Introduction
Starches produced by higher plants function as seed storage reserve carbohydrates and are probably the most important dietary source of energy for humans, representing a major proportion of the daily calorific intake. Plant starches are synthesized inside specialized subcellular organelles called plastids and provide the cell and tissue with the means to store high-density energy in the form of carbohydrate. Transient starches are synthesized in leaf chloroplasts during the day and degraded at night to provide carbon for non-photosynthetic metabolism. Starch produced in tuberous tissues also acts as a carbon store, and may need to be accessed as environmental conditions dictate, while storage starches in developing seeds, such as cereal endosperms, are a long-term carbon store for the next generation. All storage starches produced in heterotrophic tissues are made inside specialized plastids termed amyloplasts. The storage starches produced in cereal endosperm amyloplasts account for over 90% of the world market for starch (the major cereals in terms of tonnage of yield are maize and wheat). Starch is a cheap, natural renewable raw material, the varied structures of which are exploited in the agri-food sector, in many different industrial applications and as a source of energy after conversion to ethanol (Röper, Reference Röper2002; Smith, Reference Smith2008), making it a versatile and highly useful commodity (Burrell, 2003). Many of the desired physicochemical properties underpinning the different uses of starches can be produced and altered through chemical and enzyme modification, and physical treatment (Wurzburg, Reference Wurzburg and Wurzburg1986; Jobling, 2004; Xie and Liu, Reference Xie and Liu2004).
Characterization of natural mutants through plant breeding, and identification and isolation of the major genes encoding the core components of the starch biosynthetic pathway have provided a basic understanding of starch granule formation (see recent review by Keeling and Myers, Reference Keeling and Myers2010). Knowledge of the basic pathway has led to the production of a number of functional starches based upon predictions of their structures resulting from various mutations/gene deletions (see below). However, there still remain significant gaps in our understanding of the pathway, in particular how the growth of starch granules is initiated and terminated, and important details regarding the regulation and coordination of the many enzyme activities involved in polymer synthesis. Such information is a prerequisite for the rational production of ‘designer’ starches in planta, which will reduce the need for costly and environmentally hazardous post-harvest chemical treatments.
The following is a review of recent developments in our understanding of starch biosynthesis in higher plants, with an emphasis on production of storage starches in the developing seeds of cereals. I will present a brief overview of the biological significance of starch synthesis in the context of the developing seed, discuss the origins of the starch biosynthetic pathway in higher plants, outlining some emerging ideas, and then present a current overview of starch structure, the core pathway involved in its synthesis and recent developments in our understanding of regulation of the pathway at the post-translational level.
Seeds as storage organs
Human civilization is dependent on the agricultural production of seeds which provide the majority of the world's staple foods, such as cereals and legumes, and in addition provide an important source of industrial biomaterial. The development of seeds has given the Angiosperms a great selective advantage, allowing fertilization in a non-aqueous habitat and providing an ideal dispersal unit with long-term viability during quiescent periods due to the evolution of desiccation-tolerant embryos. Seeds are produced following reproduction, and an important requirement for the survival of seeds is the development of long-term carbon stores to power eventual seedling establishment. These can be in the form of starch, lipid or hemicelluloses and are deposited in storage cells usually in the cotyledons or persistent endosperm. The relative partitioning of assimilated carbon (usually in the form of sucrose) into different storage compounds in different tissues is regulated by a complex interplay of gene expression and metabolic activity during seed development (Lin et al., Reference Lin, Sun, Nguyen, Rachubinski and Goodman1999; Wobus and Weber, Reference Wobus and Weber1999; Rolletschek et al., Reference Rolletschek, Koch, Wobus and Borisjuk2005). Starch is present in most plant tissues as a carbon store, and in many agriculturally important crops, notably the cereals, it can represent as much as 70% of the dry weight of the seed (Dale and Housely, Reference Dale and Housely1986). The structure and composition of starch make it an ideal storage material in seeds as it is chemically inert and water insoluble, allowing large amounts of sugar to be stored in cells without adverse effects on solute potential. Starch is deposited in endosperm cells shortly after a period of rapid cell division and expansion of cell volume following pollination (Briarty et al., Reference Briarty, Hughes and Evers1979; Olsen, Reference Olsen2001). The number of endosperm cells formed may be a strong indicator of final yield (Brocklehurst, Reference Brocklehurst1977). This cell division phase is completed within a few days [2–4 days after pollination (DAP) in wheat, and 4–6 DAP in maize], and cell volume increases up to maturity (approximately 35 DAP). The deposition of storage material usually occurs between 10 DAP and maturation (Bewley and Black, Reference Bewley and Black1994). The following reviews our current knowledge of starch biosynthesis in plant tissues, with an emphasis on the storage starches produced in amyloplasts of cereal endosperms.
Overview of the origins of the starch biosynthetic pathway in plants
Polyglucans are the most important and widespread form of reserve carbohydrate found in nature; glycogen and starch being the predominant forms. Both glycogen and starch are comprised of glucose chains (glucans) linked in an α-(1 → 4) configuration, and branched at α-(1 → 6). Glycogen is a homogeneous water-soluble polymer with relatively uniformly distributed branches (Roach, Reference Roach2002) and is found within the archaea, bacteria and certain eukaryotes. Starch is made up of amylose (a largely unbranched, minor component) and amylopectin (an asymmetrically branched major component) and is present in the cytoplasm of Rhodophyceae (red algae) and Glaucophyta (Dauvillée et al., Reference Dauvillée, Deschamps, Ral, Planke, Puteaux, Devassine, Durand-Terrasson, Devin and Ball2009), but is confined to the plastid stroma of Chloroplastida (green algae and land plants). In fact, starch synthesis is restricted to the Archaeplastida, whose origins are thought to be via a single endosymbiotic event involving ancestors of cyanobacteria and a heterotrophic host (Cavalier-Smith, Reference Cavalier-Smith2009), rendering the organelle known as the plastid, which is capable of oxygenic photosynthesis. Recent phylogenetic studies indicate that the plastidial starch pathway is complex, and made up of genes with both cyanobacterial and eukaryotic origins (Patron and Keeling, Reference Patron and Keeling2005; Deschamps et al., Reference Deschamps, Moreau, Worden, Dauvillée and Ball2008a), and is in sharp contrast to the lower-complexity pathway of cytosolic starch synthesis found in the Rhodophyceae and Glaucophyta (Deschamps et al., Reference Deschamps, Haferkamp, d'Hulst, Neuhaus and Ball2008b). Phylogenetic analysis of the enzymes of the starch biosynthetic pathway strongly suggests that the pathway was originally cytosolic (in the common ancestor of the Archaeplastida), and then re-directed (reconstructed) to plastids via three discrete steps (see Deschamps et al., Reference Deschamps, Moreau, Worden, Dauvillée and Ball2008a for a detailed discussion), leaving some enzymes involved in the metabolism of malto-oligosaccharides (MOS) and amylopectin degradation in the cytoplasm. The three evolutionary steps involved: (1) plastidial synthesis of unbranched MOS; (2) glycogen synthesis (including priming steps and branching activities); and (3) plastidial starch synthesis, resulting in the eventual loss of cytosolic starch synthesis. Interestingly, the proposed timing of the relocation of the starch synthesis pathway to plastids coincides with the evolution of light-harvesting complexes, and it has been suggested that the presence of starch, and its degradation via starch phosphorylase, could generate the required ATP to overcome protoporphyrin IX-induced oxidative stress, thus imparting improved fitness (evolutionary benefit) to the organism (Deschamps et al., Reference Deschamps, Moreau, Worden, Dauvillée and Ball2008a).
Overview of starch structure
The starch reserves found in the tissues of higher plants are packaged as discrete granules inside plastids. The structure of the starch granule is complex and has a hierarchical order composed of two distinct types of glucose polymer: amylose, a polymer of 100–10,000 glucosyl units comprising sparsely branched α-(1 → 4)-O-linked glucan chains, and amylopectin, a larger, highly and regularly branched glucan polymer typically constituting about 75% of the granule mass, produced by the formation of α-(1 → 6)-linkages between adjoining straight glucan chains. There is approximately one branching point for every 20 glucose residues in amylopectin, which is approximately half the value normally found in glycogen. Over the years many models have been proposed for the structure of amylopectin and its organization into granules; however, the cluster model has emerged as the most likely structure (Fig. 1). The polymodal distribution of glucan chain lengths and branch point clustering within amylopectin allows the short linear chains to pack together efficiently as parallel left-handed double helices (with approximately six monomers per turn, with a pitch of 2.13 nm). These glucan chains cluster in organized arrays, forming the basis of the semi-crystalline nature of much of the matrix of the starch granule, and resulting in the water-insoluble nature of starch. Granule formation is driven by both the semi-crystalline properties of amylopectin, as determined by the length of the linear chains of amylopectin, and the clustering and frequency of α-(1 → 6)-linkages (Robin et al., Reference Robin, Mercier, Charbonniere and Guilbot1974; French, Reference French, Whistler, BeMiller and Paschall1984; Hizukuri, Reference Hizukuri1986; Myers et al., Reference Myers, Morell, James and Ball2000). The products of partial α-amylolysis of various cereal starches, resulting from the non-random hydrolysis of glucosidic bonds between unit clusters (known as long B-chains, or B2 and B3 chains, depending on the number of clusters interconnected), are fully consistent with the cluster model for amylopectin (Bertoft, Reference Bertoft1986). Pairs of adjacent chains within the clusters form double helices which help pack the glucan chains into crystalline layers (lamellae). At a higher order of organization, the lamellae form repeat units with a periodicity of approximately 9 nm. Groups of these semi-crystalline and amorphous lamellae associate into units termed blocklets (Gallant et al., Reference Gallant, Bouchet and Baldwin1997), which in turn associate into large crystalline structures several hundred nanometres wide, termed growth rings. These are visible by light and scanning electron microscopy following treatment of broken granules with acid or amylolytic enzymes (Buttrose, Reference Buttrose1960; Zeeman et al., Reference Zeeman, Pilling, Tiessen, Kato, Donald and Smith2002; Pilling and Smith, Reference Pilling and Smith2003). The crystalline structure of starch granules is highly conserved in higher plants at the molecular level (Kainuma, 1988; Jenkins et al., Reference Jenkins, Cameron and Donald1993; Zeeman et al., Reference Zeeman, Pilling, Tiessen, Kato, Donald and Smith2002), as well as at the microscopic level, where growth rings have been observed in all the higher plant starches studied (Buttrose, Reference Buttrose1960; Hall and Sayre, Reference Hall and Sayre1973; Pilling and Smith, Reference Pilling and Smith2003). By contrast, glycogen produced by animals and bacteria, including many cyanobacteria, has a more homogeneously and highly branched (approximately 10%) open structure which expands in a globular fashion (Roach, Reference Roach2002) resulting in a water-soluble polymer. However, certain cyanobacteria synthesize a polyglucan, termed semi-amylopectin, which is intermediate between amylopectin and glycogen in terms of the α-(1 → 4)-chain-length distribution (Nakamura et al., Reference Nakamura, Takahashi, Sakurai, Inaba, Suzuki, Nihei, Fujiwara, Tsuzuki, Miyashita, Ikemoto, Kawachi, Sekiguchi and Kurano2005). For more detailed reviews of starch structure models, see Manners (Reference Manners1989), Buléon et al. (Reference Buléon, Colonna, Planchot and Ball1998), Thompson (Reference Thompson2000) and Bertoft (Reference Bertoft2004).

Figure 1 Schematic representation of the basic structural unit of amylopectin – the cluster model. The starch granule (which may be as much as 50 μm in length) is made up of many regions of alternating amorphous and semi-crystalline zones, several hundred nanometres in width; pairs of these constitute a single growth ring. The growth rings, and the more structured elements of the granule, are organized within an amorphous background containing amylopectin in a less ordered state, and amylose interspersed between these amorphous zones and the amylopectin clusters. In the tandem-linked glucan clusters shown, clusters of parallel α-(1 → 4)-O-linked glucan chains of short–intermediate length [degree of polymerization (DP) ≥ 10] glycosyl units pack together to form double helices, contributing to the water-insoluble properties of the polymer. The amorphous lamellae are characterized by clustered α-(1 → 6)-linked glucosidic linkages (branch-points) and longer glucan chains connecting the crystalline lamellae. The length of the crystalline and amorphous regions (approximately 9–10 nm) appears to be highly conserved, irrespective of the source of the starch. Growth of the polymer is from the non-reducing end in the direction of the arrow.
The precise location of amylose within the granule is a matter of much debate, but it is thought to be predominantly interspersed in a single-helical or random-coil form in the amorphous, less crystalline regions (Jane et al., Reference Jane, Xu, Radosavljevic and Seib1992; Jenkins and Donald, Reference Jenkins and Donald1995) and synthesized within a pre-existing amylopectin matrix. Amylose is readily leached from starch granules, suggesting weak associations with the amylopectin granule matrix (Cowie and Greenwood, Reference Cowie and Greenwood1957).
A common feature of all starches is that, at some point, they must be degraded (e.g. seed storage starches following germination, and leaf starch at the end of the light period), and hence the granule structure must have built-in entry points (either channels, cavities or zones of attack) for the enzymes involved in its degradation (Fannon et al., Reference Fannon, Hauber and BeMiller1992; Huber and BeMiller, Reference Huber and BeMiller1997).
The synthesis of this architecturally complex polymer assembly is achieved through the coordinated interactions of a suite of starch biosynthetic enzymes, including some that have traditionally been associated with starch degradation. The complement of these starch metabolic enzymes is well conserved between plastids in tissues that make different types of starches, for example, transitory starch (made in chloroplasts) and storage starch (made in amyloplasts). With few exceptions, the various isoforms of the many starch metabolic enzymes can be found in both chloroplasts and amyloplasts, and the amino acid sequences of the enzymes involved in starch metabolism are highly conserved (Jespersen et al., Reference Jespersen, MacGregor, Henrissat, Sierks and Svensson1993; Smith et al., Reference Smith, Denyer and Martin1997; Ball and Morell, Reference Ball and Morell2003). This remarkable conservation in form and function of the starch biosynthetic enzymes is highlighted in a recent study by Sawada et al. (Reference Sawada, Francisco, Aihara, Utsumi, Yoshida, Oyama, Tsusuki, Satoh and Nakamura2009) which demonstrated complementation of function by expressing a starch branching enzyme from the alga Chlorella in transgenic rice. In addition, mutations in analogous starch biosynthetic and degradative genes in higher plants show consistent trends, which illustrates conservation of their biological roles, although their impact varies depending upon the genetic background. Models have been proposed which attempt to explain how the observed structure of starch is synthesized, based upon knowledge of the enzymes of the core pathway, essentially derived from in vitro experimental evidence and analysis of mutants (Ball et al., Reference Ball, Guan, James, Myers, Keeling, Mouille, Buléon, Colonna and Preiss1996; Waigh et al., Reference Waigh, Perry, Reikel, Gidley and Donald1998; Zeeman et al., Reference Zeeman, Umemoto, Lue, Au-Yeung, Martin, Smith and Chen1998; Nakamura, Reference Nakamura2002). The following section summarizes our knowledge of the known components of the starch biosynthetic pathway in higher plants, beginning with the formation of ADP-glucose from common metabolic intermediates (hexose-phosphate and ATP), and ending with the architecturally complex starch granule. Figure 2 presents an overview of the pathway of storage starch biosynthesis in heterotrophic plastids from developing monocot and dicot seeds.
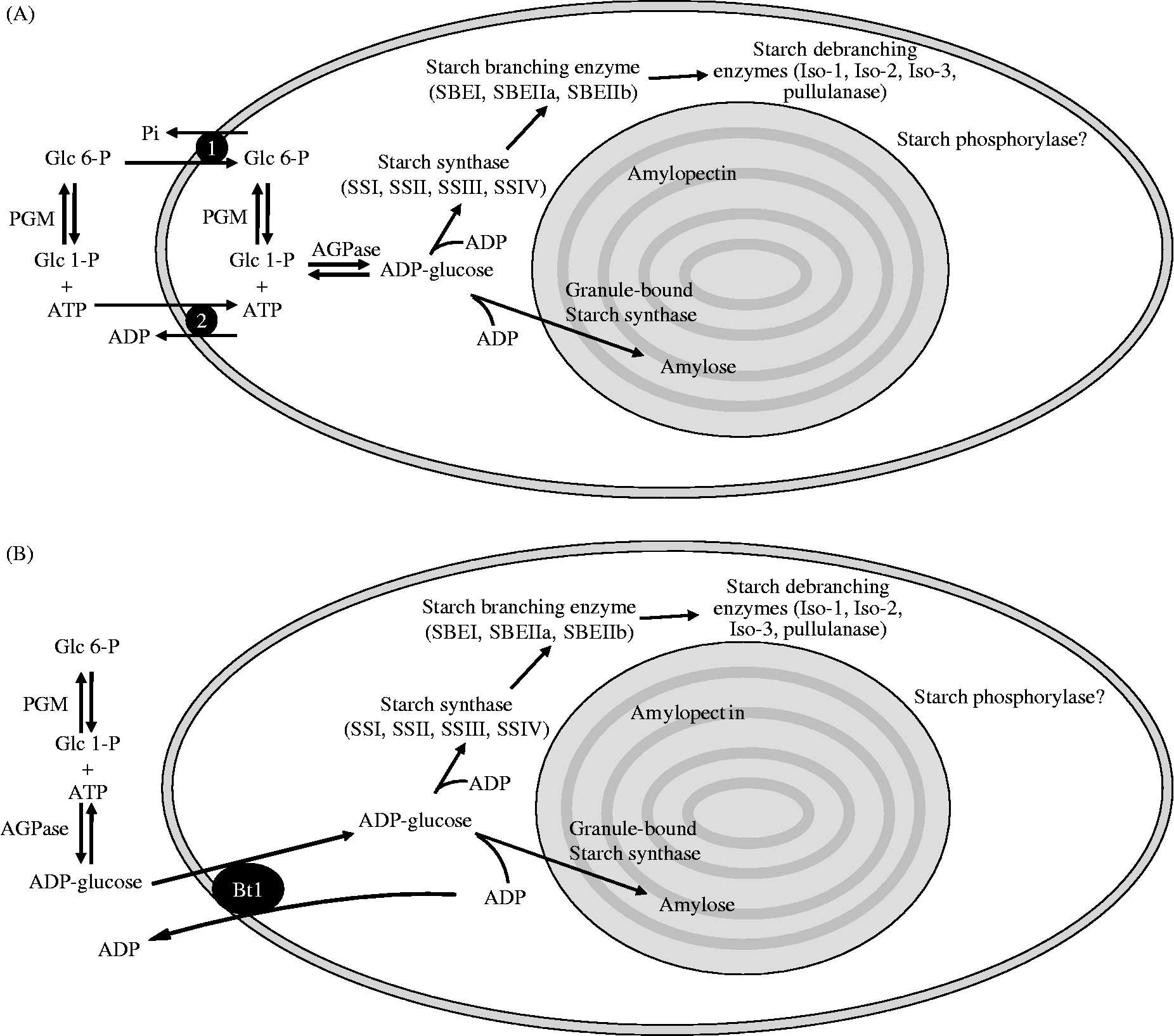
Figure 2 Overview of storage starch biosynthesis in the heterotrophic plastids (amyloplasts) of seed storage tissues. (A) The pathway of starch biosynthesis in storage tissues of dicots, where AGPase, responsible for the synthesis of ADP-glucose, is exclusively localized in the plastid stroma. Hexose-phosphates and ATP for this reaction are imported into the plastid from the cytosol via, respectively, the Glc 6-P/Pi antiporter (1) and the ATP/ADP transporter (2) located in the inner envelope membrane of the plastid. Cytosolic and plastidial isoforms of phosphoglucomutase (PGM) interconvert Glc 6-P and Glc 1-P. The antiport substrates for these transporters are generated from plastidial reactions involved in starch synthesis; Pi is generated from pyrophosphate produced by AGPase, and ADP is a by-product of the starch synthase (SS) reaction. ADP-glucose is utilized by the SSs, and the glucan chain branched and debranched by starch branching enzymes (SBE) and debranching enzymes, respectively, to form amylopectin. Granule-bound starch synthase also uses ADP-glucose, and is exclusively involved in amylose synthesis. (B) The pathway of starch synthesis operating in the endosperms of monocots such as cereals. These plants possess a cytosolic form of AGPase and import ADP-glucose from the cytosol via an ADP-glucose/ADP transporter termed Bt1. Monocots also possess a plastidial form of AGPase (as seen in A), but much of the AGPase in these tissues is extra-plastidial.
The pathway of starch biosynthesis
The formation of ADP-glucose by ADP-glucose pyrophosphorylase
Higher plant tissues capable of starch biosynthesis employ a prokaryote-like pathway for the formation of adenosine 5′-diphosphate glucose (ADP-glucose) (Greenberg and Preiss, Reference Greenberg and Preiss1964) which is the soluble precursor and substrate for starch synthases (Recondo and Leloir, Reference Recondo and Leloir1961). ADP-glucose is synthesized by ADP-glucose pyrophosphorylase (AGPase, E.C. 2.7.7.27). The AGPase reaction is the first committed step in the biosynthesis of both transient starch in chloroplasts and chromoplasts, and storage starch in amyloplasts, and is subject to different mechanisms of post-translational regulation (see below). It also catalyses a rate-limiting step in glycogen synthesis in bacteria. The reaction scheme for AGPase is as follows:

Thus, AGPase catalyses an equilibrium reaction. In vivo, the plastidial reaction is shifted away from equilibrium in favour of ADP-glucose synthesis by the action of plastidial alkaline inorganic pyrophosphatase (E.C. 3.6.1.1) (Gross and ap Rees, Reference Gross and ap Rees1986) that converts inorganic pyrophosphate (PPi) into inorganic phosphate (Pi), which can be transported across the plastid envelope membrane via different routes (for a review see Weber et al., Reference Weber, Schwacke and Flügge2005). AGPase from higher plants is heterotetrameric, consisting of two large (AGP-L) subunits and two small (AGP-S) catalytic subunits encoded by at least two different genes (Preiss and Sivak, Reference Preiss, Sivak, Zamski and Schaffter1996). In addition to the plastidial AGPase present in all starch-synthesizing tissues, biochemical evidence indicates the presence of at least two distinct AGPase enzymes in the developing endosperms of maize (Denyer et al., Reference Denyer, Dunlap, Thorbjørnsen, Keeling and Smith1996b), barley (Thorbjørnsen et al., Reference Thorbjørnsen, Villand, Denyer, Olsen and Smith1996a), rice (Sikka et al., Reference Sikka, Choi, Kavakli, Sakulsingharoj, Gupta, Ito and Okita2001) and wheat (Tetlow et al., Reference Tetlow, Davies, Vardy, Bowsher, Burrell and Emes2003b) which correspond to plastidial and cytosolic isoforms of AGPase. Further analyses have shown that cytosolic AGPase is restricted to the endosperms of the agriculturally important Graminaceae (Beckles et al., Reference Beckles, Smith and ap Rees2001). Starch synthesis, and therefore ADP-glucose formation, via a cytosolic AGPase is likely to be very dependent upon the activities of cytosolic PPi-consuming reactions which are able to shift the cytosolic AGPase reaction from equilibrium. In this regard, PPi-dependent fructose 6-phosphate 1-phosphotransferase (E.C. 2.7.1.90) and UDP-glucose pyrophosphorylase (E.C. 2.7.7.9) have been proposed to be important cytosolic PPi-consuming reactions enabling the provision of cytosolic ADP-glucose for plastidial starch biosynthesis (ap Rees and Morrell, Reference ap Rees and Morrell1990; Kleczkowski, Reference Kleczkowski1994). In the developing endosperms of wheat, maize, barley and rice, the cytosolic isoform accounts for 65–95% of the total AGPase activity. Consequently, most of the storage starch biosynthesis in these tissues occurs through import of ADP-glucose into amyloplasts via a specialized nucleotide sugar transporter which imports ADP-glucose in exchange for ADP, the by-product of the plastidial starch synthase reaction (Fig. 2B) (Möhlmann et al., Reference Möhlmann, Tjaden, Henrichs, Quick, Hausler and Neuhaus1997; Tetlow et al., 2003a; Bowsher et al., Reference Bowsher, Scrase-Field, Esposito, Emes and Tetlow2007). The amino acid sequence of the maize endosperm ADP-glucose transporter, termed Bt1 has been determined (Kirchberger et al., Reference Kirchberger, Leroch, Huynen, Wahl, Neuhaus and Tjaden2007). Storage starch biosynthesis in non-graminaceous plant tissues and algae appears to be solely dependent on a plastidial AGPase and import of hexose phosphates and ATP from the cytosol (Fig. 2A) (see review by Comparot-Moss and Denyer, Reference Comparot-Moss and Denyer2009).
Plants possess multiple genes encoding the AGP-L or AGP-S subunits and these are differentially expressed in different plant organs. This means that the AGPase subunit composition may vary in different parts of the same plant in species such as rice (Nakamura and Kawaguchi, Reference Nakamura and Kawaguchi1992), barley (Villand et al., Reference Villand, Aalen, Olsen, Lonneborg, Lüthi and Kleczkowski1992a) and potato (La Cognata et al., Reference La Cognata, Willmitzer and Müller-Röber1995). The multiple genes encoding the AGP-L subunits show strong specificity in their expression, for example, being restricted to leaf, or root and endosperm in barley (Villand et al., Reference Villand, Aalen, Olsen, Lonneborg, Lüthi and Kleczkowski1992a, Reference Villand, Olsen, Killan and Kleczkowskib), wheat (Olive et al., Reference Olive, Ellis and Schuch1989) and rice (Lee et al., Reference Lee, Hwang, Han, Eom, Kang, Han, Choi, Cho, Bhoo, An, Hahn, Okita and Jeon2007), or induced under specific conditions, such as increased sucrose or glucose levels in potato (Müller-Rober et al., Reference Müller-Rober, Kossmann, Hannah, Willmitzer and Sonnewald1990; Duwenig et al., Reference Duwenig, Steup and Kossmann1997) and Arabidopsis (Crevillén et al., Reference Crevillén, Ballicora, Mérida, Preiss and Romero2003, Reference Crevillén, Ventriglia, Pinto, Orea, Mérida and Romero2005). Multiple isoforms of the AGP-S subunit in bean show organ-specific expression patterns; one form is expressed only in leaves, the other in both leaves and cotyledons (Weber et al., Reference Weber, Heim, Borisjuk and Wobus1995). Different mRNAs encoding the AGP-S subunit in maize also have distinct tissue expression patterns (Giroux and Hannah, Reference Giroux and Hannah1994; Prioul et al., Reference Prioul, Jeanette, Reyss, Grégory, Giroux, Hannah and Causse1994). The differential expression of subunits in different tissues may produce AGPases with varying degrees of sensitivity to allosteric effectors (see below), which are suited to the particular metabolic demands of a given tissue/organ. In developing cereal endosperms, the subcellular localization of some AGPase isoforms is thought to be determined by differential splicing of AGPase genes. Studies with barley indicate that the plastidial and cytosolic AGP-S subunit mRNAs are produced from a single gene by the use of two alternative first exons (Thorbjørnsen et al., Reference Thorbjørnsen, Villand, Kleczkowski and Olsen1996b).
The catalytic activities of AGPases are, to varying degrees, subject to allosteric control. In higher plants AGPases are generally stimulated by 3-phosphoglyceric acid (3-PGA) and inhibited by inorganic orthophosphate (Pi) (Ghosh and Preiss, Reference Ghosh and Preiss1966). By contrast, the homotetrameric bacterial AGPase is stimulated by fructose 1,6-bisphosphate and inhibited by AMP (Haugen and Preiss, Reference Haugen and Preiss1979). The relative sensitivity of plant AGPases to these allosteric effectors appears to depend on the tissue and plastid type, and, in the case of cereal endosperms, the subcellular location of the enzyme. A small polymorphic motif within the small subunit of AGPase may influence both catalytic and allosteric properties by modulating subunit interactions (Cross et al., Reference Cross, Clancy, Shaw, Boehlein, Greene, Schmidt, Okita and Hannah2005). The chloroplast AGPase, which synthesizes ADP-glucose from the glucose 1-phosphate (Glc 1-P) produced from photosynthesis, is tightly regulated by metabolite concentrations, being activated by micromolar amounts of 3-PGA and inhibited by Pi (Ghosh and Preiss, Reference Ghosh and Preiss1966). The ratio of these two allosteric effectors is thought to play a key role in the control of starch synthesis in photosynthetic tissues (Preiss, Reference Preiss and Miflin1991). However, evidence from wheat (Gómez-Casati and Iglesias, Reference Gómez-Casati and Iglesias2002; Tetlow et al., Reference Tetlow, Davies, Vardy, Bowsher, Burrell and Emes2003b) and barley (Kleczkowski et al., Reference Kleczkowski, Villand, Lüthi, Olsen and Preiss1993) endosperms suggests that measurable activity in monocot storage tissues (the majority of which is cytosolic, see above) is much less sensitive to 3-PGA activation and Pi-inhibition than other forms of AGPase. However, the plastidial AGPase from the storage tissues of dicots (e.g. potato tuber) appears to be as sensitive to the allosteric effectors as its counterparts in the chloroplast (Hylton and Smith, Reference Hylton and Smith1992; Ballicora et al., Reference Ballicora, Laughlin, Fu, Okita, Barry and Preiss1995). Thus in tissues committed to the long-term storage of starch, such as developing seeds, there is reduced sensitivity of AGPase to allosteric effectors. The sensitivity of plastidial AGPase to allosteric regulation in other plastid types, such as leucoplasts and chromoplasts, is unknown.
Post-translational modification of AGPase involving thioredoxin was proposed by Fu et al. (Reference Fu, Ballicora, Leykam and Preiss1998) following the observation of partial inactivation of the recombinant potato enzyme by the formation of intramolecular disulphide bonds between the N-termini of the AGP-S subunits. Redox control of AGPase through sucrose supply in potato tubers has recently been proposed, whereby reductive activation causes channelling of carbon to starch and away from respiratory/glycolytic metabolism (Tiessen et al., Reference Tiessen, Hendriks, Stitt, Branscheid, Gibon, Farré and Geigenberger2002), and is probably a general mechanism operating in other storage tissues, such as developing seeds. The signalling components leading to redox modulation of AGPase are beginning to be understood, and are thought to involve sucrose and glucose acting via an SNF1-related protein kinase (SNF1 is sucrose non-fermenting-1 protein kinase, originally described in Saccharomyces cerevisiae) and hexokinase (E.C. 2.7.1.1), respectively (see Tiessen et al., Reference Tiessen, Prescha, Branscheid, Palacios, McKibbin, Halford and Geigenberger2003). Studies with transgenic Arabidopsis indicate a role for trehalose 6-phosphate in promoting the SNF1-related protein kinase-dependent redox activation of AGPase (Kolbe et al., Reference Kolbe, Tiessen, Schluepmann, Paul, Ulrich and Geigenberger2005). Sugar-induced redox activation of AGPase is lost in transgenic plants expressing a trehalose 6-phosphate phosphatase (E.C. 3.1.3.12), which causes a reduction in trehalose 6-phosphate content (Kolbe et al., Reference Kolbe, Tiessen, Schluepmann, Paul, Ulrich and Geigenberger2005). Changes in allosteric effectors (3-PGA and Pi) and light-dependent changes in redox activation of AGPase are likely to be more important factors in controlling rates of transient starch synthesis over a diel cycle than for storage starch synthesis in developing seeds.
Much research attention has been focused on modulating the activity of AGPase in crop plants in order to increase starch yield. Plant productivity is regulated by both the rate and duration of carbon fixation in ‘source’ leaves, and the capacity of developing ‘sink’ tissues and organs to assimilate fixed carbon (Woodrow and Berry, Reference Woodrow and Berry1988). Under environmental conditions when photosynthesis is not limiting, overall productivity appears to be regulated by sink strength (i.e. the efficiency of utilization of photosynthates for metabolism and biomass/yield production). Productivity can be enhanced if plants possess a greater capacity to store fixed carbon due to increased sink strength. This strategy has been successfully demonstrated in potato tubers (Stark et al., Reference Stark, Timmerman, Barry, Preiss and Kishore1992) and in maize endosperm (Giroux et al., Reference Giroux, Shaw, Barry, Cobb, Greene, Okita and Hannah1996) using allosterically unregulated forms of AGPase. In rice, yields are limited by sink strength, in particular the capacity of developing seeds to take up and convert photosynthates (sucrose) into starch (Rowland-Bamford et al., Reference Rowland-Bamford, Allen, Baker and Boote1990; Chen and Sung, Reference Chen and Sung1994). The sink strength of developing rice seeds is increased by the expression of an allosterically insensitive bacterial mutant AGPase (the Escherichia coli glgC mutant) in the cytosol, resulting in increased starch production (Sakulsingharoj et al., Reference Sakulsingharoj, Choi, Hwang, Edwards, Bork, Meyer, Preiss and Okita2004). Overall, these studies show that modulation of AGPase activity in sink tissues is a promising target for increasing the carbon flow into starch in a number of important crop species. However, the flux control coefficient of AGPase is low in some tissues (as low as 0.08 in developing bean seeds) (Weber et al., Reference Weber, Rolletschek, Heim, Golombek, Gubatz and Wobus2000; Rolletschek et al., Reference Rolletschek, Hajirezaei, Wobus and Weber2002), and in insertional mutant pea plants deficient in AGPase limited starch synthesis still occurs (Weigelt et al., Reference Weigelt, Küster, Rutten, Fait, Ffernie, Miersch, Wasternack, Emery, Desel, Hosein, Müller, Saalbach and Weber2009). It has recently been suggested that alternative routes of starch biosynthesis are available using hexose phosphates via a starch phosphorylase (SP)-mediated pathway (Fettke et al., Reference Fettke, Albrecht, Hejazi, Mahlow, Nakamura and Steup2010).
Glucan chain formation by starch synthases
Higher plant starch synthases (SS, E.C. 2.4.1.21) catalyse the transfer of the glucosyl moiety of the soluble precursor ADP-glucose to the non-reducing end of a pre-existing α-(1 → 4)-O-linked glucan primer to synthesize glucan polymers, which eventually, in conjunction with other enzymes (see below), form the insoluble polymers amylose and amylopectin. Plants possess multiple isoforms of SSs, containing at least five isoforms that are categorized according to conserved sequence relationships. Isoforms within each of the major classes of SS genes are highly conserved in higher plants (see Ball and Morell, Reference Ball and Morell2003). In the SSs of land plants and green algae the conserved region is localized to a ‘core’ region in the C-terminus of approximately 60 kDa, but in prokaryotic glycogen synthases (GS, E.C. 2.4.1.11) these residues/domains are distributed across the protein sequence. The highly conserved K–X–G–G–L motif responsible for substrate binding in prokaryotic GSs and higher plant SSs (Furukawa et al., Reference Furukawa, Tagaya, Inouye, Preiss and Fukui1990, Reference Furukawa, Tagaya, Tanizawa and Fukui1993; Busi et al., Reference Busi, Palapoli, Valdez, Fornasari, Wayllace, Gomez-Casati, Parisi and Ugalde2008) is found within the C-terminus in higher plant SSs (Nichols et al., Reference Nichols, Keeling, Spalding and Guan2000). Detailed biochemical analysis of maize SSs indicates the importance of glutamate and aspartate residues for catalytic activity and substrate binding (Nichols et al., Reference Nichols, Keeling, Spalding and Guan2000), and the involvement of lysine in the K–X–G–G–L domain in determining glucan primer preference (Gao et al., Reference Gao, Keeling, Shibles and Guan2004). Variation amongst the SS isoforms occurs within the N-terminus upstream of the catalytic core, which can vary greatly in length, from 2.2 kDa in granule-bound starch synthase I (GBSSI) to approximately 135 kDa in maize SSIII (Gao et al., Reference Gao, Wanat, Stinard, James and Myers1998) (Fig. 3). Research on the wheat endosperm suggests that soluble starch synthases have a high flux control coefficient, indicating their importance in carbon allocation to starch in this tissue (Jenner et al., Reference Jenner, Siwek and Hawker1993; Keeling et al., Reference Keeling, Bacon and Holt1993; Tomlinson and Denyer, Reference Tomlinson and Denyer2003). The major classes of SS genes can be broadly split into two groups, the first primarily involved in amylose synthesis, and the second principally confined to amylopectin biosynthesis.

Figure 3 Domain comparison of cereal SS sequences showing C-terminal catalytic domains (including the ADP-glucose binding domain) in black (not drawn to scale). The five known SS isoforms are shown with the name of the corresponding maize mutant in parentheses. The different SSs have N-terminal domains of varying lengths (shown as hatched bars); SSIII in particular has a unique N-terminal extension thought to be involved in controlling protein–protein interactions. Numbers of amino acids constituting each isoform in maize are shown.
Amylose biosynthesis
One group of SS genes encodes the granule-bound starch synthases (GBSS), and includes GBSSI and GBSSII. GBSSI is encoded by the Waxy locus in cereals, functioning specifically to elongate amylose (De Fekete et al., Reference De Fekete, Leloir and Cardini1960; Nelson and Rines, Reference Nelson and Rines1962) and is completely within the granule matrix (one of the so-called granule-associated proteins). Mutations in the Waxy locus leading to loss of GBSS activity result in amylose-free (waxy) starches, and in maize there is a clear effect of Waxy gene dosage on the production of GBSSI activity and resulting amylose content of the storage starch (Tsai, Reference Tsai1974; Shure et al., Reference Shure, Wessler and Fedoroff1983). In addition to its role in amylose biosynthesis, GBSSI is thought to be responsible for extension of long glucans (termed extra-long chains) of amylopectin in both in vitro and in vivo experiments in sweet potato (Baba et al., Reference Baba, Yoshii and Kainuma1987), Chlamydomonas reinhardtii (Delrue et al., Reference Delrue, Fontaine, Routier, Decq, Wieruszeski and Ball1992; Maddelein et al., Reference Maddelein, Libessart, Bellanger, Delrue, D'Hulst and Ball1994; van de Wal et al., Reference Van de Wal, D'Hulst, Vincken, Buléon, Visser and Ball1998), pea and potato (Denyer et al., Reference Denyer, Clarke, Hylton, Tatge and Smith1996a) and rice endosperm (Hanashiro et al., Reference Hanashiro, Itoh, Kuratomi, Yamazaki, Igarishi, Matsugasako and Takeda2008). Further support for this hypothesis is the observed reduction in extra-long chains in amylopectin in Waxy rice (Hizukuri et al., Reference Hizukuri, Takeda, Maruta and Juliano1989). Experiments with potato have shown that, in certain genetic backgrounds, GBSS can have a profound influence on the granule structure and morphology of tubers (Fulton et al., Reference Fulton, Edwards, Pilling, Robinson, Fahy, Seale, Kato, Donald, Geigenberger, Martin and Smith2002). Expression of GBSSI appears to be mostly confined to storage tissues, and a second form of GBSS (GBSSII), which is encoded by a separate gene, is thought to be responsible for amylose synthesis in leaves and other non-storage tissues that accumulate transient starch (Fujita and Taira, Reference Fujita and Taira1998; Nakamura et al., Reference Nakamura, Vrinten, Hayakawa and Ikeda1998; Vrinten and Nakamura, Reference Vrinten and Nakamura2000).
A unique property of GBSSI is the stimulation of its catalytic activity by MOS when synthesizing amylose (Denyer et al., Reference Denyer, Clarke, Hylton, Tatge and Smith1996a, Reference Denyer, Waite, Edwards, Martin and Smith1999). One hypothesis is that MOS diffuse into the granule matrix, where GBSSI synthesizes amylose by elongating the MOS primers (for a review of amylose biosynthesis, see Denyer et al., Reference Denyer, Johnson, Zeeman and Smith2001). In vitro experiments have shown that glucan chain extension from amylopectin to yield amylose is possible with extended incubation times, suggesting that there are two different mechanisms for the synthesis of amylose (van de Wal et al., Reference Van de Wal, D'Hulst, Vincken, Buléon, Visser and Ball1998). The properties of Waxy starches demonstrate that amylose synthesis is not required for the formation of semi-crystalline granules, and its synthesis probably takes place within a pre-existing amylopectin matrix as the granule is formed (Dauvillée et al., Reference Dauvillée, Colleoni, Shaw, Mouille, D'Hulst, Morell, Samuel, Bouchet, Gallant, Sinsky and Ball1999; Denyer et al., 1999) and the absence of amylose has no effect on the crystallinity of starch granules (Cooke and Gidley, Reference Cooke and Gidley1992; Bogracheva et al., Reference Bogracheva, Cairns, Noel, Hulleman, Wang, Morris, Ring and Gidley1999). There is also evidence that the amylose content of starch in storage tissues is affected by substrate (ADP-glucose) availability. GBSSI has a lower affinity for ADP-glucose than the soluble starch synthases involved in amylopectin synthesis [Km of 1.4 mM for pea (Clarke et al., Reference Clarke, Denyer, Jenner and Smith1999) is five- to ten-fold greater than other soluble isoforms of starch synthases], and estimates of the ADP-glucose concentration in plastids (approximately 0.9 mM), mean that the GBSSI reaction is not saturated and fluctuations in ADP-glucose concentration can reduce amylose synthesis more than amylopectin synthesis (Clarke et al., Reference Clarke, Denyer, Jenner and Smith1999). This idea is supported by studies with plant mutants predicted to modify ADP-glucose content. The rb and rug mutants of pea (Clarke et al., Reference Clarke, Denyer, Jenner and Smith1999), STA1 and STA5 mutants of Chlamydomonas (Van den Koornhuyse et al., Reference Van den Koornhuyse, Libessart, Delrue, Zabawinski, Decq, Iglesias, Carlton, Preiss and Ball1996), and potato expressing antisense AGPase constructs (Lloyd et al., Reference Lloyd, Landschütze and Kossmann1999a) are expected to have lowered amounts of ADP-glucose, and all contain less amylose in storage starch.
Amylopectin biosynthesis
A second group of SS genes (designated SSI, SSII, SSIII, and SSIV) are exclusively involved in amylopectin biosynthesis and individual SS isoforms from this group probably play unique roles. The distribution of the SSs within the plastid between the stroma and starch granules varies between species, tissue and developmental stage (Ball and Morell, Reference Ball and Morell2003). The study of SS mutants in a number of plant species has been helpful in the assignment of in vivo functions/roles for the soluble and granule-associated SS isoforms. Valuable information about their roles in amylopectin synthesis in vivo is being derived from mutants lacking specific isoforms, and is summarized below. However, in some cases there may be pleiotropic effects of mutations on more than one starch synthesis enzyme.
SSI
SSI is primarily responsible for the synthesis of the shortest glucan chains, i.e. those with a degree of polymerization (DP) of 10 glucosyl units or less (Guan and Keeling, Reference Guan and Keeling1998; Commuri and Keeling, Reference Commuri and Keeling2001), and extension of these glucan chains is achieved by the activities of SSII and SSIII isoforms, each of which acts on progressively longer glucan chains. SSI (and SSII) shows stimulated catalytic activity with glycogen in the presence of 0.5 M citrate (Imparl-Radosevich et al., Reference Imparl-Radosevich, Li, Zhang, McKean, Keeling and Guan1998, Reference Imparl-Radosevich, Nichols, Li, McKean, Keeling and Guan1999). Analyses of mutants and transgenic plants lacking SSI activity tend to support the notion that SSI elongates short (DP 4–7) glucan chains. Studies of mutants of Arabidopsis (Delvallé et al., Reference Delvallé, Dumez, Wattebled, Roldán, Planchot, Berbezy, Colonna, Vyas, Chatterjee, Ball, Mérida and D'Hulst2005) and rice (Fujita et al., Reference Fujita, Yoshida, Asakura, Ohdan, Miyao, Hirochika and Nakamura2006) lacking SSI show deficiencies in shorter (DP 6–12) glucan chain lengths of the starches, supporting its proposed role in the synthesis of short glucan chains. The fact that loss of SSI, a major SS isoform in cereal endosperms, has no effect on the size and shape of developing seeds and starch granules, nor on the crystallinity of endosperm starch in rice (Fujita et al., Reference Fujita, Yoshida, Asakura, Ohdan, Miyao, Hirochika and Nakamura2006), suggests that other SS forms are capable of partly compensating for its function. In cereals SSI is expressed early in development (5–10 DAP in wheat endosperm), and considerable amounts of the protein become entrapped as ‘granule-associated’ proteins (see later) (Peng et al., Reference Peng, Hucl and Chibbar2001).
SSII
Two classes of SSII genes are present in monocots: SSIIa and SSIIb. The role of the latter in starch biosynthesis is unknown as no mutants have been identified. In vitro studies of the two SSII forms from maize reveal different substrate specificities and kinetic properties (Imparl-Radosevich et al., Reference Imparl-Radosevich, Nichols, Li, McKean, Keeling and Guan1999, Reference Imparl-Radosevich, Gameon, McKean, Wetterberg, Keeling and Guan2003). SSIIa predominates in cereal endosperms, while SSIIb is mostly confined to photosynthetic tissues. Both SSI and SSII are also localized within the starch granules in many cereals (Mu-Forster et al., Reference Mu-Forster, Huang, Powers, Harriman, Knight, Singletary, Keeling and Wasserman1996; Morell et al., Reference Morell, Kosar-Hashemi, Cmiel, Samuel, Chandler, Rahman, Buléon, Batey and Li2003; Umemoto and Aoki, Reference Umemoto and Aoki2005). Loss of SSIIa from barley and wheat seeds results in their reduced starch content, reduced amylopectin chain-length distribution, altered granule morphology and reduced crystallinity (Morell et al., Reference Morell, Kosar-Hashemi, Cmiel, Samuel, Chandler, Rahman, Buléon, Batey and Li2003; Kosar-Hashemi et al., Reference Kosar-Hashemi, Li, Larroque, Regina, Yamamori, Morell and Rahman2007). In maize endosperm, SSIIa is the product of the sugary2 gene, a mutation resulting in more short chains of DP 6–10, fewer chains of DP 12–30 and an elevated amylose content in the endosperm of up to 40% (Zhang et al., Reference Zhang, Colleoni, Ratushna, Sirghie-Colleoni, James and Myers2004). In monocots and green algae, SSIIa appears to play a specific role in the synthesis of the intermediate-size glucan chains of DP 12–24 by elongating short chains of DP ≤ 10 (Fontaine et al., Reference Fontaine, D'Hulst, Maddelein, Routier, Pépin, Decq, Wieruszeski, Delrue, Van den Koornhuyse, Bossu, Fournet and Ball1993; Imparl-Radosevich et al., Reference Imparl-Radosevich, Gameon, McKean, Wetterberg, Keeling and Guan2003; Morell et al., Reference Morell, Kosar-Hashemi, Cmiel, Samuel, Chandler, Rahman, Buléon, Batey and Li2003). Despite SSIIa being a minor contributor to the total measurable SS activities in cereal endosperms, its loss/down-regulation has a major impact on both the amount and composition of starch. In cereals, important food-processing properties, such as starch gelatinization temperature, are controlled by the gene for SSII (Bao et al., Reference Bao, Xiao, Hiratsuka, Sun and Umemoto2009). The simultaneous down-regulation of multiple SS reactions, such as in potato following reduction of SSII and SSIII activities, causes extensive alterations in starch structure (Edwards et al., Reference Edwards, Fulton, Hylton, Jobling, Gidley, Rössner, Martin and Smith1999; Lloyd et al., Reference Lloyd, Springer, Buléon, Müller-Rober, Willmitzer and Kossmann1999b). In Arabidopsis loss of SSII results in an increase in the amylose:amylopectin ratio, an increase in total amylose and a reduction in amylopectin glucan chains of DP 12–28, whereas a double mutant deficient in SSII and SSIII causes a severe phenotype with reduced growth rate and lowered starch content (Zhang et al., Reference Zhang, Szydlowski, Delvallé, D'Hulst, James and Myers2008). The synergistic effects of the loss of SSII and SSIII are more severe than those with each individual mutation, suggesting only partial redundancy with respect to the functions of these SSs in amylopectin biosynthesis.
SSIII
After SSI, SSIII catalyses the second most abundant measurable activity in cereal endosperms such as maize and rice (Cao et al., Reference Cao, Imparl-Radosevich, Guan, Keeling, James and Myers1999; Fujita et al., Reference Fujita, Yoshida, Asakura, Ohdan, Miyao, Hirochika and Nakamura2006). Two genes are responsible for expression of SSIII in the endosperm (SSSIIIa) and leaf (SSIIIb) of rice (Hirose and Terao, Reference Hirose and Terao2004; Dian et al., Reference Dian, Jiang and Wu2005). Studies with plants lacking SSIII suggest that the primary role of this enzyme is amylopectin synthesis, although the impact of loss of SSIII appears to differ with the genetic background. Mutations in maize eliminating SSIII (du1) produce an endosperm with a glassy, dull appearance (Mangelsdorf, Reference Mangelsdorf1947), a phenotype which is only conspicuous in Waxy backgrounds (Gao et al., Reference Gao, Wanat, Stinard, James and Myers1998). Analysis of amylopectin from du1 maize and rice show altered granule morphology and crystallinity and a reduction in long glucan chains (DP ≥ 30), suggesting a role for SSIII in their elongation (Inouchi et al., Reference Inouchi, Glover, Takaya and Fuwa1983; Fujita et al., Reference Fujita, Yoshida, Kondo, Saito, Utsumi, Tokunaga, Nishi, Satoh, Park, Jane, Miyao, Hirochika and Nakamura2007; Ryoo et al., Reference Ryoo, Yu, Park, Baik, Park, Cho, Bhoo, An, Hahn and Jeon2007). Partially purified SSIII from maize endosperm has a higher affinity for amylose (longer glucan chains) compared with amylopectin and glycogen (Cao et al., Reference Cao, James and Myers2000). It has been proposed, therefore, that SSIII functions in the provision of long chains which extend between clusters of amylopectin (James et al., Reference James, Denyer and Myers2003). In addition to alterations in starch structure and physical properties, loss of SSIII from maize endosperm is also associated with pleiotropic effects on other starch synthases, causing increased activities of SS (Cao et al., Reference Cao, Imparl-Radosevich, Guan, Keeling, James and Myers1999), and a reduction in starch branching enzyme (SBE) IIa activity (Boyer and Preiss, Reference Boyer and Preiss1981). In rice endosperm increased transcripts of both SSI and GBSSI in SSIIIa-deficient plants are proposed to contribute to the observed starch phenotype (Fujita et al., Reference Fujita, Yoshida, Kondo, Saito, Utsumi, Tokunaga, Nishi, Satoh, Park, Jane, Miyao, Hirochika and Nakamura2007), and in Chlamydomonas loss of SSIII from the sta3 mutant is compensated by GBSSI (Ral et al., Reference Ral, Colleoni, Wattebled, Dauvillée, Nempont, Deschamps, Li, Morell, Chibbar, Purton, d'Hulst and Ball2006). These observations have led to the proposal that, in addition to its catalytic role, SSIII possesses regulatory properties with respect to control over the starch biosynthetic pathway. SSIII mutants of Arabidopsis show a starch excess phenotype in the leaves caused by an increased rate of starch biosynthesis (Zhang et al., Reference Zhang, Myers and James2005), suggesting that this enzyme acts as a negative regulator of transient starch biosynthesis. Although the mechanism by which SSIII influences the rate of starch synthesis and/or other biosynthetic enzymes in the pathway is not clear, analysis of the structure suggests potential regulatory properties which may also control the pathway in developing seeds. SSIII is the largest of the SS isoforms [rice SSIII is a 230 kDa polypeptide (Dian et al., Reference Dian, Jiang and Wu2005)] and has a long extension of amino acids at the N-terminus, termed the SSIII homology domain (SSIIIHD), which contains a putative 14-3-3 protein binding domain within the C-terminal (catalytic) domain. The SSIIIHD is involved in protein–protein interactions with other starch biosynthetic enzymes (see below) and glucan binding (Hennen-Bierwagen et al., Reference Hennen-Bierwagen, Liu, Marsh, Kim, Gan, Tetlow, Emes, James and Myers2008, Reference Hennen-Bierwagen, Lin, Grimaud, Planchot, Keeling, James and Myers2009; Valdez et al., Reference Valdez, Busi, Wayllace, Parisi, Ugalde and Gomez-Casati2008; Wayllace et al., Reference Wayllace, Valdez, Ugalde, Busi and Gomez-Casati2010).
SSIV
SSIV is the most recently discovered form of higher plant SSs (Dian et al., Reference Dian, Jiang and Wu2005), and has structural characteristics conserved amongst other SS forms, including two ADP-glucose binding domains (a K–X–G–G–L motif) and a unique (SSIV-specific) N-terminus, which includes two coiled-coil domains and a putative 14-3-3 protein binding domain (Leterrier et al., Reference Leterrier, Holappa, Broglie and Beckles2008). SSIV is phylogenetically related to SSIII, and two isoforms exist in plants, SSIVa and SSIVb, which are differentially expressed in endosperm and leaf tissues, respectively (Leterrier et al., Reference Leterrier, Holappa, Broglie and Beckles2008). Structural features of the ADP-glucose binding domains of SSIII and SSIV suggest they have different glucan specificities. The catalytic activity of SSIV is dependent on ADP-glucose (unlike other SSs, SSIV shows no activity with UDP-glucose) and the Arabidopsis enzyme has affinity for ADP-glucose in the same range as other SS forms (Km for ADP-glucose of 0.47 mM). In vitro studies with SSIV indicate that it only elongates glucan chains in the presence of pre-existing glucan, and is particularly active with malto-triose (shows >90% of activity with amylopectin) (Szydlowski et al., Reference Szydlowski, Ragel, Raynaud, Lucas, Roldán, Montero, Munoz, Ovecka, Bahaji, Planchot, Pozueta-Romero, D'Hulst and Mérida2009). Reverse genetics studies indicate a role for SSIV in controlling the number of starch granules within a plastid. Loss of SSIV from Arabidopsis causes chloroplasts to accumulate a single, large starch granule, without observable effects on starch composition (Roldán et al., Reference Roldán, Lucas, Delvallé, Planchot, Jimenez, Perez, Ball, D'Hulst and Mérida2007). Studies with a SSIV/SSII double mutant in Arabidopsis indicates that loss of both SSs is required to prevent granule initiation, leading to the proposal that both of these phylogenetically related SSs regulate starch granule initiation (Szydlowski et al., Reference Szydlowski, Ragel, Raynaud, Lucas, Roldán, Montero, Munoz, Ovecka, Bahaji, Planchot, Pozueta-Romero, D'Hulst and Mérida2009). It has not been determined whether SSIV in storage (seed) tissues functions in an analogous manner to the leaf enzyme.
Branching of the glucan chain
Starch branching enzymes (SBEs, E.C. 2.4.1.18) generate α-(1 → 6)-linkages by cleaving internal α-(1 → 4) bonds and transferring the released reducing ends to C6 hydroxyls to form the branched structure of the amylopectin molecule. SBEs are related to the α-amylase super-family of enzymes (Jespersen et al., Reference Jespersen, MacGregor, Henrissat, Sierks and Svensson1993) and are able to generate α-(1 → 6)-linkages on linear and branched glucan substrates. Following cleavage of a α-(1 → 4)-linkage, SBEs can transfer the cleaved glucan to an acceptor chain which is either part of the original glucan chain, or part of an adjacent glucan chain (known as inter-chain transfer). Studies with potato SBE (originally termed Q-enzyme) suggest inter-chain transfer predominates, and that close association of glucan chains, for example, in a double helical configuration, create a more favourable environment for SBE catalytic activity (Borovsky et al., Reference Borovsky, Smith, Whelan, French and Kikumoto1979). As with the elongation of glucan chains by SSs, SBE activity is also a function of multiple isoforms, some of which are tissue- and/or developmental-specific in their expression patterns (Yamanouchi and Nakamura, Reference Yamanouchi and Nakamura1992; Gao et al., Reference Gao, Fisher, Kim, Shannon and Guiltinan1997; Sun et al., Reference Sun, Sathish, Ahlandsberg and Jansson1998; Regina et al., Reference Regina, Kosar-Hashemi, Li, Pedler, Mukai, Yamamoto, Gale, Sharp, Morell and Rahman2005). Analysis of the primary amino acid sequences of higher plant SBEs reveals two major classes; SBEI (also known as SBE B) and SBEII (SBE A). These differ in terms of the length of the glucan chain transferred in vitro and their substrate specificities; SBEII proteins transfer shorter chains and show a higher affinity towards amylopectin than their SBEI counterparts, which show higher rates of branching with amylose (Guan and Preiss, Reference Guan and Preiss1993; Takeda et al., Reference Takeda, Guan and Preiss1993; Rydberg et al., Reference Rydberg, Andersson, Andersson, Åman and Larsson2001). Phylogenetic analysis indicates that SBEI evolved prior to the monocot–dicot divergence, and its retention in higher plants suggests that it plays an important role in starch biosynthesis/metabolism (Gao et al., Reference Gao, Fisher, Kim, Shannon and Guiltinan1996). It is not known whether SBEI branches amylose in vivo. The construction of chimeric forms of maize SBEI and SBEII and analysis of their catalytic properties by Kuriki et al. (Reference Kuriki, Stewart and Preiss1997) indicate that the N- and C-termini of these proteins play important roles in determining substrate preference, catalytic capacity and chain length transfer. SBEs and SSs utilize the same substrate, so it is perhaps unsurprising that structural similarities exist between the active sites of these different classes of enzymes; aspartate and glutamate residues are important for the catalytic function of SBEs and α-amylase (E.C. 3.2.1.1) (Boel et al., Reference Boel, Brady, Brzozowski, Derewenda, Dodson, Jensen, Petersen, Swift, Thim and Woldike1990; Kuriki et al., Reference Kuriki, Guan, Sivak and Preiss1996) as with SSs (above). Studies with wheat endosperm indicate that the catalytic activity of SBEII forms is regulated by protein phosphorylation (Tetlow et al., Reference Tetlow, Wait, Lu, Akkasaeng, Bowsher, Esposito, Kosar-Hashemi, Morell and Emes2004). In monocots the SBEII class is made up of two closely related but discrete gene products, SBEIIa and SBEIIb (Rahman et al., Reference Rahman, Regina, Li, Mukai, Yamamoto, Kosar-Hashemi, Abrahams and Morell2001). In developing wheat endosperm there are marked differences in the expression of these enzymes, with the SBEIIb form being at much lower levels than the IIa (Morell et al., 1997; Regina et al., Reference Regina, Kosar-Hashemi, Li, Pedler, Mukai, Yamamoto, Gale, Sharp, Morell and Rahman2005). This is in contrast to the maize endosperm, where SBEIIb is the predominant form, being expressed at approximately 50 times the level of the IIa form (Gao et al., Reference Gao, Fisher, Kim, Shannon and Guiltinan1997); it is the most abundant protein in the maize endosperm amylopast stroma (Mu et al., Reference Mu, Yu, Wasserman and Carman2001).
Mutations in SBEII isoforms give the clearest phenotypes. In maize and rice, mutation of the gene encoding SBEIIb [also known as ‘amylose extender’ (ae − )] produces a high-amylose starch phenotype characterized by longer internal chain lengths of amylopectin than in normal starches and less frequently branched outer chains (Nishi et al., Reference Nishi, Nakamura, Tanaka and Satoh2001; Klucinec and Thompson, Reference Klucinec and Thompson2002; Evans and Thompson, Reference Evans and Thompson2004). In wheat such starches are only produced by suppression of both genes encoding the SBEIIa and SBEIIb forms, resulting in starches containing >70% amylose (Regina et al., Reference Regina, Li, Bird, Topping, Bowden, Freeman, Barsby, Kosar-Hashemi, Li, Rahman and Morell2006). In potato, down-regulation of the equivalent SBE form produces a high-amylose starch (Schwall et al., Reference Schwall, Safford, Westcott, Jeffcoat, Tayal, Shi, Gidley and Jobling2000). Down-regulation or elimination of SBEI activity in both monocots and dicots appears to have minimal effects on starch synthesis and composition in photosynthetic and non-photosynthetic tissues (Flipse et al., Reference Flipse, Suurs, Keetels, Kossmann, Jacobsen and Visser1996; Blauth et al., Reference Blauth, Kim, Klucinec, Shannon, Thompson and Guiltinan2002; Satoh et al., Reference Satoh, Nishi, Yamashita, Takemoto, Tanaka, Hosaka, Sakurai, Fujita and Nakamura2003). In addition, loss of SBEI from a SBEIIb-deficient background causes increased branching, suggestive of a regulatory role for SBEI in influencing other SBEs (Yao et al., Reference Yao, Thompson and Guiltinan2004). Physical interactions between SBEI and SBEIIb occur in amyloplasts from wheat endosperm (Tetlow et al., Reference Tetlow, Wait, Lu, Akkasaeng, Bowsher, Esposito, Kosar-Hashemi, Morell and Emes2004).
The maize SBEIIa mutant shows a clear phenotype in leaf starch, but no apparent alterations in the storage starch in the endosperm (Blauth et al., Reference Blauth, Yao, Klucinec, Shannon, Thompson and Guiltinan2001). This suggests a primary role for SBEIIa in leaf (transient) starch synthesis, and either no critical role in amylopectin biosynthesis in the endosperm, or else one that can easily be compensated for by other SBEs. Arabidopsis has two SBE isoforms belonging to the SBEII class (Fisher et al., Reference Fisher, Gao, Kim, Boyer and Guiltinan1996), plus a third putative form unrelated to the SBEII class, and with no assigned function. Loss of both SBEII forms from Arabidopsis results in a failure to synthesize starch, and accumulation of maltose in the cytosol (Dumez et al., Reference Dumez, Wattebled, Dauvillée, Delvallé, Planchot, Ball and D'Hulst2006). SBEII isoforms are also partitioned between the plastid stroma and the starch granules (Mu-Forster et al., Reference Mu-Forster, Huang, Powers, Harriman, Knight, Singletary, Keeling and Wasserman1996). As with the granule-associated SSs, the factors/mechanisms involved in partitioning the SBE proteins to the starch granules remain undetermined. The ability of proteins to become granule-associated may be a function of the relative affinities of their active sites for the glucan polymer, as with some isoforms of SS (Commuri and Keeling, Reference Commuri and Keeling2001), although it has been suggested that alternative splicing of an SBEII form in Phaseolus vulgaris causes an alteration in the properties of the enzyme, and partitioning within the starch granule (Hamada et al., Reference Hamada, Ito, Hiraga, Inagaki, Nozaki, Isono, Yoshimoto, Takeda and Matsui2002).
In vitro analysis of heterologously expressed maize SBEs (Seo et al., Reference Seo, Kim, Scott, Singletary, Wong, James and Myers2002) has shed further light on the roles of different SBE isoforms in the construction of the starch granule. Expression of three functional maize SBE genes in a yeast strain lacking the endogenous yeast glucan branching enzyme showed that SBEI is unable to act in the absence of SBEIIa or SBEIIb, and that SBEII may act before SBEI on precursor polymers. These data suggest that SBEI does not play a central role in this in vitro system, leaving its role in the starch biosynthetic pathway still an open question.
The role of debranching enzymes in amylopectin synthesis
In addition to SSs and SBEs, isoamylases, also termed debranching enzymes (DBEs, E.C. 3.2.1.41 and E.C. 3.2.1.68, originally termed R-enzyme) may be important in the formation of crystalline amylopectin. Analysis of low-starch mutants that accumulate a disordered water-soluble polysaccharide, termed phytoglycogen, have been described in a wide range of higher plants, including Arabidopsis and maize, the unicellular alga Chlamydomonas (Morris and Morris, Reference Morris and Morris1939; James et al., Reference James, Robertson and Myers1995; Mouille et al., Reference Mouille, Maddelein, Libessart, Talaga, Decq, Delrue and Ball1996; Zeeman et al., Reference Zeeman, Umemoto, Lue, Au-Yeung, Martin, Smith and Chen1998) and recently in rice (Fujita et al., Reference Fujita, Toyosawa, Utsumi, Higuchi, Hanashiro, Ikegami, Akuzawa, Yoshida, Mori, Inomata, Itoh, Miyao, Hirochika, Satoh and Nakamura2009), indicating that starch synthesis involves debranching enzymes working in conjunction with the SSs and SBEs. Two groups of DBEs exist in plants: the isoamylase-type (of which at least three forms exist in angiosperms: isoamylase-1, isoamylase-2 and isoamylase-3) and the pullulanase-type (also known as limit-dextrinases) which efficiently hydrolyse (debranch) α-(1 → 6)-linkages in amylopectin and pullulan (a fungal polymer of malto-triose residues), respectively, and are part of the α-amylase ‘super-family’ of enzymes. The Arabidopsis genome contains three isoamylase-type DBEs and one pullulanase-type DBE; these enzymes are present in most starch-synthesizing organisms (Deschamps et al., Reference Deschamps, Haferkamp, d'Hulst, Neuhaus and Ball2008b). Both groups in higher plants share a common N-terminal domain, the function of which is yet to be elucidated. The decrease or loss of either isoamylase-1 or isoamylase-2 type DBE activities is thought to be responsible for the accumulation of phytoglycogen rather than starch in mutant/transgenic plants (Bustos et al., Reference Bustos, Fahy, Hylton, Seale, Nebane, Edwards, Martin and Smith2004) and algae (Mouille et al., Reference Mouille, Maddelein, Libessart, Talaga, Decq, Delrue and Ball1996), and, in rice, endosperm residual pullulanase-type DBE activity may modulate these phenotypic effects (Nakamura et al., Reference Nakamura, Vrinten, Hayakawa and Ikeda1998). Although isoamylase-2 lacks amino acid residues essential for catalytic activity, isoamylase-1 and isoamylase-2 polypeptides constitute a hetero-oligomeric complex to form a functional enzyme (Hussain et al., Reference Hussain, Mant, Seale, Zeeman, Hinchliffe, Edwards, Hylton, Bornemann, Smith, Martin and Bustos2003). In maize this complex is approximately 400 kDa, and is also found with a 300 kDa complex containing isoamylase-1, but not isoamylase-2. Studies with an isoamylase-1-null line show that isoamylase complexes are absent, indicating the importance of this isoform for complex assembly (Kubo et al., Reference Kubo, Colleoni, Dinges, Lin, Lappe, Rivenbark, Meyer, Ball, James, Hennen-Bierwagen and Myers2010). The idea of isoamylase catalytic activity being a result of a hetero-enzyme complex was given support by the observation that targeted mutants of Arabidopsis lacking one or both of isoamylase-1 and isoamylase-2 exhibit identical phenotypes (Delatte et al., Reference Delatte, Trevisan, Parker and Zeeman2005; Wattebled et al., Reference Wattebled, Dong, Dumez, Delvallé, Planchot, Berbezy, Vyas, Colonna, Chatterjee, Ball and D'Hulst2005). Complementation of sugary-1 mutants with isoamylase-1 results in restoration of amylopectin synthesis, suggestive of a role for this DBE isoform (Kubo et al., Reference Kubo, Rahman, Utsumi, Li, Makai, Yamamoto, Ugaki, Harada, Satoh, Konik-Rose, Morell and Nakamura2005). The isoamylase-1 and isoamylase-2 forms, therefore, are probably involved in developing amylopectin crystallinity by trimming inadequately spaced glucan branches, thus allowing tighter packing of glucan chains. A third isoform of isoamylase (isoamylase-3) appears to be compulsory for starch degradation at night in Arabidopsis leaves, and is catalytically more active on water-soluble polysaccharides that have been processed by β-amylase and starch phosphorylase (Wattebled et al., Reference Wattebled, Dong, Dumez, Delvallé, Planchot, Berbezy, Vyas, Colonna, Chatterjee, Ball and D'Hulst2005). Studies with barley mutants and transgenic rice suggest that isoamylases play a crucial role in starch granule initiation (Burton et al., Reference Burton, Jenner, Carrangis, Fahy, Fincher, Hylton, Laurie, Parker, Waite, van Wegen, Verhoeven and Denyer2002; Kawagoe et al., Reference Kawagoe, Kubo, Satoh, Takaiwa and Nakamura2005). In maize endosperm the pullulanase-type DBE activity is thought to have a bifunctional role, assisting in both starch synthesis and degradation (Dinges et al., Reference Dinges, Colleoni, James and Myers2003). Pullulanase tends to work on tightly branched glucans (including pullulan and amylopectin), and has weak affinity for loosely spaced glucan polymers such as glycogen, whereas the isoamylases are unable to hydrolyse pullulan, but can hydrolyse α-(1 → 6)-linkages in amylopectin and glycogen. In rice, reduction in pullulanase activity has no pleiotropic effects on other enzymes of starch synthesis; double mutants showing loss of pullulanase and isoamylase-1 show increased accumulation of phytoglycogen and water-soluble polysaccharides over those in the isoamylase-1 mutant alone, indicating partial overlap of function between pullulanase and isoamylase-1 (Fujita et al., Reference Fujita, Toyosawa, Utsumi, Higuchi, Hanashiro, Ikegami, Akuzawa, Yoshida, Mori, Inomata, Itoh, Miyao, Hirochika, Satoh and Nakamura2009).
The precise roles for the isoamylase-type and pullulanase-type DBEs in starch biosynthesis are not fully understood. Two models have been proposed which could define a role for the DBEs in starch synthesis and phytoglycogen accumulation. The ‘glucan-trimming’ (pre-amylopectin trimming) model proposes that glucan trimming is required for amylopectin aggregation into an insoluble granular structure (Ball et al., Reference Ball, Guan, James, Myers, Keeling, Mouille, Buléon, Colonna and Preiss1996; Myers et al., Reference Myers, Morell, James and Ball2000). DBE activity is responsible for the removal of inappropriately positioned branches (pre-amylopectin) generated at the surface of the growing starch granules, which would otherwise prevent crystallization. As such, the debranched structure would favour the formation of parallel double helices, leading to polysaccharide aggregation. The surface of immature starch granules contains numerous short chains, consistent with this model (Nielsen et al., Reference Nielsen, Baunsgaard and Blennow2002). An alternative to the glucan-trimming model proposes that DBEs function in starch synthesis indirectly in a ‘clearing’ role, removing soluble glucans from the stroma which are substrates for the amylopectin-synthesizing enzymes (SSs and SBEs), and thereby preventing the random/futile synthesis of glucan polymers by these enzymes which could cause accumulation of phytoglycogen (leading to a reduction in the rate of starch synthesis). This model could also explain the accumulation in phytoglycogen at the expense of amylopectin observed in DBE mutants in a number of species (Morris and Morris, Reference Morris and Morris1939; James et al., Reference James, Robertson and Myers1995; Mouille et al., Reference Mouille, Maddelein, Libessart, Talaga, Decq, Delrue and Ball1996; Zeeman et al., Reference Zeeman, Umemoto, Lue, Au-Yeung, Martin, Smith and Chen1998; Wattebled et al., Reference Wattebled, Dong, Dumez, Delvallé, Planchot, Berbezy, Vyas, Colonna, Chatterjee, Ball and D'Hulst2005, Reference Wattebled, Planchot, Dong, Szydlowski, Pontoire, Devin, Ball and D'Hulst2008; Fujita et al., Reference Fujita, Toyosawa, Utsumi, Higuchi, Hanashiro, Ikegami, Akuzawa, Yoshida, Mori, Inomata, Itoh, Miyao, Hirochika, Satoh and Nakamura2009).
Despite this experimental evidence, some recent studies have argued against a mandatory role for DBEs in starch granule formation. The loss of all four DBEs from Arabidopsis leads to the accumulation of maltose and soluble branched glucans which are degraded by α- and β-amylases; however, additional loss of a chloroplastic form of α-amylase (AMY3) restores starch synthesis in this quadruple mutant (Streb et al., Reference Streb, Delatte, Umhang, Eicke, Schorderet, Reinhardt and Zeeman2008). Furthermore, it has been suggested that the role played by DBEs in amylopectin crystallization is a sole feature of the Chloroplastida (see Deschamps et al., Reference Deschamps, Haferkamp, d'Hulst, Neuhaus and Ball2008b). For example, apicomplexan parasites, such as the starch-accumulating Toxoplasma gondii, do not contain functional isoamylases (Coppin et al., Reference Coppin, Varré, Lienard, Dauvillée, Guérardel, Soyer-Gobillard, Buléon, Ball and Tomavo2005), suggesting that, in these organisms at least, crystalline amylopectin formation is possible in the absence of DBEs.
Other enzymes implicated in the pathway
D-enzyme
Plastidial 1,4-α-d-glucan:1,4-α-d-glucan, 4-α-d-glucanotransferase (disproportionating enzyme, D-enzyme, E.C. 2.4.1.25) is present in many different starch-containing organs of plants (Lin et al., Reference Lin, Spilatro and Preiss1988; Takaha et al., Reference Takaha, Yanase, Okada and Smith1993). D-enzyme transfers two of the glucosyl units from malto-triose on to a longer glucan chain, making them available to the β-amylases, and the resulting glucosyl monomer becomes available for export from the plastid via a glucose transporter in the inner envelope membrane (Weber et al., Reference Weber, Rolletschek, Heim, Golombek, Gubatz and Wobus2000; Niittylä et al., Reference Niittylä, Messerli, Trevisan, Chen, Smith and Zeeman2004). Knock-out mutants of D-enzyme in Arabidopsis show reduced rates of nocturnal starch degradation (Critchley et al., Reference Critchley, Zeeman, Takaha, Smith and Smith2001), indicating that this enzyme plays a part in the pathway of (chloroplast) starch degradation. Mutants of C. reinhardtii specifically lacking D-enzyme (in the STA11 locus) show a severe decrease in starch content and clearly indicate a role for the enzyme in starch (amylopectin) synthesis (Colleoni et al., Reference Colleoni, Dauvillée, Mouille, Buléon, Gallant, Bouchet, Morell, Samuel, Delrue, d'Hulst, Bliard, Nuzillard and Ball1999a, Reference Colleoni, Dauvillée, Mouille, Morell, Samuel, Slomiany, Liénard, Wattebled, d'Hulst and Ballb; Ball et al., Reference Ball, Liénard, Wattebled, Steup, Hicks and d'Hulst2003). Analysis of D-enzyme levels in the developing endosperm of wheat is consistent with a role in starch biosynthesis (Bresolin et al., Reference Bresolin, Li, Kosar-Hashemi, Tetlow, Chaterjee, Rahman, Morell and Howitt2005). Its precise role in plant storage tissues remains unclear.
Starch phosphorylase
Starch phosphorylase (SP, E.C. 2.4.1.1) catalyses the reversible transfer of glucosyl units from glucose 1-phosphate to the non-reducing end of α-1,4-linked glucan chains and may be driven in either a synthetic or a degradative direction by the relative concentrations of the soluble substrates. However, the role of SP in higher plant starch metabolism is unclear. Plastidial SP (referred to as Pho1 or the L-form) is characterized by a higher affinity for amylopectin than glycogen, and is inhibited by ADP-glucose (Dauvillée et al., Reference Dauvillée, Chochois, Steup, Haebel, Eckermann, Ritte, Ral, Colleoni, Hicks, Wattebled, Deschamps, d'Hulst, Liénard, Cournac, Puteaux, Dupeyre and Ball2006). Kinetic analysis of maize endosperm Pho1 shows that the phosphorolytic reaction is favoured over the synthetic reaction in the presence of MOS (Mu et al., Reference Mu, Yu, Wasserman and Carman2001). Phosphorylases from various sources occur as homotetrameric or homodimeric assemblies (Nakano and Fukui, Reference Nakano and Fukui1986; Albrecht et al., Reference Albrecht, Greve, Pusch, Kossmann, Buchner, Wobus and Steup1998; Buchbinder et al., Reference Buchbinder, Rath and Fletterick2001). Elution profiles of maize amyloplast SP from gel permeation chromatography are consistent with a homotetrameric form of SP, although evidence suggests SP may exist as monomers or lower complexity multimers when associating with other enzymes of starch synthesis (Liu et al., Reference Liu, Makhmoudova, Lee, Wait, Emes and Tetlow2009). Although the precise role of Pho1 in starch metabolism is unclear, it probably contributes to starch synthesis, as a number of studies have found that both SP/Pho1 gene expression and activity measurements correlate with starch biosynthesis (Van Berkel et al., Reference Van Berkel, Conrads-Strauch and Steup1991; Duwenig et al., Reference Duwenig, Steup and Kossmann1997; Yu et al., Reference Yu, Mu, Wasserman and Carman2001), and SP transcripts in potato increase in the light (Albrecht et al., Reference Albrecht, Koch, Lode, Greve, Schneider-Mergener and Steup2001). Analysis of STA4 mutants of C. reinhardtii, lacking the plastidial SP isoform, show reduced starch content and abnormally shaped granules with high amylose content, arguing for a role for SP in starch biosynthesis (Dauvillée et al., Reference Dauvillée, Chochois, Steup, Haebel, Eckermann, Ritte, Ral, Colleoni, Hicks, Wattebled, Deschamps, d'Hulst, Liénard, Cournac, Puteaux, Dupeyre and Ball2006). In storage tissues the activity of the plastidial isozyme (L-form) of SP may be regulated by proteolysis of a 78-amino acid peptide (termed L78). Removal of L78 by an endogenous protease increased the catalytic activity of SP in the phosphorolytic direction (Chen et al., Reference Chen, Chang, Wu, Cuo, Wu and Juang2002). Phosphorylation of L78 at a single serine residue increases the susceptibility of the plastidial L-form of SP to proteolytic degradation (Young et al., Reference Young, Chen, Lin, Tseng, Wu and Juang2006). This suggests a role for protein phosphorylation in the regulation of catalytic activity of SP, which is supported by other studies that show direct phosphorylation of the protein in storage tissues such as cereal endosperm (Grimaud et al., Reference Grimaud, Rogniaux, James, Myers and Planchot2008; Liu et al., Reference Liu, Makhmoudova, Lee, Wait, Emes and Tetlow2009). One role for Pho1 could be in controlling the availability of MOS, which are required for amylose synthesis (see above), and acting in a ‘clearing’ role similar and complementary to that proposed for the DBEs. It has been suggested that D-enzymes work in conjunction with SP, contributing to starch synthesis via the phosphorolytic SP reaction (Takaha et al., Reference Takaha, Critchley, Okada and Smith1998). According to this model, which is based on the ‘glucan-trimming’ model proposed by Ball et al. (Reference Ball, Guan, James, Myers, Keeling, Mouille, Buléon, Colonna and Preiss1996), short-chain MOS liberated in the trimming reaction by DBEs are converted to longer-chain glucans by D-enzyme, which in turn are available for phosphorolysis by SP, liberating glucose 1-phosphate used to synthesize ADP-glucose by plastidial AGPase. Indeed, the phosphorolytic SP reaction is stimulated by the presence of D-enzyme (Colleoni et al., Reference Colleoni, Dauvillée, Mouille, Morell, Samuel, Slomiany, Liénard, Wattebled, d'Hulst and Ball1999b).
Coordination of enzyme activities during starch granule synthesis
During starch deposition in the seed, all of the enzyme classes (and most of the isoforms within each class) involved in amylopectin synthesis (and degradation) are expressed simultaneously. This implies that some degree of coordination between these potentially counter-productive enzyme activities is required for granule assembly. Functional interactions and physical associations between enzymes involved in amylopectin biosynthesis have been suspected for some time, based on the analysis of enzyme kinetics and mutations in the pathway (Hawker et al., Reference Hawker, Ozbun, Ozaki, Greenberg and Preiss1974; Boyer and Preiss, Reference Boyer and Preiss1979, Reference Boyer and Preiss1981; Gao et al., Reference Gao, Wanat, Stinard, James and Myers1998; Beatty et al., Reference Beatty, Rahman, Cao, Woodman, Lee, Myers and James1999; Dinges et al., Reference Dinges, Colleoni, Myers and James2001, Reference Dinges, Colleoni, James and Myers2003; Nishi et al., Reference Nishi, Nakamura, Tanaka and Satoh2001; Seo et al., Reference Seo, Kim, Scott, Singletary, Wong, James and Myers2002; Yao et al., Reference Yao, Guiltinan, Shannon and Thompson2002, Reference Yao, Thompson and Guiltinan2004; Colleoni et al., Reference Colleoni, Myers and James2003). More recently, biochemical analysis of plastid extracts has provided direct evidence for protein–protein interactions between enzymes of starch synthesis (Tetlow et al., Reference Tetlow, Wait, Lu, Akkasaeng, Bowsher, Esposito, Kosar-Hashemi, Morell and Emes2004, Reference Tetlow, Beisel, Cameron, Makhmoudova, Liu, Bresolin, Wait, Morell and Emes2008; Hennen-Bierwagen et al., Reference Hennen-Bierwagen, Liu, Marsh, Kim, Gan, Tetlow, Emes, James and Myers2008). Experiments with isolated amyloplasts from wheat endosperm have shown that some of the key enzymes of the starch (amylopectin) biosynthetic pathway form heteromeric protein complexes, the formation of which is dependent upon phosphorylation status. Phosphorylation of SBEI, SBEIIb and SP by plastidial protein kinase(s) results in the formation of a protein complex between these enzymes which is lost following in vitro dephosphorylation (Tetlow et al., Reference Tetlow, Wait, Lu, Akkasaeng, Bowsher, Esposito, Kosar-Hashemi, Morell and Emes2004). Our knowledge of the regulation of the enzymes of starch synthesis and degradation by protein phosphorylation and complex assembly has been summarized in a recent review by Kötting et al. (Reference Kötting, Kossmann, Zeeman and Lloyd2010). Protein phosphorylation modifies the catalytic activities of starch branching enzymes in amyloplasts and chloroplasts of wheat (Tetlow et al., Reference Tetlow, Wait, Lu, Akkasaeng, Bowsher, Esposito, Kosar-Hashemi, Morell and Emes2004), and may also play a role in their association with starch granules (Grimaud et al., Reference Grimaud, Rogniaux, James, Myers and Planchot2008). Early studies with SSs and SBEs showed that the activity of the former can be stimulated in the presence of the latter and salts such as sodium citrate (Hawker et al., Reference Hawker, Ozbun, Ozaki, Greenberg and Preiss1974), and the potentially cyclic catalytic activities of SSs and SBEs led some researchers to propose amylopectin synthesis via a ‘synthetase–branching enzyme complex’ (Schiefer et al., Reference Schiefer, Lee and Whelan1973). Recent work with wheat and maize amyloplasts demonstrated protein–protein interactions between SSI, SSII and SBEII isoforms, and the improved stability of these protein complexes under relatively high salt (NaCl) concentrations (Hennen-Bierwagen et al., Reference Hennen-Bierwagen, Liu, Marsh, Kim, Gan, Tetlow, Emes, James and Myers2008; Tetlow et al., Reference Tetlow, Beisel, Cameron, Makhmoudova, Liu, Bresolin, Wait, Morell and Emes2008). Formation of a protein complex of approximately 260 kDa between SSI, SSII and SBEII in the endosperms of wheat and maize requires protein phosphorylation, and in vitro dephosphorylation causes its disassociation (Tetlow et al., Reference Tetlow, Beisel, Cameron, Makhmoudova, Liu, Bresolin, Wait, Morell and Emes2008; Liu et al., Reference Liu, Makhmoudova, Lee, Wait, Emes and Tetlow2009) (Fig. 4). Further analysis of this protein complex in maize endosperm showed that the SBEIIb in the complex is phosphorylated (Liu et al., Reference Liu, Makhmoudova, Lee, Wait, Emes and Tetlow2009). Other, larger protein complexes (of approximately 670 kDa), which include the SSIII isoform, are present in maize endosperm, and formation of these complexes is also phosphorylation dependent (Hennen-Bierwagen et al., Reference Hennen-Bierwagen, Lin, Grimaud, Planchot, Keeling, James and Myers2009). Biochemical analysis of mutants will likely benefit our understanding of the regulation of the pathway of starch biosynthesis. For example, the ae − mutant of maize lacks SBEIIb, which is the major form of SBEII in the endosperm, resulting in starches with reduced branch point frequency and longer glucan chains within clusters (termed a ‘high-amylose’ starch, but is in fact a starch with modified amylopectin) (Hilbert and McMasters, Reference Hilbert and MacMasters1946; Banks et al., Reference Banks, Greenwood and Muir1974; Klucinec and Thompson, Reference Klucinec and Thompson2002). Analysis of protein complexes in the amyloplasts of the ae − mutant reveals a distinct pattern of protein–protein interactions which indicate functional complementation for the loss of SBEIIb by SBEI (which has a low branching frequency with amylopectin, and transfers longer glucan chains than SBEII). In ae − mutant endosperm amyloplasts the wild-type protein complex (SSI, SSIIa and SBEIIb) is replaced by a novel complex consisting of SSI, SSIIa, SBEI, SP and SBEIIa (Liu et al., Reference Liu, Makhmoudova, Lee, Wait, Emes and Tetlow2009). In the protein complex in ae − amyloplasts both SBEI and SP are phosphorylated (Liu et al., Reference Liu, Makhmoudova, Lee, Wait, Emes and Tetlow2009). Furthermore, differences in stromal protein complexes are mirrored in the complement of starch-synthesizing enzymes detected within the starch granules (granule-associated proteins, see below) of each genotype, supporting the idea that protein complexes play a functional role in amylopectin biosynthesis. The presence of phosphorylated forms of proteins in starch granules (granule-associated proteins), which are also components of phosphorylation-dependent protein complexes (Tetlow et al., Reference Tetlow, Wait, Lu, Akkasaeng, Bowsher, Esposito, Kosar-Hashemi, Morell and Emes2004; Grimaud et al., Reference Grimaud, Rogniaux, James, Myers and Planchot2008), reinforces the idea that complexes involved in amylopectin biosynthesis constitute the functional units which become entrapped within the granule (Liu et al., Reference Liu, Makhmoudova, Lee, Wait, Emes and Tetlow2009). The operation of SSI, SSIIa and SBEII isoforms (working as one or more protein complexes) in semi-crystalline cluster formation, and SSIII and SBEI (probably working with other SSs and SBEs) involved in the synthesis of the cluster-connecting glucan chains in the amorphous zones, fits well with the ‘two-step branching and improper branch clearing’ model proposed by Nakamura (Reference Nakamura2002). In this model, glucan extension and branching activities occur in both the cluster and amorphous regions of the granule. During the whole process DBEs play critical roles in trimming the cluster shape at the periphery of the growing granule, since they act on sparsely localized branch points more rapidly than those in the densely packed semi-crystalline lamellae (Ball et al., Reference Ball, Guan, James, Myers, Keeling, Mouille, Buléon, Colonna and Preiss1996; Mouille et al., Reference Mouille, Maddelein, Libessart, Talaga, Decq, Delrue and Ball1996; Nakamura et al., 1998). Consequently, those enzymes involved in synthesis of the amorphous region and in the trimming process, remain as soluble proteins in the amyloplast stroma, or are removed during the washing of starch granules.

Figure 4 Protein–protein interactions between amylopectin-synthesizing enzymes during starch granule formation. Many of the enzymes of amylopectin biosynthesis are in the plastid stroma and also associated with a number of protein complexes (see text for details). One such protein complex (in wheat and maize) occurring between SBEII, SSI and SSIIa is shown, the assembly of which is dependent on protein phosphorylation (Hennen-Bierwagen et al., Reference Hennen-Bierwagen, Liu, Marsh, Kim, Gan, Tetlow, Emes, James and Myers2008; Tetlow et al., Reference Tetlow, Beisel, Cameron, Makhmoudova, Liu, Bresolin, Wait, Morell and Emes2008). In maize SBEIIb is phosphorylated (denoted as ‘P’ symbols in the diagram) (Liu et al., Reference Liu, Makhmoudova, Lee, Wait, Emes and Tetlow2009). In vitro dephosphorylation with alkaline phosphatase causes disassociation of the protein complex assembly; in vivo this function is presumably performed by a plastidial protein phosphatase. The diagram also shows how the proteins, which are present as complexes in the stroma, are partitioned within the starch granule as ‘granule-associated’ proteins, and also shows the granule-associated GBSS. It is proposed that the SS/SBEII protein complexes work at the periphery of the nascent granule, being involved in assembly of the clusters of short- to intermediate-sized glucan chains which eventually form the semi-crystalline lamellae of starch granules within which the enzymes become entrapped. There is currently no evidence to suggest they remain as complexes when they become granule-associated.
The role of protein complex formation between these starch biosynthetic enzymes in starch synthesis is not fully understood, but it is thought that such functional assemblies improve the efficiency of polymer construction as the product of one reaction becomes a substrate for another within the complex (substrate channelling). The formation of complexes of starch metabolic enzymes via protein–protein interactions may directly alter the kinetic properties of individual components of the complex through conformational changes. Other, not mutually exclusive, functions of these multiprotein complexes could be the shielding of the growing polymer from degradative enzymes also present within the plastid. At a higher level of organization, the formation of protein complexes during starch biosynthesis may promote a certain favoured, necessary three-dimensional structure within the growing polymer, e.g. in the case of amylopectin this could be a structure necessary for, or promoting, crystallinity (clustered branch points, side chains of defined length, particular side-chain packing). In this context, such multiprotein complexes may act as a form of ‘carbohydrate chaperonin’.
Direct regulation of enzyme activity, and the regulation of groups of reactions by protein complex formation, in some cases involves the phosphorylation of target proteins followed by the formation of a complex with 14-3-3 proteins; this appears to be a general mechanism for regulating enzymes and pathways in eukaryotic systems (for general reviews of plant 14-3-3 proteins, see Sehnke and Ferl, Reference Sehnke and Ferl2002; Comparot et al., Reference Comparot, Lingiah and Martin2003; Roberts, Reference Roberts2003). These proteins are a structurally highly conserved group of approximately 30–35 kDa, and exist as dimers. Isoforms have been identified in chloroplasts from pea leaves (Sehnke et al., Reference Sehnke, Henry, Cline and Ferl2000), although there is currently no evidence for the presence of 14-3-3 proteins in other plastid types. The involvement of 14-3-3 proteins in the regulation of starch metabolism was proposed by Sehnke et al. (Reference Sehnke, Chung, Wu and Ferl2001), who showed that a form of 14-3-3 protein (from the ε sub-group) is present in Arabidopsis leaf starch as a granule-associated protein. 14-3-3 Proteins have also been detected inside starch granules from developing barley endosperm (Alexander and Morris, Reference Alexander and Morris2006) and immature maize pollen (Datta et al., Reference Datta, Chamusco and Choury2002), further implicating this family of proteins in starch metabolic pathways. Phosphorylation-dependent binding of key starch metabolic enzymes (SSI, SSII, SBEIIa and GBSS) to 14-3-3 proteins was demonstrated in the amyloplasts of developing barley endosperm (Alexander and Morris, Reference Alexander and Morris2006), suggesting a mechanism for protein complex formation between the enzymes of starch biosynthesis described above. Despite the above circumstantial evidence, a direct role for 14-3-3 proteins in starch metabolism remains to be established.
Starch granule proteins
Starch granule proteins are a group of proteins which are consistently found associated with these structures following extensive washing with detergents and acetone as well as protease treatments (Denyer et al., Reference Denyer, Sidebottom, Hylton and Smith1993, Reference Denyer, Hylton, Jenner and Smith1995; Rahman et al., Reference Rahman, Kosar-Hashemi, Samuel, Hill, Abbott, Skerritt, Preiss, Appels and Morell1995; Mu-Forster et al., Reference Mu-Forster, Huang, Powers, Harriman, Knight, Singletary, Keeling and Wasserman1996; Borén et al., Reference Borén, Larsson, Falk and Jansson2004). Granule-associated proteins are routinely found in higher plants and green algae, but it is not clear whether they play a functional role within the granule matrix, or are present as a result of entrapment during polymer biosynthesis. Some proteins appear to be exclusively associated with the starch granules, e.g. GBSS isoforms involved in amylose biosynthesis (Denyer et al., Reference Denyer, Clarke, Hylton, Tatge and Smith1996a). Other proteins that are detected exclusively in starch granules include: SBEIc, a large 152 kDa form of SBEI within the large A-type starch granules of storage starches from the endosperms of Festucoideae (e.g. Triticum, Hordeum and Secale species) (Båga et al., Reference Båga, Nair, Repellin, Scoles and Chibbar2000; Peng et al., Reference Peng, Gao, Båga, Hucl and Chibbar2000), and α-glucan-water dikinase [ATP:α-1,4-glucan, water phosphotransferase (GWD), E.C. 2.7.9.4, previously designated R1], which phosphorylates glucose residues at the C6 position in amylopectin, probably preparing the polymer for degradation by hydrolytic enzymes (Ritte et al., Reference Ritte, Lloyd, Eckermann, Rotmann, Kossmann and Steup2002, Reference Ritte, Scharf, Eckermann, Haebel and Steup2004; Kötting et al., 2005; Hejazi et al., Reference Hejazi, Fettke, Haebel, Edner, Paris, Frohberg, Steup and Ritte2008; Zeeman et al., Reference Zeeman, Kossman and Smith2010). Another enzyme transiently associated with starch granules is phospho-α-glucan-water dikinase [ATP:phospho-α-1,4-glucan, water phosphotransferase (PWD) E.C. 2.7.9.5] involved in phosphorylating amylopectin at the C3 position of glucose residues pre-phosphorylated by GWD during starch degradation (Baunsgaard et al., Reference Baunsgaard, Lütken, Mikkelsen, Glaring, Pham and Blennow2005; Kötting et al., Reference Kötting, Pusch, Tiessen, Geigenberger, Steup and Ritte2005). However, most other granule-associated enzymes appear to be partitioned to varying degrees between the soluble (stromal) phase and starch granules. Other proteins in the starch pathway probably play a functional role at, or inside, the growing granule, but are more loosely associated. The amylopectin-synthesizing enzymes routinely found as granule-associated proteins in cereal endosperms are all involved in the synthesis of short- to intermediate-length glucan chains, which are known to form clusters, resulting in semi-crystalline lamellae (see Ball and Morell, Reference Ball and Morell2003). The gradual, periodic synthesis of amylopectin clusters joined via amorphous lamellae, as proposed by the French (Reference French, Whistler, BeMiller and Paschall1984) and Hizukuri (Reference Hizukuri1986) models, has been suggested to be the cause of entrapment of proteins (specifically, amylopectin biosynthetic enzymes) within granules. However, detailed kinetic analysis of some SS isoforms suggests that granule association of these proteins, at least, may be a product of their increased affinity for longer glucan chains during catalysis (Commuri and Keeling, Reference Commuri and Keeling2001). In higher plants, only a specific group of amylopectin-synthesizing proteins are consistently observed within granules (SSI, SSIIa and isoforms of SBEII), whereas other enzyme classes, which likely play an important role in amylopectin biosynthesis, are either absent from the granule (SSIV, SBEI, SP, isoforms of isoamylase, pullulanase-type DBE and D-enzyme) or are found in very small amounts (SSIII). SSIII and SBEI, respectively, elongate and branch relatively long glucan chains (Gao et al., Reference Gao, Wanat, Stinard, James and Myers1998; Blauth et al., Reference Blauth, Kim, Klucinec, Shannon, Thompson and Guiltinan2002), and it has been proposed these enzymes may function in glucan chain formation between clusters in the amorphous region of the starch granule (Nakamura, Reference Nakamura2002; James et al., Reference James, Denyer and Myers2003). Notably, all of the granule-associated proteins known to be involved in amylopectin synthesis are also components of identified soluble protein complexes in the endosperms of wheat and maize (Hennen-Bierwagen et al., Reference Hennen-Bierwagen, Liu, Marsh, Kim, Gan, Tetlow, Emes, James and Myers2008; Tetlow et al., Reference Tetlow, Beisel, Cameron, Makhmoudova, Liu, Bresolin, Wait, Morell and Emes2008), and it has been suggested that they become entrapped as functional protein complexes during amylopectin cluster formation (Liu et al., Reference Liu, Makhmoudova, Lee, Wait, Emes and Tetlow2009) (see above). Of the starch granule-associated proteins, some are phosphorylated, e.g. SBEII forms (Tetlow et al., Reference Tetlow, Wait, Lu, Akkasaeng, Bowsher, Esposito, Kosar-Hashemi, Morell and Emes2004; Grimaud et al., Reference Grimaud, Rogniaux, James, Myers and Planchot2008). It is not yet clear whether granule-associated phosphoproteins are present as a consequence of protein complex assembly, or if protein phosphorylation causes granule-association.
Analysis of mutants of the starch pathway strengthens the notion that stromal protein complexes involved in α-polyglucan cluster formation become granule localized. A number of mutants in the pathway display loss of proteins from the starch granule proteome (Morell et al., Reference Morell, Kosar-Hashemi, Cmiel, Samuel, Chandler, Rahman, Buléon, Batey and Li2003; Borén et al., Reference Borén, Larsson, Falk and Jansson2004; Regina et al., Reference Regina, Kosar-Hashemi, Li, Pedler, Mukai, Yamamoto, Gale, Sharp, Morell and Rahman2005; Umemoto and Aoki, Reference Umemoto and Aoki2005; Grimaud et al., Reference Grimaud, Rogniaux, James, Myers and Planchot2008), consistent with the idea that their loss (they are components of multi-enzyme complexes in amyloplasts) causes disruption of the complex, preventing the remaining proteins from becoming granule-associated. Some mutations (notably the ae − mutant of maize) cause association of novel proteins within the starch granule, which appear to correlate with the altered composition of protein complexes in the amyloplast stroma (see section on protein complexes).
Other proteins that are not directly involved in starch biosynthesis or degradation, but have no assigned function, have been identified in starch granules. These include a plastid-localized starch granule-associated glycogen synthase kinase (GSK-3)-like protein kinase in Medicago and Arabidopsis. Increased expression of the GSK-3-like kinase, termed MsK4, results in starch accumulation in leaves under saline conditions (Kempa et al., Reference Kempa, Rozhon, Šamaj, Erban, Baluška, Becker, Haselmayer, Schleiff, Kopka, Hirt and Jonak2007). It is hypothesized that this granule-associated protein kinase regulates starch synthesis or turnover during stress. In addition, starch granule-associated amylopectin phosphatases have been identified to play a key role in starch degradation by priming the polymer for degradation via phosphorylation of glucose residues (Fordham-Skelton et al., Reference Fordham-Skelton, Chilley, Lumbreras, Reignoux, Fenton, Dahm, Pages and Gatehouse2002; Kerk et al., Reference Kerk, Conley, Rodriguez, Tran, Nimick, Muench and Moorhead2006; Niittylä et al., Reference Niittylä, Comparot-Moss, Lue, Messerli, Trevisan, Seymour, Gatehouse, Villadsen, Smith, Chen, Zeeman and Smith2006; Sokolov et al., Reference Sokolov, Dominguez-Solis, Allary, Buchannan and Luan2006; Kötting et al., Reference Kötting, Santelia, Edner, Eicke, Marthaler, Gentry, Comparot-Moss, Chen, Smith, Steup, Ritte and Zeeman2009; Comparot-Moss et al., Reference Comparot-Moss, Kötting, Stettler, Edner and Graf2010) and are related to the laforin protein which regulates mammalian glycogen metabolism (Fernandez-Sanchez et al., Reference Fernandez-Sanchez, Criado-Garcia, Heath, Garcia-Fojeda, Medrano-Fernandez, Gomez-Garre, Sanz, Serratosa and Rodriguez de Cordoba2003; Lohi et al., Reference Lohi, Ianzano, Zhao, Chan, Turnbull, Scherer, Ackerley and Minassian2005). Pores have been observed at the surface of starch granules from a number of species (Fannon et al., Reference Fannon, Hauber and BeMiller1992; Glaring et al., Reference Glaring, Koch and Blennow2006), and are present in both A- and B-types in cereals (Kim and Huber, Reference Kim and Huber2008). The pores are openings of channels connecting the external surface to the inside of the granule (Huber and BeMiller, Reference Huber and BeMiller1997). Recent analysis of these channels in maize endosperm indicates the presence of actin-like and tubulin-like (FtsZ) proteins, along with proteins involved in starch biosynthesis such as AGPase and SS (Benmoussa et al., Reference Benmoussa, Hamaker, Huang, Sherman, Weil and BeMiller2010). Their presence implies physical connections between the cytosol, plastid stroma and starch granule. Analysis of developing endosperm cells in wheat by Briarty et al. (Reference Briarty, Hughes and Evers1979) suggested to these authors that enzymes of starch synthesis associate with plastid tubuli at the initial stages of granule deposition, and that there is a direct relationship between starch granule volume and amyloplast tubule volume.
Granule initiation and control of starch granule size
Despite the considerable advances in our knowledge of the pathway of starch biosynthesis, the factors controlling starch granule initiation and granule size remain unclear. For a detailed current overview of initiation of storage glucan synthesis the reader is referred to a recent review by D'Hulst and Mérida (Reference D'Hulst and Mérida2010). The locus of granule initiation is termed the hilum, located in the centre of the growing granule and thought to be essential for granule initiation/priming (Ziegler et al., Reference Ziegler, Creek and Runt2005). It has been suggested that the granule forms from the hilum, from which radially oriented microtubules emanate, and these become the channels that terminate as openings (pores) on the granule surface (Fannon et al., Reference Fannon, Gray, Gunawan, Huber and BeMiller2004). A system of glucan polymer (starch) initiation similar to the proteinaceous priming mechanism of glycogen biosynthesis (Rodriguez and Whelan, Reference Rodriguez and Whelan1985; Whelan, Reference Whelan1986) has been proposed in higher plants, with the discovery of a glycogenin-like starch initiation protein in Arabidopsis (PGSIP1) (Chatterjee et al., Reference Chatterjee, Berbezy, Vyas, Coates and Barsby2005). Loss of isoamylase activity from barley, rice and potato leads to an increase in granule initiation (Burton et al., Reference Burton, Jenner, Carrangis, Fahy, Fincher, Hylton, Laurie, Parker, Waite, van Wegen, Verhoeven and Denyer2002; Bustos et al., Reference Bustos, Fahy, Hylton, Seale, Nebane, Edwards, Martin and Smith2004; Kawagoe et al., Reference Kawagoe, Kubo, Satoh, Takaiwa and Nakamura2005), consistent with the idea that isoamylases suppress the sites of new granule initiation, although DBEs are probably not directly involved in initiating granule synthesis (Delatte et al., Reference Delatte, Trevisan, Parker and Zeeman2005; Wattebled et al., Reference Wattebled, Dong, Dumez, Delvallé, Planchot, Berbezy, Vyas, Colonna, Chatterjee, Ball and D'Hulst2005; Streb et al., Reference Streb, Delatte, Umhang, Eicke, Schorderet, Reinhardt and Zeeman2008). Pullulanase-type DBE (limit dextrinase) activity may also play a role in determining granule size. Down-regulation of a pullulanase-type DBE inhibitor activity in barley causes a reduction in the small (B-type) granules, reduces amylose content, alters amylopectin glucan chain-length distribution and reduces starch content (Stahl et al., Reference Stahl, Coates, Bryce and Morris2004). Specific classes of SS may initiate starch granule formation. Mutants of Arabidopsis lacking SSIV are incapable of synthesizing more than one starch granule per plastid (the single granule has a distinct glucan organization in the hilum), indicating its involvement in granule initiation (Roldán et al., Reference Roldán, Lucas, Delvallé, Planchot, Jimenez, Perez, Ball, D'Hulst and Mérida2007). Recent data by Szydlowski et al. (Reference Szydlowski, Ragel, Raynaud, Lucas, Roldán, Montero, Munoz, Ovecka, Bahaji, Planchot, Pozueta-Romero, D'Hulst and Mérida2009) suggest that the role of SSIV in granule initiation can be replaced by the phylogenetically related SSIII, and that elimination of both SSIII and SSIV prevents starch synthesis altogether in Arabidopsis, thus indicating a dual role for these proteins in granule initiation. Glycogen-like structures have been associated with the priming of insoluble starch-like polyglucans (Puteaux et al., Reference Puteaux, Potocki-Veronese, Remaud-Simeon and Buléon2006), leading Szydlowski et al. (Reference Szydlowski, Ragel, Raynaud, Lucas, Roldán, Montero, Munoz, Ovecka, Bahaji, Planchot, Pozueta-Romero, D'Hulst and Mérida2009) to speculate upon a role for SSIV in the interaction and formation of these polyglucans in the seeding of the starch granule. To date there is no evidence for autoglucosylation activity by SSIII or SSIV acting in a priming role analogous to glycogenin or prokaryotic glycogen synthase.
There is huge variation in the size and morphology of starch granules from different species and genotypes within a species, although in many species granule size is homogeneous for a given developmental stage (Jane et al., Reference Jane, Kasemsuwan, Leas, Ames, Zobel and Robyt1994). Populations of different granule size show different physicochemical characteristics (reviewed by Lindeboom et al., Reference Lindeboom, Chang and Tyler2004). Granule size and morphology range from < 0.5 μm diameter for the spherical granules in cells of the picophytoplanktonic green alga Ostreococcus tauri (Ral et al., Reference Ral, Derelle, Ferraz, Wattebled, Farinas, Corellou, Buléon, Slomianny, Delvallé, d'Hulst, Rombauts, Moreau and Ball2004), ~5 μm for the round, flattened granules of Arabidopsis (Delvallé et al., Reference Delvallé, Dumez, Wattebled, Roldán, Planchot, Berbezy, Colonna, Vyas, Chatterjee, Ball, Mérida and D'Hulst2005; Wattebled et al., Reference Wattebled, Planchot, Dong, Szydlowski, Pontoire, Devin, Ball and D'Hulst2008), 2–30 μm for polyhedral granules of maize (Buléon et al., Reference Buléon, Colonna, Planchot and Ball1998) and 20–100 μm for the large, oval granules in potatoes and pea (Pisum sativum L.) cotyledons (Buléon et al., Reference Buléon, Colonna, Planchot and Ball1998). Cereals belonging to the Festucoid family of grasses, such as wheat, barley, oats and rye, are characterized by a bimodal (or trimodal) distribution of starch granules in storage tissues (Evers, Reference Evers1973; Meredith, Reference Meredith1981; Bechtel et al., Reference Bechtel, Zayas, Kaleikau and Pomeranz1990). Large lenticular-shaped A-type granules (15–30 μm diameter) are formed early in endosperm development, while the smaller B-type ones (averaging 5–9 μm in diameter) are formed later (Parker, Reference Parker1985). Recent observations suggest a third, smaller, class of starch granules ( < 5 μm, termed C-type granules) in cereals such as wheat (Wilson et al., Reference Wilson, Bechtel, Todd and Seib2006). The difficulty in isolating and quantifying C-type granules has led to their inclusion as B-type granules in many studies. Analysis of A- and B-type starch granules from developing wheat endosperm indicates that they have distinct physicochemical properties (Wei et al., Reference Wei, Zhang, Chen, Zhou, Xu, Wang and Chen2010). The selective advantage conferred upon plants displaying a bi/trimodal distribution of starch granules in storage tissues is at present unclear, but the formation of smaller-sized granules may offer a more efficient means of packing more stored carbon in a cell. It has been proposed that the different classes of starch granules in wheat endosperm (the large A-type, and smaller B- and C-type granules) are produced in different cell types at different stages of endosperm development (May and Buttrose, Reference May and Buttrose1959; Briarty et al., Reference Briarty, Hughes and Evers1979; Parker, Reference Parker1985). The smaller B-type granules present in wheat and barley form inside protrusions from the amyloplast termed stromules (stroma containing tubules) (Buttrose, Reference Buttrose1963; Köhler et al., 1997; Langeveld et al., Reference Langeveld, van Wijk, Stuurman, Kijne and de Pater2000; Bechtel and Wilson, Reference Bechtel and Wilson2003). Experiments with potato suggest that the presence of proteins with starch-binding domains may in some way limit glucan chain extension, and ultimately, granule size (Ji et al., Reference Ji, Oomen, Vincken, Bolam, Gilbert, Suurs and Visser2004). The specific factors controlling the size of starch granules in any species are unknown, however.
Summary
Starch is a raw material of fundamental significance to many human activities, and ultimately to civilization. It is therefore of crucial importance to understand the various processes involved in starch granule assembly, and the regulatory factors governing carbon allocation for this process inside plastids, so that increased food demands and the desire for specific starches in the food and non-food sectors can be met. In addition to the important contribution being made by plant breeders, future increases in starch yield and improvements in quality of agriculturally produced starches will be driven by the isolation of natural mutants or through the use of genetic engineering. Underpinning this latter technology is the cloning and characterization of genes directly and indirectly associated with starch metabolism for any given crop.
The availability of complete plant genome sequences now offers more detailed insight into the potential genes involved in starch metabolism, and points to key differences between different model systems in terms of the number of isoforms of particular enzymes (e.g. multiple SSII and SBEII genes in rice, which are not present in Arabidopsis). Different suites of enzymes are clearly involved in the synthesis of leaf and endosperm starches in rice, and such information should present a note of caution when attempting to make generalized conclusions from one species to another about the roles of particular isoforms.
The isolation and analysis of natural and insertion mutants has made, and will continue to make, an invaluable contribution towards our understanding of starch metabolism in higher plants. The tendency has been to try to explain the functions of genes based solely on their mutant phenotype. The many pleiotropic effects arising from single mutations, coupled with research indicating that some of the starch metabolic enzymes may operate within complexes, suggest that this approach is, in some cases, too simplistic. Some of the mutant phenotypes, therefore, are probably the result of the disruption of various protein complexes and associations, and not just the mutated gene.
The diverse pleiotropic effects observed with various mutations in genes of the starch metabolic pathways indicate that there are probably many more interactions between the enzymes of starch metabolism and other signalling pathways than have been identified to date. More work is required to discover new interactions between starch metabolic enzymes in starch-storing crops, and to elucidate the mechanisms and signalling cues that govern this aspect of metabolic regulation, as well as to identify the components controlling protein complex formation in the plastid. Signalling pathways involved in connecting photosynthate delivery in source leaves with plastidial carbon metabolism in sink tissues are still not clearly understood (Smith and Stitt, Reference Smith and Stitt2007). The exact role of the different protein complexes involved in starch biosynthesis needs to be elucidated. Given that assembly of most of these is dependent on protein phosphorylation, further research promises to uncover many new potential targets for manipulation of starch structure and yield.