Introduction
The United States Antarctic Research Program undertook an oversnow traverse programme in the late 1950s. One such traverse, the Victoria Land Traverse 1959–60 (VLT) (Fig. 1), was a geological and geophysical exploration of Victoria Land involving seismic, gravimetric, magnetic, geological, glaciological, isotopic and atmospheric surveys. A number of discoveries were made including the Wilkes Subglacial Basin (WSB), ice streams in the continental ice sheet, chaotic ice surface terrain and underlying complex subglacial topography, the Outback Nunataks, the upper reaches of the Rennick Glacier and the USARP Mountain Range. Furthermore, the VLT determined the continental ice sheet thickness in East Antarctica and variations in gravity over the traverse route. The VLT also discovered the Wilkes Land Anomaly (WLA), a substantial gravity anomaly centred at 70°00'S-140°00'E. The feature exhibited a negative free air gravity anomaly of 158.3 mgals (Weihaupt Reference Weihaupt1961, Reference Weihaupt1976, Schmidt Reference Schmidt1962) that has been a subject of interest and some controversy (Bentley Reference Bentley1979) since first reported. The controversy, a dispute about the use of gravity data over the 400 km transect of the WLA to define subglacial topography, was resolved by examination of the gravity profile of the remaining 2000 km of the same survey. This examination confirmed that, in addition to the VLT’s accurate depiction of the subglacial topography of the WSB on the basis of gravity data, the profile of the WLA segment of the traverse was also accurate. The subglacial topography of both the WSB and the WLA reported by the VLT has been further confirmed by more recent investigations in the region related to the continental ice sheet, crust, lithosphere and mantle. These include airborne radiosound survey (Steed & Drewry Reference Steed and Drewry1982, Lythe & Vaughan Reference Lythe and Vaughan2001), airborne magnetic survey (Ferraccioli et al. Reference Ferraccioli, Coren, Bozzo, Zanolla, Gandolfi, Tabacco and Frezotti2001), airborne gravity survey (Jordan et al. Reference Jordan, Ferraccioli, Armadillo and Bozzo2014) and satellite remote sensing (Reigber et al. Reference Reigber, Balmino, Schwintzer, Biancale, Bode, Lemoine, König, Loyer, Neumayer, Marty, Barthelmes, Perosanz and Zhu2002, Weihaupt et al. Reference Weihaupt, Rice and van der Hoeven2010), as well as WISE/ISODYN British-Italian multidisciplinary surveys involving aeromagnetic, aerogravity, seismic, electrical conductivity and petrologic studies (Bozzo & Ferraccioli 2007). All report the subglacial topography of the WSB and the WLA to be in very good agreement with that of the original VLT ground-based survey. Most recently, BEDMAP2 datasets and maps (Fretwell et al. Reference Fretwell, Pritchard and Vaughan2013) and examination of the origin and subglacial topography of the WSB (Weihaupt et al. Reference Weihaupt, van der Hoeven, Lorius and Chambers2014a) have again confirmed the validity of using gravity data to profile the subglacial topography in East Antarctica.

Fig. 1 Route of the United States Victoria Land Traverse (VLT) of 1959–60 (after Weihaupt Reference Weihaupt1961; base map compliments SCAR). Station 531 marks the westernmost point of the VLT where it joins the French Adélie Land Traverse of 1958–59 (Rouillon Reference Rouillon1960).
Airborne sensing elsewhere in Antarctica suggests that structures similar to the WLA may exist in eastern Victoria Land, the Ross Sea sector, West Antarctica (LeMasurier et al. Reference LeMasurier and Thomson1990, Behrendt et al. Reference Behrendt, Flinn, Blankenship and Bell1998) and in the Weddell Sea sector (Weihaupt et al. Reference Weihaupt, Rice and van der Hoeven2010). Considering the half century hiatus in scientific observations since the discovery of the WLA, these new data are welcome and provide an important opportunity to update our knowledge of the feature to further detail its geological and geophysical characteristics, and to evaluate the possible origin of the site.
A review of the geological setting of the region, the principal observational results and alternative explanations for the WLA is provided here. This review gave rise to the following questions: i) What is the most likely explanation for the association of the gravity anomalies with the complex subglacial topography? ii) What is the significance of the associated magnetic anomalies? iii) Are the properties of the WLA comparable to the properties of other similar structures? iv) What, therefore, is the most likely origin of the WLA and its associated features?
Geological and geophysical setting
The area of investigation is located in Wilkes Land in East Antarctica, approximately 350 km inland from George V Coast (Fig. 2). A sector of the Precambrian East Antarctic craton, the subglacial geology of the Victoria Land and Wilkes Land regions ranges from ancient igneous and metamorphic complexes to Mesozoic sedimentary and volcanic sequences in the vicinity of the Transantarctic Mountains (TAM). This region underwent tectonic extension in late Cretaceous, large scale sedimentation in late Eocene-Neogene, and initiation of glaciation for the TAM, Victoria Land and Wilkes Land sectors in late Eocene-early Oligocene. The development of the Antarctic continental ice sheet commenced c. 20 Ma, reaching its maximum extent in the last 2.58 Ma.

Fig. 2 Antarctica, showing the location of a. the original Wilkes Land Anomaly and b. the updated and enlarged area of the Wilkes Land Anomaly.
Located in the near-coastal vicinity of the WSB, the surface of the continental ice sheet overlying the basin lies at elevations ranging from 2300–2600 m and is characterized by the broad level plateau of East Antarctica. Sloping toward the continental coastline to the north, the ice sheet is underlain by subglacial rock topography of relatively moderate relief that varies from 1700–2300 m above sea level, and displays regional free air gravity anomalies that range from -42.6 to +23.7 mgal, averaging a rate of change of 0.1 mgal km-1 over a distance of c. 700 km (Weihaupt Reference Weihaupt1961). In contrast, the area of the WLA is characterized by steeper ice surface slopes to the coast of the Southern Ocean, and consequently more rapid movement of the continental ice sheet. Ice streams in the continental ice sheet have been reported in the area of the WLA as the Mertz and Ninnis glaciers (Weihaupt Reference Weihaupt1961, Weihaupt et al. Reference Weihaupt, Chambers, van der Hoeven and Lorius2014b).
Within this setting, centred at 70°00'S-140°00'E, the area of investigation exhibits subglacial topography, gravity and magnetic anomalies that distinguish it from adjacent areas in East Antarctica. The area is also observed to have a dynamically disturbed continental ice sheet surface with basins, troughs, unusually large crevasses, local topographical ice surface relief of ≥62 m and an ice sheet thickness of ≥3042 m, c. 1000 m thicker than the adjacent regional ice sheet (Rouillon Reference Rouillon1960, Weihaupt et al. Reference Weihaupt, Stuart, van der Hoeven, Lorius and Smith2012). The free air gravity anomaly reported in 1961, reflecting lithospheric structure, density and subglacial topography, varies from -106.3 mgal near the centre of the feature to +41.0 mgal in its south-eastern extremity. Gravity data observed by the Adélie Land Traverse nearer the coast (Rouillon Reference Rouillon1960), when adjusted to the VLT gravity data farther inland, gave a total free air gravity anomaly in the area of initial investigation of -158.3 mgal (Weihaupt Reference Weihaupt1976). Representing a local rate of change of gravity with distance more than 18 times that of the mean rate of change regionally, the free air gravity anomaly represents a major mass deficit in the lithosphere. The importance of this feature lies not only in the question of its origin in itself, but this part of the WSB is one of the most prominent regional negative geoid and associated gravity anomalies of the Antarctic continent.
The minimum and maximum elevations of the subglacial profile, originally set at -500 m and +350 m, respectively, were believed at the time to be accompanied by greater relief in the area because of the unsurveyed local and regional slopes adjacent to the profile. Subsequent airborne radiosound data reveal these original estimates to have been conservative (Steed & Drewry Reference Steed and Drewry1982, Lythe & Vaughan Reference Lythe and Vaughan2001); data now confirmed by BEDMAP2 (Fretwell et al. Reference Fretwell, Pritchard and Vaughan2013). The minimum and maximum subglacial topographical elevations are now shown to be in the range of -1000 m and +500 m, respectively, defining a total subglacial topographical relief of ≥1500 m in contrast to the original estimate of 848 m. Therefore, the maximum ice thickness in the area of investigation is ≥3500 m in contrast to the original estimate of 3042 m, and the diameter of the feature is significantly greater than first proposed. Climatically and glaciologically, the region is comparable to that elsewhere in East Antarctica in the vicinity of the Southern Ocean (Mayewski Reference Mayewski1976).
On the basis of combined French, United States, British, German and Italian data, the anomalous character of the area of investigation is confirmed. While a variety of explanations have been suggested for this assemblage of anomalies, five explanations merit closer examination: i) a volcanic construct, ii) an igneous intrusion, iii) an ancient igneous diapir, iv) a sedimentary basin, v) a glacially eroded subglacial valley, vi) a tectonic feature, or vii) an impact crater. Whatever its origin and original topographical expression, the feature has been modified since its formation by subaerial erosion or glacial erosion by the overriding continental ice sheet, or both.
Principal observational results
The discovery of the WLA and its associated subglacial topography was accomplished using gravity and seismic ground-based observations (Weihaupt Reference Weihaupt1961, Weihaupt et al. Reference Weihaupt, Stuart, van der Hoeven, Lorius and Smith2012).
The results enable the identification of the negative free air gravity anomaly of 158.3 mgal, i.e. the original anomaly observed for the WLA. The computed gravity values, reported elsewhere (Weihaupt Reference Weihaupt1961, Weihaupt et al. Reference Weihaupt, Rice and van der Hoeven2010, Reference Weihaupt, Stuart, van der Hoeven, Lorius and Smith2012), were then tied to the seismic depth determinations and the subglacial topography profiled (Weihaupt et al. Reference Weihaupt, van der Hoeven, Lorius and Chambers2014a); profiles that are in good agreement with the recent airborne gravity data reported by Jordan et al. (Reference Jordan, Ferraccioli, Armadillo and Bozzo2014).
The seismic values, which represent continental ice sheet thicknesses, provide subglacial rock surface control for the gravity values, enabling construction of rock surface profiles of the feature, profiles that are in very good agreement with the recent airborne and satellite surveys.
The combined seismic-gravity data reveal subglacial circular basin topography. And while the 1958–60 surveys were ground-based, subsequent surveys have involved largely airborne remote sensing and satellite remote sensing. While the focus of most of these investigations has been the nature of the subglacial lithosphere and of the overlying continental ice sheet, all have confirmed the subglacial topography in the vicinity of the WLA. Additionally, the region inland of the WLA is confirmed to display subglacial topography and continental ice sheet surface of little relief as reported by the initial ground survey. That survey also observed the ice surface in the area of investigation to undulate, displaying broad basins as large as 6.5 km in diameter and long troughs in the ice up to 5 km long and 50–100 m deep. Accompanied by ice streams in the continental ice sheet, these features overlie the complex subglacial topography, confirming the boundaries of the WLA, originally postulated to have a diameter of 243 km (Fig. 2a), as a diameter of 510 km (Fig. 2b).
Analysis and interpretation
Among the explanations for the origin of the WLA, the complex subglacial topography and the chaotic overlying continental ice sheet is the suggestion that it is due to a subglacial volcanic construct similar to others reported in Antarctica (LeMasurier et al. Reference LeMasurier and Thomson1990, Behrendt et al. Reference Behrendt, Flinn, Blankenship and Bell1998), or an igneous intrusion, structures that are abundant on the continent. Other potential explanations are those of an ancient igneous diapir, a subglacial sedimentary basin, a glacially eroded subglacial valley (Ferraccioli et al. Reference Ferraccioli, Coren, Bozzo, Zanolla, Gandolfi, Tabacco and Frezotti2001), a tectonic feature in the WSB lithosphere perhaps including a back-arc formation (Jordan et al. Reference Jordan, Ferraccioli, Armadillo and Bozzo2014), or that the feature is an impact crater following reports of similar anomalies elsewhere in Antarctica (Behrendt et al. Reference Behrendt, Flinn, Blankenship and Bell1998, Weihaupt et al. Reference Weihaupt, Rice and van der Hoeven2010, Weihaupt et al. Reference Weihaupt, Chambers, van der Hoeven and Lorius2014b).
Gravity anomalies are characteristic of volcanic constructs, and normally reflect solidified magma chambers or volcano topography. Characteristically such magma chambers give rise to positive free air gravity anomalies not unlike that observed for the WLA, but of smaller area, lower absolute magnitude and without comparable accompanying negative gravity anomalies. Some contribution to such anomalies is also made by the morphology of a volcano, differing depending upon whether it is a shield, cinder cone, composite, dome or caldera type volcano. However, such free air gravity anomalies and topographical profiles differ from Wilkes Land in that the WLA exhibits both positive and negative gravity anomaly values, which are also of much greater absolute magnitude than those of volcanoes, and a more complex topography. On the other hand, modified volcanic topography may be created by later stages of eruption, caldera collapse or subsequent erosion. Later eruption may create central peaks within the caldera such as cinder cones which often appear as islands in a crater lake. Such cones normally consist of a single peak in contrast to the double peak noted in the centre of the profile of the Wilkes Land structure, a feature that is also considerably larger than volcanic cinder cones. With few exceptions, volcanic constructs on Earth are much smaller than the subglacial structure in Wilkes Land, and are typically of the order of a few kilometres in diameter or less. Further, there is no significant evidence for major Cenozoic volcanics or intrusions in the region of the WSB (Goodge & Fanning Reference Goodge and Fanning2010). Therefore, the volcanic explanation for the WLA is regarded as less feasible than other explanations.
Igneous intrusions, our second possible explanation for the WLA, also give rise to positive gravity anomalies, whether magma chambers, batholiths, stocks, dikes, sills, laccoliths or similar constructs. All may exhibit positive free air gravity anomalies of a magnitude approaching that of the WLA, but all exhibit profiles that are substantially different, and are not normally accompanied by negative free air gravity anomalies. Originally intrusive, and unless exposed by erosion, such structures do not have topographical expressions like those of volcanoes, or of the complex subglacial topography observed in Wilkes Land. Therefore, the igneous structure explanation for the WLA is also regarded to be less feasible than the presence of an igneous diapir, subglacial basin, glacially eroded subglacial valley, tectonic feature or impact crater.
Igneous diapiric structures produce circular gravity anomalies, usually functions of relatively low density buoyant silicic melts that penetrate denser overlying crust, giving rise to granitic plutons. The upward movement of the magma results in a pipe shape with lateral spreading near the surface, producing a teardrop configuration of the emplaced magma, sometimes causing central uplift and surface deformation. Depending upon the size of the emplacement and the density contrast between the granitic material and the surrounding crustal rock, negative gravity anomalies of varying magnitude may be created. Most known diapirs are of the order of tens of kilometres in diameter, such as that of the La Bazana diapir in Spain (Galadi-Enriquez et al. Reference Galadi-Enriquez, Galindo-Zaldivar, Simancas and Exposito2003), to hundreds of kilometres, such as the Sombrero Uplift in the Altiplano-Puna in the Andes and the Rio Grande Uplift in New Mexico (Fialko & Pearse Reference Fialko and Pearse2012). While the signature of diapiric gravity anomalies is traditionally circular, diameters of such features are normally much smaller than that of the WLA. Similarly, the magnitudes of the anomalies are of the order of 20–50 mgals, small compared to the gravity anomaly of the WLA. On the other hand, igneous diapirs emplaced relatively early in Earth history may have been larger than those emplaced later, such as the La Bazana and Sombrero diapirs. Therefore, it might be argued that the WLA represents a feature created in Earth’s very early history when mantle temperatures and circulations were more robust. However, given the characteristics of diapirs noted above, the diapiric explanation is considered to be less probable at this time than those of the presence of a subglacial sedimentary basin or glacially eroded subglacial valley.
Free air gravity anomalies reflect subsurface mass variations as well as topography, thus the negative free air gravity portion of the WLA may be due to the presence of a subglacial sedimentary basin or a glacially eroded subglacial valley, such as those created by ice streams in the continental ice sheet. However, the subglacial basin and subglacial valley suggestions appear inconsistent with the depth of erosion required to produce subglacial topography of this magnitude, i.e. valleys 1000 m below sea level. Although a substantially greater continental ice sheet thickness of the past and greater ice stream vigour might be invoked to achieve such basin or valley erosion, a continental ice sheet thickness of 7000 m is implied to achieve this depth of erosion, including isostatic considerations, considerably greater than the Pleistocene Antarctic record reveals. If, on the other hand, the continent were at a higher isostatic elevation due to a much thinner continental ice sheet sometime during the Pleistocene, allowing for the subglacial surface to achieve a higher stand closer to sea level, it might be argued that a vigorous ice stream could accomplish the required subglacial erosion and that the continent was later isostatically depressed by an increased continental ice sheet mass, resulting in a subglacial basin or valley at the present depth of the WLA. However, the time frame of the Pleistocene appears insufficient to have allowed the proposed isostatic depression and rebound in this vicinity, as does the range of isostatic depression and elevation revealed in the Antarctic record. Therefore, the record of the recent glaciological past is regarded to be inadequate to explain the subglacial topography or the negative free air gravity anomaly on the basis of continental ice stream erosion, or of a substantial glacial detrital sedimentary basin or subglacially eroded valley. Furthermore, both are inadequate to account for the entire negative gravity anomaly of the WLA.
Beyond that, because the bottoms of valley floors are not yet apparent in modern two-dimensionally focused radar surveys, these basin and valley depths, including the nearby Astrolabe Subglacial Basin, Adventure Subglacial Trench, Webb Subglacial Trench (Western Basins) and Zélée Subglacial Trench in the vicinity of the WLA, may in fact all be deeper than previously reported, making the subglacial erosion argument even less plausible. Depths as much as 1500–2100 m below sea level may exist in the northern portion of the WSB. It should be noted that ice streams in the continental ice sheet drain each of these subglacial features, perhaps not unrelated to the Mertz and Ninnis glaciers that overly the WLA. Furthermore, if these lowland trenches represent inherited ancient structures, glacial incision may be much older than the Pleistocene. Glacial erosion may have occurred between the Oligocene and mid-Miocene when the early East Antarctic Ice Sheet was probably much more dynamic. While large and deep subglacial valleys exist in the interior (Ferraccioli et al. Reference Ferraccioli, Coren, Bozzo, Zanolla, Gandolfi, Tabacco and Frezotti2001, Fretwell et al. Reference Fretwell, Pritchard and Vaughan2013, Weihaupt et al. Reference Weihaupt, van der Hoeven, Lorius and Chambers2014a), significant retreat of the actively eroding margin of the ice sheet near the WLA probably occurred in the Pliocene. The East Antarctic Ice Sheet may have been very dynamic in the Pliocene, allowing quite sufficient time to erode very deep valleys with the potential for steering ice streams in the overlying continental ice sheet. Inherited tectonic structures and differential erosion have the potential to influence the presence and configuration of both subglacial valleys and overlying ice streams. However, the subglacial valley topography does not in itself provide an explanation for the substantial positive free air gravity anomaly at the centre of the WLA feature, although the presence of a highland, e.g. a mesa resulting from ancient structure, may satisfy this requirement. Similarly, the linear shapes of most of these subglacial valleys are unlike the circular shape of the WLA. Therefore, the presence of a subglacial sedimentary basin or valley alone is not thought to provide an adequate explanation at this time for the origin of the WLA.
However, tectonic features commonly give rise to gravity anomalies (Jordan et al. Reference Jordan, Ferraccioli, Armadillo and Bozzo2014), as well as to magnetic anomalies (Ferraccioli et al. Reference Ferraccioli, Coren, Bozzo, Zanolla, Gandolfi, Tabacco and Frezotti2001). This calls into question the tectonic history not only of the WLA, but of the WSB. The WSB owes its origin to large scale tectonic processes and post-tectonic modification by glacial and marine erosion and deposition (Weihaupt et al. Reference Weihaupt, van der Hoeven, Lorius and Chambers2014a). Tectonic processes which have been suggested for the origin of the WSB include lithospheric flexure that involved thick continental crust as a flexural ‘low’ adjacent to the TAM, the flexural ‘high’ driven by extension within the Ross Sea Rift. Central uplift of the TAM is considered by these investigators to reflect the differential flexural rigidity of thicker East Antarctica crust and the thinner Ross Sea embayment due, in part, to contrasting thermal ages and geotherms in these two provinces. On the other hand, the TAM boundary between East and West Antarctica has also been described as divergent. This model, augmented by the isostatic effects of erosion and mass loss combined with thermal uplift of the TAM, is offered in support of hypothetical rifting reminiscent of foreland basins. However, gravity modelling by Jordan et al. (Reference Jordan, Ferraccioli, Armadillo and Bozzo2014) has revealed East Antarctic crustal thickness to be less than that predicted by the flexural model. Sedimentary infill of <1 km in basins of the WSB leads to the belief that the WSB contains a former broad back-arc basin and fold-and-thrust belts, as well as craterform topography (Ferraccioli et al. Reference Ferraccioli, Coren, Bozzo, Zanolla, Gandolfi, Tabacco and Frezotti2001, Weihaupt et al. Reference Weihaupt, Rice and van der Hoeven2010). The data from Jordan et al. (Reference Jordan, Ferraccioli, Armadillo and Bozzo2014), which provide aeromagnetic and aerogravity data in the northern portion of the WSB, raise questions about the separation of the East Antarctic craton from south-eastern Australia and about the significance this may have for the origin of the WLA.
This suggests that the circular footprint of the WLA may potentially be a remnant of the Gawler Craton of southern Australia, a post-separation component now of the Terre Adélie Craton in Antarctica. Substantial gravity and magnetic anomalies and the circular boundaries often associated with structures within cratons, or associated with the boundaries of cratons, may provide insight into the origin of the WLA. The Terre Adélie Craton may reflect the edge of the Gawler Craton in southern Australia (Reid & Martin Reference Reid and Martin2012), e.g. both as components of the larger Mawson Craton. This explanation has the advantage of providing circular geometry, similar to the Gruehonga Craton that, due to the diverse bedrock type and structure, provides differential erosion and overdeepened topography which controls the Jutulstraumen ice stream in Dronning Maud Land. Similarly, mesa-like topography may be created in ancient lithologies where differential subaerial erosion occurs due to resistant rocks such as the early to mid-Jurassic Ferrar dolerites compared to the much older host Beacon sandstones. Because topography may be a function of isostatic response to differential erosion, including the dynamic ice flow of the Oligocene-Miocene interval, mesa-like highlands may be created. The tectonic history of the WSB, particularly its relation to the separation of Antarctica from Australia, is believed to provide a potentially valuable explanation for the origin of the WLA, although it does not yet provide an adequate explanation for the craterform subglacial morphology or the circular negative free air gravity anomaly incorporated into a positive free air gravity anomaly. Furthermore, the patterns of tectonically-generated anomalies tend to be linear, reflecting the linearity of the tectonic structures, such as faults, that generate them, as opposed to the circular configuration of the WLA.
Although not specifically tectonic, gravity signals from the Antarctic mantle, a function of density or thermal variations, have the potential to create gravity signatures at Earth’s surface. Mantle structural features are normally revealed as broad large scale variations which are resolved into long wavelength gravity signals of the order of 2000–3000 km, much larger than that of the Wilkes Land feature. Density and thermal variations at the top of the mantle are more functions of broad lithospheric control, and are only very modest in the upper mantle, demonstrating that the mantle is unlikely to be the direct source of the WLA.
Gravity anomalies characteristic of meteorite impact structures are normally circular, and reflect both complex topography and density variations in the impact structure. Furthermore, large meteorite impacts have the potential to penetrate the Earth’s crust, resulting in a buoyant plutonic structure, a diapir. On the other hand, the association of both negative and positive free air gravity anomalies is more characteristic of large impact structures on virtually all terrestrial objects in the solar system (Sharpton et al. Reference Sharpton, Burke, Camargozanoguera, Hall, Lee, Marin, Suarez-Reynoso, Quezandamuneton, Spudis and Urrutiafucugauchi1993). Satellite remote sensing reveals a mass concentration at the site of the WLA on the basis of gravity data (Fig. 3b), whereas a major circular positive free air gravity anomaly appears elsewhere in Wilkes Land (Fig. 3c), reported to be a mascon uplifted as a dense mantle plug recoiled from meteorite impact, surrounded by a negative free air gravity anomaly. Such rebound is commonly expressed as an elevated peak or peak ring, a mascon, in the centre of large impact structures (Grieve Reference Grieve2006, Melosh et al. Reference Melosh, Freed, Johnson, Blair, Andrews-Hanna, Neumann, Phillips, Smith, Solomon, Wieczorek and Zuber2013). Impact fracturing, melt and brecciation commonly accompany such impacts, accounting for the negative gravity anomaly that surrounds the central positive anomaly. Features comparable to these are also associated with recently acquired magnetic data in the WLA. Therefore, we will examine the gravity deficit associated with the WLA, and then the accompanying magnetic signatures.
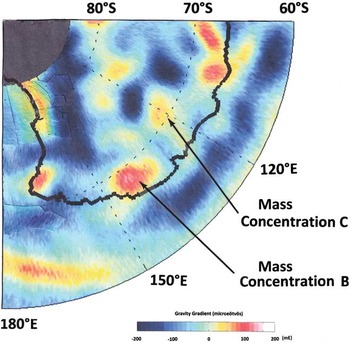
Fig. 3 Regions of mass concentration in Wilkes Land identified from GOCE (Gravity field and steady-state Ocean Circulation Explorer) showing b. the mass concentration of the Wilkes Land Anomaly (Weihaupt et al. Reference Weihaupt, Stuart, van der Hoeven, Lorius and Smith2012) and c. a nearby mass concentration based upon gravity gradient satellite data (Bouman et al. Reference Bouman, Floberghagen and Rummel2013; Courtesy of Johannes Bouman, Deutsches Geodätisches Forschungsinstitute).
Gravity deficit
The negative free air gravity anomaly in Wilkes Land appears to be partially due to the subglacial basin topography (Figs 4 & 5). However, the lowland subglacial topography is inadequate to account for the entire negative free air gravity anomaly. That is, the absolute magnitude of the negative gravity anomaly is greater than can be accounted for by topography alone. The additional mass deficit can be accounted for by the presence of a low density mass beneath the subglacial rock surface. The crystalline and metamorphic complexes that underlie the area have an average density of 2.67 gcm-3. Departures from this value may be explained by the presence of a substantial thickness of less dense material such as fluvial sediment, glacial drift or a low density breccia lens (Innes Reference Innes1961). The density of fluvial or glacial detritus is c. 2.20 gcm-3, and that of impact breccia c. 2.50 gcm-3. These density values, while sufficiently different from the density of crystalline or metamorphic country rock to account for the negative gravity portion of the larger anomaly, are also sufficiently different from one another to require substantially different thicknesses to account for the gravity deficit.

Fig. 4 Subglacial topographical image of the Wilkes Land Anomaly from Gravity Recovery and Climate Experiment (GRACE) satellite gravity-radar imaging. Regions of high elevation appear in red, those of lower elevation appear in yellow, and those of lowest elevation (oceanic regions) appear in blue.

Fig. 5 Subglacial topography of the Wilkes Land Anomaly from BEDMAP2 airborne radiosound survey, confirming the results of the Victoria Land Traverse and Gravity Recovery and Climate Experiment (GRACE) surveys (after Fretwell et al. Reference Fretwell, Pritchard and Vaughan2013; cf. Fig. 4.)
The deficit that must be explained by a lower density subsurface mass is 101.9 mgal. Given a country rock density of 2.67 gcm-3, the density contrast between country rock and sedimentary or glacial detrital material is 0.47 gcm-3. Therefore, the thickness of sedimentary fluvial or glacial detrital accumulation beneath the subglacial rock surface of the basin topography that is required to account for the balance of the negative free air gravity anomaly is:

where (0.04185) is g, the gravitational attraction in milligals, and d is the depth of the base of the sedimentary accumulation. A 5180 m accumulation of fluvial or glacial detritus requires that the base of the accumulation lie ≥5 km below sea level, and perhaps more given the present depth of the subglacial surface which extends as much as 1000 m below sea level (Steed & Drewry Reference Steed and Drewry1982, Fretwell et al. Reference Fretwell, Pritchard and Vaughan2013). The depth of erosion and subsequent deposition needed to produce a fluvial sedimentary or glacial detrital column of this thickness is inconsistent with fluvial and glacial theory, and with the geological history of northern Victoria Land. Nevertheless, it is useful to consider the possible effects of isostatic depression of the continent due to the continental ice sheet mass to account for such a sediment thickness, as with the presence of a subglacial valley.
The thickness of the continental ice sheet averages 2300 m, and East Antarctica is presently in isostatic equilibrium. The existing continental ice sheet is adequate to account for <12% of a 5 km depression of the base of an accumulation of fluvial or glacial sedimentary material. On the other hand, if the continental ice sheet were sufficiently thicker in the geological past, a greater isostatic depression may have been achieved. However, the former additional thicknesses of the East Antarctic continental ice appear to have been in the 600–700 m range, still dramatically inadequate to account for the isostatic depression required for the substantial sediment thickness needed, and thus inadequate to account for the free air gravity anomaly deficit.
Therefore, the alternative low density-related explanation for the gravity deficit is the presence of a breccia lens beneath the site. Breccia lenses of the size required are characteristic on Earth almost exclusively of meteoroid impact sites, and negative free air anomalies are common accompaniments of breccia lenses (Innes Reference Innes1961, Pike Reference Pike1974, Ugalde et al. Reference Ugalde, Artemieva and Milkereit2005, Grieve Reference Grieve2006). Determination of the gravity deficit of the WLA as a possible consequence of a breccia lens may be achieved by examining density contrasts. The density difference between unbrecciated country rock (2.67 gcm-3) and brecciated country rock (2.50 gcm-3) is 0.17 gcm-3, thus the depth of brecciation (d) required to explain the additional gravity deficit is:

This depth is well within the constraints of hypervelocity impact theory for a structure ≥200 km in diameter (Innes Reference Innes1961, Melosh Reference Melosh1989), and is consistent with the breccia lens thickness expected of an impact structure with a diameter of 510 km. Therefore, the additional mass deficit may be attributed to the presence of a breccia lens. This explanation does not require an appeal to substantial isostatic adjustment, given the smaller density contrast between brecciated and unbrecciated country rock. Therefore, the combination of subglacial basin topography and a breccia lens is adequate to explain the 101.9 mgal gravity deficit.
Regardless of the origin of the WLA subglacial topography, it is apparent that there has been deformation upstream and downstream (toward the coast) of the surface expression of the structure (Fig. 6a). The two parallel lowlands are overlain by two parallel ice streams, and appear as parts of the circular basin lowland of the Wilkes Land structure (Weihaupt et al. Reference Weihaupt, Chambers, van der Hoeven and Lorius2014b). Additionally, an overlying ‘footprint’ (in the continental ice sheet surface) of the subglacial topography is seen by RADARSAT imaging of the continental ice sheet surface (Fig. 6b; Jezek Reference Jezek2002, Weihaupt et al. Reference Weihaupt, Chambers, van der Hoeven and Lorius2014b). The subglacial topography (Fig. 6a) is oriented in the regional downslope direction, buttressing and depositional highlands appearing as an apparent peak ring along the axis of the structure (S in Fig. 7), and highlands appear as an apparent circular rim (t, u and w in Fig. 7), both having been deformed by the overlying ice. The triangular central highland at S is the result of ice movement, namely upslope buttressing and downslope deposition, the effect of the central peak ring. Still further downslope near the coast a lowland appears (v in Fig. 7), the apparent deformed continuation of the circular lowlands at r, again the result of glacier movement and more highland buttressing at w, which represents a portion of the deformed circular rim structure.
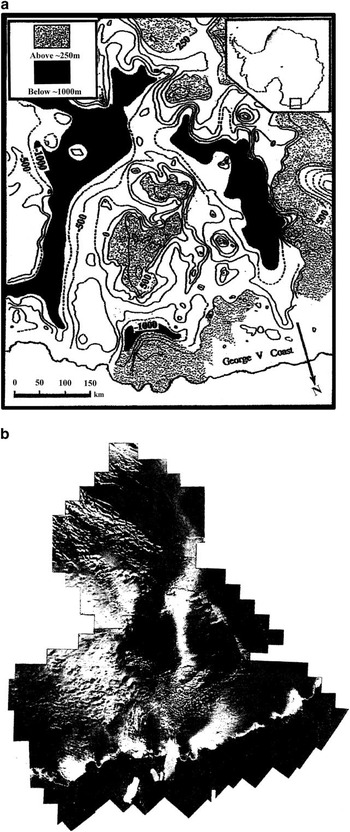
Fig. 6a Enlargement of the area of the subglacial topography of the Wilkes Land Anomaly (WLA) shown in Figs 4 & 5. Compiled from Victoria Land Traverse and Adélie Land Traverse ground-based gravity and seismic surveys (after Rouillon Reference Rouillon1960, Weihaupt Reference Weihaupt1961, Weihaupt et al. Reference Weihaupt, Rice and van der Hoeven2010, Weihaupt et al. Reference Weihaupt, Stuart, van der Hoeven, Lorius and Smith2012), from airborne radiosound survey (after Steed & Drewry Reference Steed and Drewry1982), from airborne gravity and magnetic survey (after Ferraccioli et al. Reference Ferraccioli, Coren, Bozzo, Zanolla, Gandolfi, Tabacco and Frezotti2001) and from the BEDMAP2 dataset (after Fretwell et al. Reference Fretwell, Pritchard and Vaughan2013). b. Mosaic of RADARSAT imaging of the continental ice sheet surface overlying the WLA, reflecting the subglacial morphology of the craterform structure. Note that there are two basins in the ice sheet surface divided by an elevation high along the central axis, and chaotic ice terrain downslope of the basins. Together these images appear as a large circular feature in the continental ice sheet surface (from Weihaupt et al. Reference Weihaupt, Chambers, van der Hoeven and Lorius2014b, after Jezek Reference Jezek2002).

Fig. 7 Circular morphology exhibited in the glacially deformed subglacial topography of the Wilkes Land Anomaly (WLA). r represents lowland basin topography (black), s represents the central highlands (stippled; the circle on the central highlands is 115 km in diameter). Rim structures (stippled) are represented by t, u and w (the outer circle is 510 km in diameter). A deformed lowland at v, a continuation of the basin lowland at r, occurs downslope of the central highland and upslope of the buttressing rim highland at w. Intermediate elevations are shown in white; contour elevations are in metres.
Figure 8a & b depicts the subglacial topography and profile of the WLA, reflecting the craterform nature of the structure. The elevations and locations of the peak ring and circular rim-like features are consistent with the peak ring and rim structures of known impact basins. The subglacial topographical structure of the WLA, elongated downslope as a degraded circular symmetric erosional and depositional feature with a degree of asymmetry, is therefore characteristic of a degraded impact crater (Plado et al. Reference Plado, Pesonen and Puura1999, Rebolledo-Vieyra et al. Reference Rebolledo-Vieyra, Urrutia-Fucugauchi and Lỏpez-Loera2010, Whitehead et al. Reference Whitehead, Grieve, Garvin and Spray2010) due to target irregularities or subsequent tectonic or erosional modification. Weathering and erosion are both capable of modifying such circular topography (Melosh Reference Melosh1989, Plado et al. Reference Plado, Pesonen and Puura1999, Rebolledo-Vieyra et al. Reference Rebolledo-Vieyra, Urrutia-Fucugauchi and Lỏpez-Loera2010, Whitehead et al. Reference Whitehead, Grieve, Garvin and Spray2010), as are subsequent tectonic events, producing structures that are nevertheless observable not only with gravity, seismic and radiosound surveys, but also with magnetic surveys (Morgan et al. Reference Morgan, Warner, Brittan, Buffler, Camargo, Christeson, Denton, Hildebrand, Hobbs, Macintyre, Mackenzie, Maguire, Marin, Nakamura, Pilkington, Sharpton, Snyder, Suarez and Trejo1997, Kinsland et al. Reference Kinsland, Pope, Cardador, Cooper, Cowan, Kobrick and Sanchez2005).

Fig. 8a Route of the Victoria Land Traverse (line W–X), route of the Adélie Land Traverse (line X–Y–Z), and the northern portion of the profile (line X–Y’–Z’). b. Profile of the subglacial topography of the Wilkes Land Anomaly (along W–X–Y’–Z’). The rim-like highlands (t, u and w of Fig. 7) are projected onto this profile for reference.
Magnetic signature of the Wilkes Land Anomaly
Magnetic signatures are characteristic of a variety of structures in Earth’s lithosphere and are capable of revealing the depth to magnetic sources, magnetic susceptibility differences between rock units, buried rock contacts, linear and circular tectonic patterns, subsurface geology and structure, subglacial topographical variations, and the dimensions of magnetically susceptible structures (Rebolledo-Vieyra et al. Reference Rebolledo-Vieyra, Urrutia-Fucugauchi and Lỏpez-Loera2010). Combined gravity and magnetic anomalies, which characteristically parallel one another, have the potential to provide even more detail about buried geological features. Aeromagnetic anomalies in the vicinity of the WLA are particularly useful for identifying structural, stratigraphic, topographical and subglacial geological features (Ferraccioli et al. Reference Ferraccioli, Coren, Bozzo, Zanolla, Gandolfi, Tabacco and Frezotti2001, Rebolledo-Vieyra et al. Reference Rebolledo-Vieyra, Urrutia-Fucugauchi and Lỏpez-Loera2010).
Magnetic surveys in the vicinity of the WLA (Ferraccioli et al. Reference Ferraccioli, Coren, Bozzo, Zanolla, Gandolfi, Tabacco and Frezotti2001) (Fig. 9) may reflect topography, as well as crustal structure beneath the subglacial rock surface. In particular, the central portion of the Wilkes Land feature, marked by positive free air gravity, is also marked by high amplitude magnetic anomalies much the same as traditionally observed in the topography and crustal structure of impact basins. The lowland basin topography of the WLA, marked by negative free air gravity, is also marked by a magnetic low characteristic of topographical lowland, as well as impact melt and breccia lenses like those observed for virtually all impact basins in the solar system, and eroded ancient terrains. The magnetic lows created by impact events result in part from thermoremnant magnetization of the target material caused by the shock of meteorite impact (Plado et al. Reference Plado, Pesonen and Puura1999), as well as structural or topographical effects. The magnetic signature of the WLA may, therefore, be due to an ancient eroded valley or to an impact basin. The presence of two ice streams and a chaotic ice surface, and of an overlying ‘footprint’ in the surface of the continental ice sheet (Fig. 6b), may be explained on the basis of either.

Fig. 9 Subglacial topography and crustal structure of the Wilkes Land Anomaly revealed by airborne magnetic survey. The image depicts features consistent with those revealed by gravity, seismic, radiosound and satellite surveys (after Ferraccioli et al. Reference Ferraccioli, Coren, Bozzo, Zanolla, Gandolfi, Tabacco and Frezotti2001).
Comparison of the Wilkes Land Anomaly structure with known impact structures
Having examined the evidence favouring the erosion of ancient terrain, we next examine the evidence favouring impact as the cause of the WLA. Such an examination requires consideration of the dimensions, aspect ratios and history of impact structures on terrestrial objects. The circular footprint of the subglacial topography, the gravity and magnetic anomalies and in the overlying continental ice sheet surface define a geometry and structure that are diagnostic of large impact structures.
A large number of known impact craters on Earth and elsewhere in the solar system were examined (Pike Reference Pike1974, Melosh Reference Melosh1989, Ugalde et al. Reference Ugalde, Artemieva and Milkereit2005, Grieve Reference Grieve2006, Whitehead et al. Reference Whitehead, Grieve, Garvin and Spray2010). In the case of small impact craters the gravity signature is normally that of a circular negative free air gravity anomaly (Innes Reference Innes1961). For large impact craters and basins a positive free air gravity anomaly and a magnetic high anomaly characteristically occur in the centre of the structure representing a central peak ring. These frequently indicate the presence of a mascon (Melosh et al. Reference Melosh, Freed, Johnson, Blair, Andrews-Hanna, Neumann, Phillips, Smith, Solomon, Wieczorek and Zuber2013), while the surrounding negative gravity anomaly represents basin lowland and subsurface impact melt and brecciation.
Gravity and magnetic signatures similar to the WLA reported elsewhere in Antarctica are observed in both continental and offshore regions (Behrendt et al. Reference Behrendt, Flinn, Blankenship and Bell1998, Weihaupt et al. Reference Weihaupt, Rice and van der Hoeven2010). While each of these constitutes a separate study, it should be noted that the ages of these anomalies are presently undetermined. The impact crater explanation is ultimately dependent upon demonstrating the presence or absence of traditional impact crater evidence, such as, free air gravity and magnetic anomalies and patterns, gravity surpluses and deficits, craterform morphology, brecciation, impact melts, shocked minerals, shatter cones, tektites, aspect ratios, and consistency with the characteristics of impacting projectiles and solar system impact history. While some of these properties are known for the WLA through direct and remote observation, others are not because of the overlying continental ice sheet. The presence of brecciation, impact melts, shock minerals and shatter cones (Robolledo-Vieyra et al. Reference Rebolledo-Vieyra, Urrutia-Fucugauchi and Lỏpez-Loera2010) cannot be confirmed or ruled out at this time. However, subglacial craterform morphology, gravity anomalies, magnetic anomalies and low density subglacial substrate are evident. Similarly, projectile characteristics and the consequent basin morphology can be calculated on the basis of impact theory. This evidence allows comparison of the WLA with known impact basins on the basis of craterform morphology, basin dimensions and the basin-forming capacity of impact projectiles; as well as with the solar system impact record, i.e. the times and frequencies of meteoroid impacts in the solar system.
Pike (Reference Pike1974) noted that the scaled dimensions of complex craters on Earth resemble large craters and basins on the Moon, Mars and Mercury. The peak rings of such structures are also normally ringed by negative gravity anomalies, and these are sometimes ringed in turn by positive free air gravity anomalies representing portions of circular highlands (the crater rims), unless the rims are diminished by erosion (Melosh Reference Melosh1989, Collins et al. Reference Collins, Melosh and Marcus2005). This description is consistent with the free air gravity anomalies, magnetic anomalies, and associated buried topography of the WLA which displays a basin diameter of 510 km, a crater rim width of 35 km, and a crater rim height of 2.0 km. The depth of the craterform structure in Wilkes Land is 2.3 km, in good agreement with scaling relationships provided by Collins et al. (Reference Collins, Melosh and Marcus2005) which calculate a depth of 1.97 km for a structure of this size. The diameter of the peak ring structure of the WLA is 115 km, also in good agreement with Pike’s (Reference Pike1974) determination that the diameter of peak rings falls in the range of 20–25% or less of the crater diameter.
The relationships between the properties of impact craters and the properties of the generating projectiles, similarly enables us to model the size, density, velocity and impact angles of projectiles capable of creating the 510 km diameter of the WLA (Melosh et al. Reference Melosh, Freed, Johnson, Blair, Andrews-Hanna, Neumann, Phillips, Smith, Solomon, Wieczorek and Zuber2013). Noting that impacting meteoroids may be asteroids or comets, these data provide an opportunity to determine whether the required projectiles existed and were available in the past to create a feature like the WLA, and to compare the morphology and dimensions of the WLA with the morphology and dimensions of basins modelled on the basis of projectile characteristics (Table I). Table I shows the summary of a compilation of 176 projectile values capable of generating a 510 km diameter basin in a target with a density of 3000 kgm-3 and gravitational acceleration of 9.8 m sec-2 (the gravitational acceleration of Earth). Of the potential projectiles modelled, six were found to have the requisite combination of diameters, densities, velocities and impact angles to create an impact basin with diameters ranging from 470–525 km, bracketing the 510 km diameter of the WLA. The velocity of 17 km sec-1 represents the most common velocity anticipated for Earth impacts, and the velocity of 72 kmsec-1 represents the maximum velocity anticipated for retrograde impacts. The frequency of projectiles with these dimensions was highest in Earth’s early history during the period of intense bombardment 4.1 to 3.8 Ga, and declined with geological time, although large impacts are evident in the solar system in much more recent time, e.g. the Shoemaker-Levy impacts on Jupiter. In conclusion, from these projectile and related parameters, the WLA could have been created by such bolides.
Table I Parameter combinations capable of yielding an impact basin with a diameter of c. 510 km, based upon a target density of 3000 kg m-3 and a gravitational acceleration of 9.8 m sec-2.

In addition, the topographical expression and profile of the WLA (Fig. 10a & b) is essentially the same as that modelled on the basis of impacting projectiles (Fig. 10c & d; the diameters, densities, velocities and impact angels of probable projectiles). The basin properties include diameter, depth, rim width and peak ring diameter. The actual WLA basin and modelled basin are shown in Fig. 10 to be much the same. Similarly, the aspect ratios of the WLA, i.e. the relationships between the basin diameter, rim width, depth, peak ring dimensions and ejecta blanket width, can be compared with the aspect ratios of know impact craters and basins (Pike Reference Pike1974, Melosh Reference Melosh1989, Morgan et al. Reference Morgan, Warner, Brittan, Buffler, Camargo, Christeson, Denton, Hildebrand, Hobbs, Macintyre, Mackenzie, Maguire, Marin, Nakamura, Pilkington, Sharpton, Snyder, Suarez and Trejo1997, Walsh et al. Reference Walsh, Holloway, Habdas and de Bruyn2003). These relationships, shown in Fig. 11 for the WLA, are plotted with other craters and basins in the solar system, the data were acquired from the United States Aeronautical Chart and Information Center and other investigators including Pike (Reference Pike1974). The aspect ratios for crater rim width and crater diameter (Fig. 11a), crater depth and crater diameter (Fig. 11b), and crater rim height and crater diameter (Fig. 11c) are shown to be essentially the same for the Wilkes Land structure as for other Earth, Moon and Martian craters and basins.
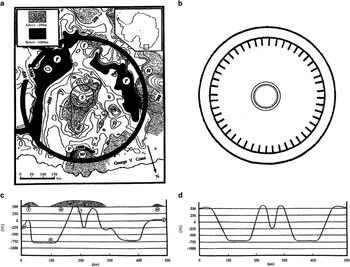
Fig. 10a Subglacial topography and b. profile of the Wilkes Land Anomaly (WLA), compared with models of the c. plan and dimensions and d. profile of comparable known impact basins, based upon Melosh & Beyer’s 2004 programs for determining crater characteristics from impact projectile parameters, projectile diameter, density, velocity and impact angle (Table I). The features of the WLA are found to be comparable to the features of known impact basins, and comparable to basins modelled on the basis of known projectile characteristics.

Fig. 11 Aspect ratios of the Wilkes Land Anomaly (WLA) and of known impact basins in the solar system. a. The relationship between crater rim width and crater diameter for the WLA and known Earth and Moon craters (US Aeronautical Chart and Information Center 1999) (after Pike Reference Pike1974, Walsh et al. Reference Walsh, Holloway, Habdas and de Bruyn2003). b. The relationship between crater depth and crater diameter for the WLA, Earth craters and Martian basins (after Walsh et al. Reference Walsh, Holloway, Habdas and de Bruyn2003). c. The relationship between crater rim height and crater diameter for the WLA and Earth and Moon craters (after Pike Reference Pike1974, Walsh et al. Reference Walsh, Holloway, Habdas and de Bruyn2003).
The aspect ratios and modelling enable us to examine the WLA in terms of known hypervelocity impact basins in the solar system, to determine whether the WLA is comparable to such structures. This examination reveals a large inventory of basins the size of the structure reported in Antarctica. These include the Moon’s Schroedinger Crater (320 km) and Mercury’s Rembrandt Crater (700 km), which exhibit craterform properties and aspect ratios like those discussed above. Larger basins confirm the impacts of even larger meteoroids, such as that of Caloris Basin (1550 km) on Mercury, and Hellas (2300 km) and Borealis (5300 km) basin on Mars, which also exhibit properties and aspect ratios much the same as those described above. The largest known craterform structures on Earth are the Vredefort, Sudbury and Chicxulub structures, and the subglacial structure in Wilkes Land. All of these impact basins display comparable aspect ratios and morphology, and all have undergone post-impact degradation not unlike that of Chicxulub (Rebolledo-Vieyra et al. Reference Rebolledo-Vieyra, Urrutia-Fucugauchi and Lỏpez-Loera2010) and of the Wilkes Land structure.
In contrast, and in spite of the fact that some 180 impact structures have been identified on Earth, few are of the size of the impact basins found on other terrestrial objects in the solar system. Most of Earth’s earliest and largest structures have disappeared, eroded by atmospheric processes, destroyed by the planet’s plate tectonics, or concealed by the ocean, sedimentation or continental ice sheets (Collins et al. Reference Collins, Melosh and Marcus2005).
Conclusion
The presence of two large ice streams and a chaotic ice surface in the continental ice sheet can be explained by the complex subglacial topography that exhibits two parallel lowlands separated by a central buttressing highland. The complex subglacial topography, in turn, may be explained in part by the effects of inherited ancient terrain, erosion and deposition, but not solely by such processes. The circular morphology, uncharacteristic of glacial erosion and of most tectonic structures, implies that another mechanism is responsible for the WLA. In contrast, the presence of the substantial gravity anomalies can only be partially explained by the subglacial topography. The existence of subglacial material with a density lower than that of the surrounding country rock is adequate to account for the gravity deficit. However, the association of a positive free air gravity anomaly with a surrounding negative free air gravity anomaly of this magnitude cannot be easily explained by erosion or tectonic structures. The magnitude of the gravity anomalies and the close association of both positive and negative gravity anomalies could be explained by an impact structure. Magnetic anomalies parallel the gravity anomalies, and together define a circular footprint paralleling the circular subglacial topography. The magnetic anomalies are particularly important, as they are generated by both topography and lithospheric structure, and therefore support the possibility of impact-generated geological structure.
We began this investigation citing four questions that must be answered in order to explain the WLA: i) What is the most likely explanation for the association of the gravity anomalies with the complex subglacial topography? ii) What is the significance of the associated magnetic anomalies? iii) Are the properties of the WLA comparable to the properties of other similar structures? iv) What, therefore, is the most likely origin of the WLA and its associated features? These observations, including the modelling of the WLA, calculation and modelling of potential crater-generating projectiles, the similarity of the characteristics of the WLA to those of known impact basins, and consistency of the properties of the WLA with meteoroid impact theory and history, favour the suggestion that the WLA is an impact site, as will the presence of diapiric material, brecciation, impact melt or shocked minerals, if observed. Nonetheless, because of the constraints imposed by the overlying continental ice sheet, and the paucity of other investigations of the area of the WLA, we believe that the other explanations for the subglacial structure remain viable, although that of an impact crater most clearly satisfies the data presently available. Because of the potential importance of the gravity characteristics of the WSB for the geoid of the entire continent, and the potential of the WLA to provide more pertinent information regarding its origin, we encourage further geophysical investigation. We also encourage drilling onshore and offshore for additional evidence of impact, such as diapiric material, brecciation, impact melt or shocked minerals; evidence that will satisfy the inventory of the characteristics of major meteoroid impact.
Acknowledgements
We wish to express our appreciation first to other colleagues of the United States Victoria Land Traverse who accompanied and assisted us in the field, A.W. Stuart, A.J. Heine, L. Roberts, W.M. Smith, A. Taylor, T. Baldwin and W. Jackman. We wish to thank also the Editor and reviewers of the manuscript who made valuable comments and critical suggestions. We appreciate as well the U.S. National Science Foundation, the Arctic Institute of North America, the University of Colorado, Delft Technical University, Laboratoire de Glaciologie et Geophysique de Environment, the Institute of Arctic and Alpine Research, University of Colorado Boulder, and the University of Wisconsin for administrative and financial support.
Author contributions
John Weihaupt, Frans G. van der Hoeven, Claude Lorius and Devin Castendyk: members of the field research teams. John Weihaupt: manuscript, research, editing and figures. Frederick B. Chambers: manuscript, research, editing and figures. John W. Wyckoff: manuscript, research, editing and figures.