East, only Stephanoprora pseudoechinata Olsson, 1876 and Stephanoprora skrjabini Dozenko, 1954 have been found in naturally infected definitive hosts (Skrjabin and Bashkirova, Reference Skrjabin, Bashkirova and Skrjabin1956; Oshmarin, Reference Oshmarin1963), while S. chasanensis was experimentally obtained in the study of the life cycle (Besprozvannykh et al., Reference Besprozvannykh, Rozhkovan and Ermolenko2017). The mature worms described in this study had significantly smaller body sizes than the trematodes of first two species (S. pseudoechinata was 3100–5920 × 319–480 μm, S. skrjabini was 7000 × 800 μm), and differed in metric data for body width, sizes of the oral and ventral suckers, pharynx, etc. from S. chasanensis (Table 2). However, at the stages of cercaria and metacercaria, the differences between the new representative of the genus and S. chasanensis were only in the number of granules of the excretory system (Table 3). Based on the above-mentioned facts, the trematodes of the genus Stephanoprora found in Russia in this study belong to a new species, S. amurensis sp. nov. In addition, the classification of S. amurensis sp. nov. and S. chasanensis to different species was confirmed by the participation of mollusks from different orders, Sorbeoconcha Ponder & Lindberg, 1997 and Hypsogastropoda Ponder & Lindberg, 1997, respectively, in their life cycles as the first intermediate hosts.
The identification of these worms as a new species was also reaffirmed by genetic data. In the reconstruction obtained using the 28S rRNA gene, S. amurensis sp. nov. entered into a cluster containing other representatives of the genus (Fig. 2). Genetic distances between species of the genus Stephanoprora did not exceed 0.6% according to this marker. The closest to the new species was the representative from the Russian southern Far East, S. chasanensis; between them, two nucleotide substitutions were revealed (T ↔ C transitions), which account for 0.2% of the differences.

Fig. 2. The phylogeny based on 28S rRNA gene sequences using the BI method. Bayesian posterior probabilities of ≥0.50 are shown. The developmental stages and localities of echinochasmids, as well as outgroup species are listed in Table 1.
On the tree based on the partial ITS2 rDNA data (Fig. 3), species of Stephanoprora from the Russian southern Far East (S. amurensis sp. nov. and S. chasanensis) also formed a separate branch inside the subcluster C, including all representatives of this genus, as well as the species Echinochasmus sp. 3 (FJ756940), which was apparently mistakenly assigned to Echinochasmus when working with parthenitae from the snail. The genetic distance between S. amurensis sp. nov. and S. chasanensis was 0.5%, while the level of differences from other species of Stephanoprora reached 1.3–2.6%.

Fig. 3. The phylogeny based on ITS2 rDNA sequences using the BI method. Bayesian posterior probabilities of ≥0.50 are shown. The developmental stages and localities of echinochasmids, as well as outgroup species are listed in Table 1. The p-distances between clusters and subclusters are shown in the in the upper right corner.
Differences in the mitochondrial sequences of the cox1 gene separated these species more significantly. Between S. amurensis sp. nov. and S. chasanensis, 2.6 and 1.3% differences were revealed at the nucleotide and amino acid levels, respectively. Data for other species were absent from GenBank.
Echinochasmus suifunensis Besprozvannykh, Reference Besprozvannykh1991
Host: Gallus gallus dom. (experimental host).
Site: small intestine.
Intensity of infection: 17 specimens.
First intermediate host: Parajuga subextensa Prozorova et Starobogatov.
Second intermediate host: Rhodeus sericeus sericeus (experimental host).
Locality: the Razdolnaya River, Primorsky Region, southern Far East, Russia (43°20′N, 131°47′E).
Echinochasmus milvi Yamaguti, Reference Yamaguti1939
Host: Butorides striatus (native host), Mus musculus, Felis catus dom., Anas platyrhynchos dom., Gallus gallus dom. (experimental hosts) (Yamaguti, Reference Yamaguti1939; Besprozvannykh, Reference Besprozvannykh1989; Besprozvannykh et al., Reference Besprozvannykh, Rozhkovan and Ermolenko2017)
Site: small intestine.
First intermediate host: Semisulcospira libertina (Koga, Reference Koga1952), Parajuga spp. (Besprozvannykh, Reference Besprozvannykh1989).
Second intermediate host: predominantly, Cyprinidae (native and experimental hosts) (Koga, Reference Koga1952; Besprozvannykh, Reference Besprozvannykh1989).
Locality: Japan, southern Far East, Russia (Yamaguti, Reference Yamaguti1939; Besprozvannykh, Reference Besprozvannykh1989).
Genetic data for E. suifunensis and E. milvi
In both E. suifunensis and E. milvi, variability between nucleotide sequences within a species was absent for the following nuclear ribosomal markers: complete ITS1 and partial 28S. The analysed length of the above-mentioned sequences was 426 and 1145 bp, respectively.
The length of partial sequences of the ITS2 rDNA region was 420 bp for E. suifunensis. Within the species, the ITS2 sequences were 100% identical. The size of ITS2 of E. milvi varied from 414 to 420 bp due to a 6 bp insertion in two samples (KT873318 and KT873319). The insertion was associated with a G/A transition at position 87. In addition, there was one T/C transition at position 366 of the sequences with no insertion. These substitutions in the ITS2 region accounted for 0.3% of the differences within E. milvi.
The sequence length of the mitochondrial cox1 gene was 432 bp for both species. The distances within E. suifunensis and E. milvi were 1.2 and 0.7%, respectively. Amino acid substitutions were not obtained within species.
Remark
Snails of Parajuga emitting E. suifunensis cercariae were collected from a type locality of species (Besprozvannykh, Reference Besprozvannykh1991). These cercariae, as well as the metacercariae and mature worms obtained from them, were morphometrically identical to worms described in Besprozvannykh (Reference Besprozvannykh1991). Given this and the fact that the author of the species and life cycle studies of E. suifunensis is the author of this study, we used the data presented in Besprozvannykh (Reference Besprozvannykh1991) in the discussion and also provided further detail of the metric data and photographs of the different developmental stages of worms (Fig. 4A–D) that were absent in the earlier publication. Another representative of the genus, E. milvi (Fig. 4E–I), was also described previously (Besprozvannykh, Reference Besprozvannykh1989); therefore, all of the above applies to this species.
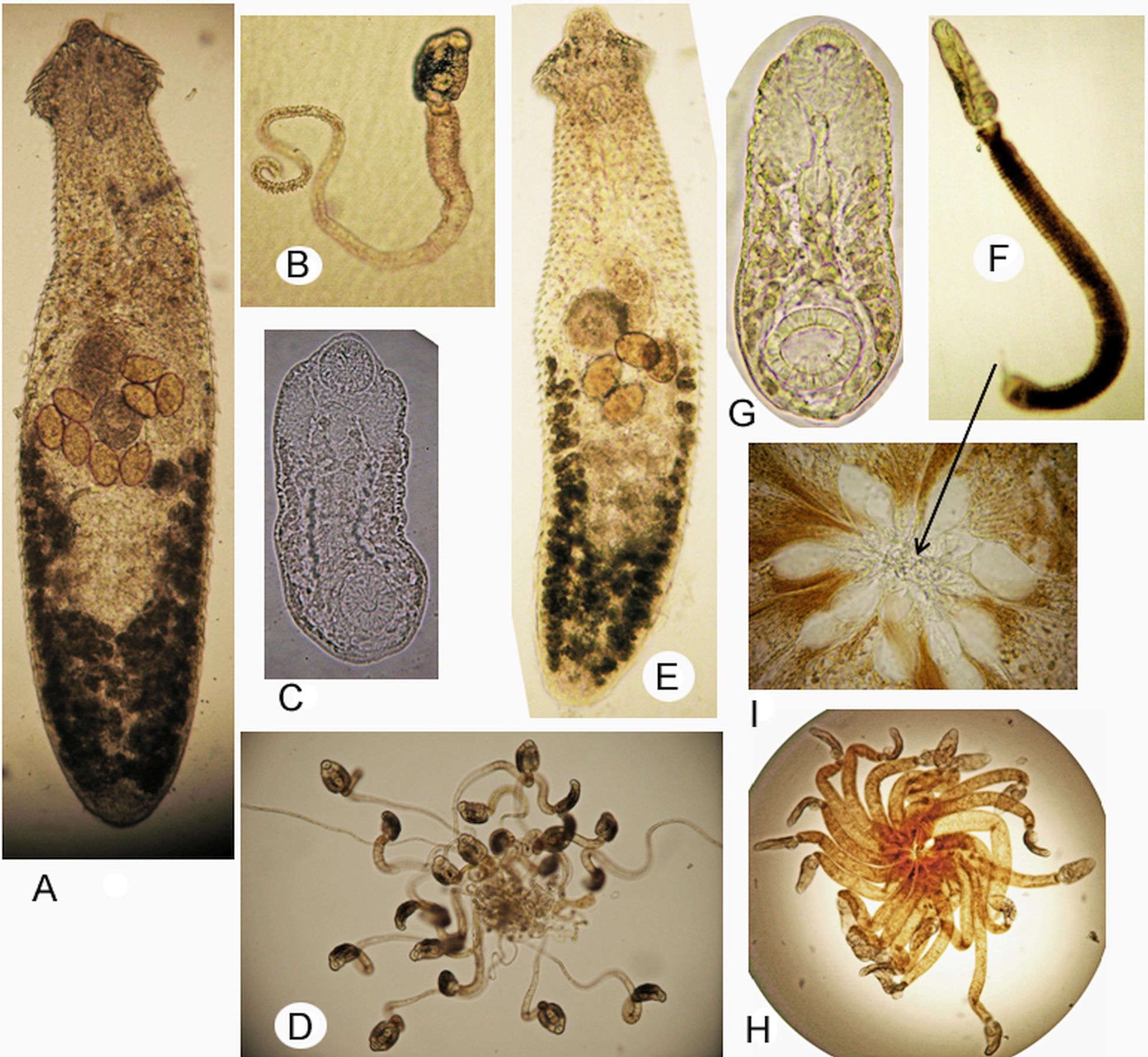
Fig. 4. Echinochasmus suifunensis: (A) adult worm; (B) cercaria; (C) body of cercaria; (D) ‘Rattenkonig’; Echinochasmus milvi: (E) adult worm; (F) cercaria; (D) body of cercaria; (H) ‘Rattenkonig’; and (I) interlacing of the bulbous outgrowths of tails.
The molecular studies showed 100% identity in the nucleotide sequences of the ITS1 rDNA region and 28S rRNA gene obtained from mature worms of E. suifunensis and E. milvi. In addition, there was an absence of species separation based on the data of the ITS2 rDNA region. The nucleotide distances, based on partial sequences of the ITS2 rDNA region (including data from GenBank), were low within E. suifunensis and E. milvi (from 0 to 0.5%); the two detected nucleotide substitutions were not fixed and did not distinguish these species. Moreover, both species have a similar life cycle in which, on the territory of the Russian southern Far East, the first and second intermediate hosts are snails of Parajuga and freshwater fish (with metacercariae localized on the gills).
However, the affiliation of these trematodes to different species is not in doubt and was confirmed by the morphometric data of adult worms, cercariae and metacercariae. Mature worms of E. suifunensis and E. milvi differed in body length and the length of angular spines (Table 2); the parasites differed in body size at the cercaria stage and there were differences in all metric indicators at the metacercaria stage (Table 3). In addition, unlike E. milvi, cercariae of E. suifunensis had spines on both sides of the oral sucker. Moreover, despite the fact that the cercariae of both species united to form the ‘Rattenkonig’ (for E. suifunensis, this fact was established in the current study), they differed in the morphology of the tail and type of cercariae aggregation. Echinochasmus suifunensis had a transparent tail, twisted in a spiral at the end, while the tail of E. milvi was dull white or pigmented (dark or light brown) and there was a thin outgrowth with a bulbous tip at its distal end. In the first case, ‘Rattenkonig’ was formed due to the interlacing of the twisted parts of the tails of the cercariae and in the second, due to the interlacing of the bulbous outgrowths (Fig. 2D, H, I). These data indicated the validity of E. suifunensis and E. milvi at the morphological level.
At the molecular level, the validity of species was confirmed only by the sequences of the mitochondrial cox1 gene. The distance between E. suifunensis and E. milvi was 6.7% (including one fixed nonsynonymous and 24 fixed synonymous substitutions), while the distances within these species were 1.2 and 0.7%, respectively; that is, the difference was almost 6–10 times higher than within each separate species. Moreover, one amino acid substitution (I ↔ M) was also identified between E. suifunensis and E. milvi. The presence of a large number of fixed substitutions indicated the presence of speciation processes, which have so far only been detected by analysis of the mitochondrial gene, which is more sensitive, thus revealing cryptic species with recent ancestry. Previously, using mitochondrial DNA, the presence of cryptic species was detected for different groups of worms (Blouin, Reference Blouin2002; Vilas et al., Reference Vilas, Criscione and Blouin2005; Lavikainen et al., Reference Lavikainen, Haukisalmi, Lehtinen, Laaksonen, Holmström, Isomursu, Oksanen and Meri2010). Thus, we considered that the validity of E. suifunensis and E. milvi were confirmed by genetic data.
Discussion
Phylogenetic analysis of Echinostomatoidea based on molecular studies of species from different families was carried out relatively recently by Tkach et al. (Reference Tkach, Kudlai and Kostadinova2016). Unfortunately, notwithstanding the scope of this review, data on individual families, including Echinochasmidae, were still insignificant and more information was required for this taxonomic group. In the present study, in addition to the differences that we identified for the analysed echinochasmids, it is necessary to note some aspects in the distribution of species of this family on phylogenetic trees built using nuclear markers, 28S and ITS2. As in previously published studies (Tkach et al., Reference Tkach, Kudlai and Kostadinova2016; Besprozvannykh et al., Reference Besprozvannykh, Rozhkovan and Ermolenko2017), representatives of the genus Echinochasmus were divided into two separate clusters (Figs 2 and 3), the first of which included species whose cercariae had a short tail, including a type species, Echinochasmus coaxatus Dietz, 1909. In addition, all species of Echinochasmus of this cluster had 24 collar spines. Echinochasmus bursicola (Creplin, 1837) also clustered with these species and as Tkach et al. (Reference Tkach, Kudlai and Kostadinova2016) previously noted, was apparently mistakenly assigned to a different genus, Uroproctepisthmium. In the second cluster, species of Echinochasmus joined with representatives of another genus Stephanoprora (Figs 2 and 3). Most species in this cluster had 20–22 collar spines and their cercariae had a long tail. Based on the 28S rRNA gene sequences, the distances between these two clusters and the only representative of the genus Microparyphium were in a range from 4.5 to 5.4%, while the distances within the first and second clusters were 1.4 and 2.4%, respectively. Both clusters were also clearly separated using data from the ITS2 rDNA region (Fig. 3).
Based on the data obtained in the first cluster, the genus Echinochasmus combines E. coaxatus and E. japonicus using both 28S and ITS2 markers, along with E. beleocephalus and E. bursicola according to the 28S rRNA gene sequences (Figs 2 and 3). As for the species Stephanoprora and Echinochasmus being united in the second cluster by the 28S rRNA gene, the absence of any statistically confirmed differences between them makes it necessary to designate, at this stage of the research, the whole group as Stephanoprora-like (Fig. 2). In contrast, based on the ITS2 region, the distance between the first and second clusters of 17.3% was comparable with the distance between all representatives of the family Echinochasmidae and Echinostoma trivolvis (Echinostomatidae), 19.6%. At the same time, within cluster 2, there was also a subdivision into three more equivalent subclusters, A, B and C (Fig. 3), the distances between which are in the range from 6 to 9.5%. Such high values of differences may indicate that the species belonging to each of the subclusters belong to separate genera. Thus, there is a morphological similarity in the number of adoral spines and tail length in cercaria of species in the second cluster obtained using both nuclear markers; however, there were significant distances between subclusters on the ITS2 tree. The data obtained indicated the possibility of distinguishing at least two subfamilies that separately combined the species of the first and second clusters, as well as new genera based on the structure of the second cluster. However, we considered it premature to make a final conclusion about whether the worms belong to one or different genera, as well as to solve taxonomic problems within Echinostomatoidea at a higher level. The main reason for this is the limited amount of genetic data for both Echinochasmidae and other representatives of the subfamily.
As mentioned above, most representatives of the second cluster of the phylogenetic reconstruction based on the 28S and ITS2 markers had cercariae with long tails. However, questions remain regarding E. mordax, for which the morphology of cercariae was unknown, as well as E. donaldsoni and representatives of Echinochasmus with unknown species affiliation. E. donaldsoni had 20 adoral spines, but cercariae with short tails (Beaver, Reference Beaver1941). For worms designated as E. donaldsoni, genetic data were available for only one specimen obtained from naturally infected animals (Tkach et al., Reference Tkach, Kudlai and Kostadinova2016). Morphometric and biological descriptions were not given for confirmation of the belonging to this species. It was important to remember that the presence of morphological twins within the genera is quite widespread among digeneans. In this case, in the absence of data on the morphology of individuals of the species, assigning it the first obtained genetic data may be incorrect. This also applies to Echinochasmus with an unknown species affiliation: the nucleotide sequences for Echinochasmus sp. 1. were obtained from parthenitae (Stanevičiūtė et al., Reference Stanevičiūtė, Stunžėnas and Petkevičiūtė2015) and there was no morphological description for Echinochasmus sp. 3.
Designated difficulties may complicate the interpretation of the results, including this study. Based on the molecular data of the ITS2 rDNA region, Echinochasmus sp. 2, obtained experimentally by Molnár et al. (Reference Molnár, Gibson, Majoros, Székely, Sándor and Cech2016), entered the second cluster together with Stephanoprora. Mature worms of this species, like other echinochasmids in the cluster, had 20 adoral spines. Unfortunately, the authors (Molnár et al., Reference Molnár, Gibson, Majoros, Székely, Sándor and Cech2016) did not provide a detailed description of morphological characteristics for cercaria but indicated that ‘the tail was almost as long as the body’. However, based on the figure in the publication, this larval stage had a tail that is characteristic of long-tailed Echinochasmidae cercariae. The tail had numerous folds formed during its contraction, as well as numerous vacuole-like inclusions, which are not typical for short-tailed Echinochasmus cercariae. At the same time, the cercaria shown in micrograph differs in a number of morphological features from cercaria in the figure: ventral sucker is smaller than the oral one vs ventral sucker is equal to or larger than the oral one; and a tail typical for short-tailed cercariae vs tail like long-tailed cercariae. Thus, the use of data obtained by Molnár et al. (Reference Molnár, Gibson, Majoros, Székely, Sándor and Cech2016) violates the objectivity of taxonomy assessment and analysis of phylogenetic relationships of Echinochasmidae to a certain extent. The greatest problems of taxonomy, systematics and phylogeny arise when, during the study of the life cycle of both echinostomatids and other representatives of digeneans, some developmental stages were obtained experimentally, while others were collected by dissecting naturally infected animals. These results are combined and a priori assigned to the same species. Considering that closely related worms belonging to different species can have similar morphometries at different stages, cases of using incorrect methodology for studying the life cycle can lead to erroneous conclusions on the taxonomy of the studied trematodes. If such studies are accompanied by obtaining genetic data, the resolution of questions of taxonomy, systematics and phylogeny becomes even more difficult.
This situation is compounded by the fact that there are no clear criteria for differentiating trematode species in different systematic groups due to a limited amount of molecular data. Our studies of echinochasmids showed that the 28S rRNA gene, as well as the ITS1 and ITS2 regions, did not resolve the problem of species differentiation for E. milvi and E. suifunensis. In the presence of a high level of nuclear DNA conservatism, and subject to obtaining adequate data on the life cycle, the taxonomic status can be confirmed by nucleotide sequences of the mitochondrial genome, since this is more variable. However, there are no significant criteria of these differences for establishing the taxonomic affiliation of most trematodes, since, in the structure of macro- and micro-populations of worms from various taxonomic groups, there is no data on the rate of mutation accumulation in the mitochondrial genome. Based on this, we emphasize that data for the adequate taxonomic and phylogenetic classification of digenean species should include a description of both the morphology of developmental stages and the genetic data for the studied worms. Additionally, it should particularly be done at the first acquisition of genetic data for the designated species.
Financial support
This research was funded by the Government basic research program (project no. 0267-2019-0018).
Conflict of interest
The authors declare no conflict of interest.
Ethical standards
The authors assert that all procedures contributing to this work comply with the ethical standards of the relevant national and institutional guides on the care and use of laboratory animals including fish and birds.