1. Introduction
Palaeotethys-related ophiolites and metamorphic rocks mark the northward subduction of the ocean crust and subsequent collision of Gondwana and Laurasia (Stampfli & Borel, Reference Stampfli and Borel2002; Rossetti et al. Reference Rossetti, Monié, Nasrabadi, Theye, Lucci and Saadat2017). Carboniferous metamorphic rocks (Variscan) are important in this regard, and are ascribed to high-pressure metamorphism during subduction of the oceanic crust (Faryad & Kachlik, Reference Faryad and Kachlik2013; Omrani et al. Reference Omrani, Moazzen, Oberhänsli, Tsujimori, Bousquet and Moayyed2013; Rossetti et al. Reference Rossetti, Monié, Nasrabadi, Theye, Lucci and Saadat2017) or high-temperature metamorphism by crustal thickening and subsequent thinning by erosion (Gerdes et al. Reference Gerdes, Wörner and Henk2000) or magma underplating (Büttner & Kruhl, Reference Büttner and Kruhl1997). The Variscan orogeny is related to the collision of Gondwanan terrains with Laurasia during the Late Palaeozoic (mainly 300–350 Ma; Kröner & Romer, Reference Kröner and Romer2013). Variscan magmatic and metamorphic rocks are exposed in the central and eastern basement rocks of the Pontides (to the west of the study area; Okay & Şahintürk, Reference Okay and Şahinturk1997; Topuz et al. Reference Topuz, Altherr, Satir and Schwarz2004a, b; Reference Topuz, Altherr, Schwarz, Dokuz and Meyer2007; Dokuz, Reference Dokuz2011; Dokuz et al. Reference Dokuz2011; Ustaömer et al. Reference Ustaömer, Robertson, Ustaömer, Gerdes, Peytcheva, Robertson and Ünlügenç2013), in the Caucasus (to the north of the study area; Somin, Reference Somin and Korikovsky1991; Perchuk & Philippot, Reference Perchuk and Philippot1997), the Transcaucasus (Zakariadze et al. Reference Zakariadze, Karpenko and Bazilev1998; Gamkrelidze et al. Reference Gamkrelidze, Shengelia, Tsutsunava, Chung, Yichiu and Chikhelidze2011; Mayringer et al. Reference Mayringer, Treloar, Gerdes, Finger and Shengelia2011) and in Central Iran (Bagheri & Stampfli, Reference Bagheri and Stampfli2008; Kargaranbafghi et al. Reference Kargaranbafghi, Neubauer and Genser2015; Zanchi et al. Reference Zanchi, Malaspina, Zanchetta, Berra, Benciolini, Bergomi, Cavallo, Javadi and Kouhpeyma2015).
There are several models for the formation of the Variscan metamorphic and igneous rocks in the Pontides, Transcaucasus and Balkan regions (Ustaömer et al. Reference Ustaömer, Robertson, Ustaömer, Gerdes, Peytcheva, Robertson and Ünlügenç2013). These rocks are formed along the southern margin of Eurasia, due to rifting at the northern margin of Gondwana and drifting of the continental slivers and their accretion to the south Eurasia margin in the Carboniferous (Adamia et al. Reference Adamia, Lordkipanidze and Zakariadze1977; Dercourt et al. Reference Dercourt, Ricou and Vrielynck1993; Stampfli & Borel, Reference Stampfli and Borel2002; Nikishin et al. Reference Nikishin, Ziegler, Bolotov and Fokin2011). Ustaömer & Robertson (Reference Ustaömer and Robertson1993) considered the closure of a marginal back-arc basin in response to the pre-Late Jurassic southward subduction and accretion. Some researchers assumed either a southward subduction for the Palaeotethys (Şengör & Yilmaz, Reference Şengör and Yilmaz1981; Şengör et al. Reference Şengör, Yilmaz, Sungurlu, Dixon and Robertson1984; Göncüoğlu et al. Reference Göncüoğlu, Turhan, Şentürk, Özcan, Uysal, Yaliniz, Bozkurt, Bozkurt, Piper and Winchester2000; Romano et al. Reference Romano, Brix, Dorr, Fiala, Krenn, Zulauf, Robertson and Mountrakis2006; Zulauf et al. Reference Zulauf, Romano, Dorr and Flala2007) or a combined northward and southward subduction of the Rheic Ocean (Dokuz et al. Reference Dokuz2011). Alternative models propose that northward subduction below Eurasia accreted several Gondwana-derived continental blocks during Carboniferous–Permian times to the southern margin of Eurasia. This caused the closure of the Rheic Ocean and the generation of a Cordilleran-type orogeny (Nikishin et al. Reference Nikishin, Ziegler, Bolotov and Fokin2011). Rolland et al. (Reference Rolland, Sosson, Sh and Sadradze2011) explained the Variscan high-temperature metamorphism and related magmatism as due to an active margin setting which followed the accretion of these Gondwana-derived blocks to Eurasia. In this model, metamorphism could have been achieved without any collisional thickening. Due to scarcity of studies on the Palaeotethys-related rocks in Iran, it is difficult to assess the timing and geodynamics of continental assembly during the Palaeozoic. A relatively small tectonic window of metamorphic rocks, composed of pelitic, psammitic and mafic protolith compositions along with serpentinized harzburgite, gabbro and diabase, occurs on the eroded surface of a NW–SE-trending anticline in the Allahyarlu area, NW Iran, known as the Allahyarlu complex (Babakhani & Nazer, Reference Babakhani and Nazer1991; Fig. 1). These rocks are unconformably covered by Late Cretaceous carbonate and volcanic units. The complex is located at a crucial position regarding the exact location of the Palaeotethys and Neotethys sutures in NW Iran, the Caucasus and eastern Turkey (Fig. 1a). Some researchers consider the Allahyarlu complex as an ophiolitic mélange (e.g. Babakhani & Nazer, Reference Babakhani and Nazer1991; Barzegar & Pourkermani, Reference Barzegar and Pourkermani2011; M. Sudi Ajirlu, unpub. MSc thesis, Univ. Tabriz, 2011). They interpret these rocks as the southern continuation of the Sevan–Akera ophiolite belt of the lesser Caucasus (Stöcklin, Reference Stöcklin1977; Stampfli, Reference Stampfli1978; Berberian & King, Reference Berberian and King1981; Boulin, Reference Boulin1988; Galoyan et al. Reference Galoyan, Rolland, Sosson, Corsini, Bilio, Verati and Melkonyan2009), while Eftekharnejad et al.(Reference Eftekharnejad, Alavi-Naini and Behrouzi1993) argued that the Allahyarlu complex is a continuation of the south Mashhad ophiolites (Shafaii Moghadam et al. Reference Shafaii Moghadam, Li, Ling, Stern, Khedr, Chiardia, Ghorbani, Arai and Tamura2015) and/or the Shanderman and Masuleh complexes (Omrani et al. Reference Omrani, Moazzen, Oberhänsli, Tsujimori, Bousquet and Moayyed2013; Rossetti et al. Reference Rossetti, Monié, Nasrabadi, Theye, Lucci and Saadat2017) along the Palaeotethys suture in North Iran (Fig. 1a). However, to fulfil the Penrose ophiolite definition, the Allahyarlu complex lacks some typical components such as pillow basalts and sheeted dykes, and serpentinized peridotites only have limited exposure. The metamorphic rocks are thrust on the ophiolite mélange. There is no published account on the petrology, geochemistry and geochronology of the Allahyarlu rock suite. Babakhani & Nazer (Reference Babakhani and Nazer1991) considered the Early Cimmerian orogenic phase responsible for metamorphism in the area. On the other hand, Stampfli & Borel (Reference Stampfli and Borel2002) interpreted this type of rock as part of a Cimmerian ribbon, which rifted from Gondwana during the Late Permian and collided with Eurasia during the Late Triassic. This event is thought to terminate the history of Palaeotethys subduction and resulted in the accretion or obduction of NW Iran Neotethys ophiolites. Besides, ongoing intra-oceanic subduction to the north of the Anatolian–Armenian blocks in Jurassic time shows that there was no Cimmerian continental fragment along the Izmir–Ankara–Erzincan suture (Rolland et al. Reference Rolland, Pernicek, Kaymakci, Sosson, Barrier and Avagyan2012; Topuz et al. Reference Topuz, Göçmengil, Rolland, Celik, Zack and Schmitt2013; Hässig et al. Reference Hässig, Rolland, Sahakyan, Sosson, Galoyan, Avagyan, Bosch and Muller2015). Therefore, the question of the correspondence of the Sevan–Akera suture in NW Iran is raised: is it the continuation of the Allahyarlu suture or should it rather be considered as the lateral continuation of the Zagros suture (through the Khoy ophiolite; Khalatbari Jafari & Babaie, Reference Khalatbari Jafari, Babaie and Sorkhabi2016; Shafaii Moghadam et al. Reference Shafaii Moghadam, Corfu, Stern and Lotfibakhsh2018), offset by a N–S transform fault (e.g. Rolland, Reference Rolland2017)? Further, the age of metamorphism of the Allahyarlu complex is put into question, as there is no absolute geochronological constraint on these rocks. Babakhani & Nazer (Reference Babakhani and Nazer1991) attributed folding of the Cretaceous sediments and following magmatism of the area to the impact of a Laramide-type (active margin) orogeny, corresponding to ongoing subduction below southern Iran. Barzegar & Pourkermani (Reference Barzegar and Pourkermani2011) consider folding of Palaeocene to Eocene sandstones and conglomerates in the area as a result of the Late Alpine orogeny. However, Allahyarlu-complex metamorphism may rather correspond to an older tectonic event like the Variscan high-pressure (HP) metamorphism evidenced by Rossetti et al. (Reference Rossetti, Monié, Nasrabadi, Theye, Lucci and Saadat2017) to the west of the Caspian Sea along the same Allahyarlu–Hovai fault. The relationship of the Allahyarlu-ccomplex metamorphism to Neotethys subductions, Alpine collisions or a preserved older tectonic phase is not yet resolved. In this paper, we consider the metamorphic rocks, which form the crystalline basement representing the oldest units in the Allahyarlu area. Field relationships and petrography as well as whole rock and mineral chemistry of these rocks are reported and complemented by 40Ar/40Ar dating on white mica and amphibole in meta-psammitic and metabasic lithologies, respectively. We will show that the oldest rocks of the Allahyarlu complex were metamorphosed up to the amphibolite facies during the Hercynian orogeny and thus are unrelated to the Cimmerian phase. This result is used to discuss the geodynamic context of NW Iran in relation to adjacent areas in east Anatolia–Armenia and the Lesser Caucasus.

Fig. 1. (a) The locality of the study area in NW Iran and in relation to the main Neotethys and Palaeotethys sutures in the Middle East. Some examples of Variscan metamorphic rocks are shown with peak metamorphic ages: (1) Eastern Pontides (Topuz et al. Reference Topuz, Altherr, Schwarz, Dokuz and Meyer2007); (2) Armenia (Rolland et al. Reference Rolland, Sosson, Sh and Sadradze2011); (3) Rasht (Rossetti et al. Reference Rossetti, Monié, Nasrabadi, Theye, Lucci and Saadat2017); (4) Anarak (Buchs et al. Reference Buchs, Bagheri, Martin, Herman and Arculus2013). (b) The study area in the northern part of the Ahar Block. The legends are: 1. remnants of the oceanic crust; 2. local structural trends; 3. main faults; 4. hidden faults; 5. country borders; 6. anticline; 7. syncline; 8. depression; 9. thrust; 10. Allahyarlu–Hovai thrust.
2. Geological setting of the Allahyarlu area
Northwest Iran, east Anatolia and the Lesser Caucasus constitute a complicated geological terrain. There are many studies and opinions on the geology of this area, but there is no general consensus on its geodynamic evolution. NW Iran is attributed to different geological and tectonic subdivisions. It is located in the west Alborz–Azerbaijan (Nabavi, Reference Nabavi1976), Alborz range (Stöcklin, Reference Stöcklin1968), Western Alborz (Stampfli, Reference Stampfli1978) and Central Iran (Aghanabati, Reference Aghanabati2004) geological units. The Alborz range is a composite poly-orogenic belt within the Alpine–Himalayan orogen, the main geomorphological features of which are brought about by Alpine orogeny. The Allahyarlu area in Iranian Azerbaijan is located between the Caucasus and Zagros orogenic belts, at the northern boundary of the Ahar Block (Fig. 1b; Stöcklin, Reference Stöcklin1968; Berberian & King, Reference Berberian and King1981; Sudi Ajirlu & Moazzen, Reference Sudi Ajirlu and Moazzen2014). The late Alpine orogenic phase is evidenced by folding of Eocene to Oligocene/Miocene flysch-type sediments. The Ahar Block basement is characterized by greenschist- to amphibolite-facies psammites, metapelites and metabasites, which are the subject of this study. The study area can be divided into three geologic units: (i) old metamorphic rocks, (ii) ophiolite mélange and Cretaceous sedimentary rocks and (iii) Tertiary volcanic rocks. The basement rocks and overlying Cretaceous sediments have been thrust onto the Pre- to Late Cretaceous ophiolite rock suite, comprising ultramafic, mafic and intermediate igneous rocks (Fig. 2). All these rock units are subsequently folded into a relatively small (∼12 km long and 2 km across) anticline; and later a further low-angle fault has cut out the core of the anticline. The older rocks are covered by Late Cretaceous sedimentary and volcanic units, and thick outcrops of Palaeocene–Eocene volcanic and pyroclastic rocks (Fig. 2). Metamorphic rocks are exposed on an eroded surface in the core of the anticline (Fig. 3a). The main faults cross-cutting the basement are top-to-the-south thrusts along the anticline axis, which have juxtaposed the basement units onto the younger rocks. Some minor NE–SW and NW–SE-trending strike-slip faults also cross-cut most units. The metamorphic and ophiolitic rocks are exposed only at the anticline core and are covered by widespread Palaeogene volcanic rocks in other parts of the area. The contacts between the ophiolitic rocks and the metamorphic rocks are defined by faults. The Pre- to Late Cretaceous ophiolite mélange is composed of serpentinized peridotite, gabbro, diabase and pelagic limestone with minor amounts of radiolarian chert. Serpentinized peridotites are mainly restricted to the shear zones and appear as sporadic lenses or blocks. The protolith was harzburgite in which spinel chemistry is in favour of a supra-subduction zone and fore-arc setting (Z. Salimi, unpub. PhD thesis, Univ. Tabriz, 2019). Gabbros in the ophiolitic mélange are isotropic, and associated with small outcrops of diorite and micro-diorite. Diabase appears mainly as dykes. The Late Cretaceous units are formed by a sequence of deep marine fossil-bearing limestone of Lower Cenomanian age (Babakhani & Nazer, Reference Babakhani and Nazer1991), a layer of andesite and hyaloclastite, sandy limestone with thin chert layers and spilitic andesite with some pillow structures. These rocks are thrust onto Campanian and Maastrichtian sediments (Babakhani & Nazer, Reference Babakhani and Nazer1991). Metamorphosed mafic rocks are scarce and are mainly represented by amphibolites (Fig. 3b) with alternating dark hornblende-rich and pale plagioclase-rich layers, with a distinct lineation. The main metamorphic rocks of the area are garnet-±-chlorite white mica schists, which have a pelitic to semi-pelitic protolith composition. The pelitic and semi-pelitic rocks show a well-developed high-temperature mineral foliation (Fig. 3c), underlined by garnet + white mica with a main NW–SE direction, parallel to the anticline axis. Some slightly metamorphosed (greenschist facies) mafic dykes occasionally cross-cut the metapelitic schists. Garnet gneisses are present as massive bodies with a folded foliation defined by mica- and quartz-rich layers (Fig. 3d). Garnet-free gneisses occur as interlayers in pelitic schists, possibly indicating a shallow turbiditic environment of deposition for the parental rocks. In some parts of the gneisses, the quartz-rich layers reach a thickness of up to 30 cm and form distinct quartzite layers.

Fig. 2. The geological map and cross-section of the studied area, modified from Babakhani & Nazer (Reference Babakhani and Nazer1991).

Fig. 3. (a) Outcrop of ophiolitic and metamorphic rocks at the erosion surface on the Allahyarlu anticline. (b) Outcrop of amphibolites. (c) Metapelitic rocks with pervasive S1 foliation. (d) Outcrop of gneiss with quartz- and plagioclase-rich pale and biotite-rich dark bands.
3. Analytical methods
Whole rock analyses were carried out on 14 representative samples (8 amphibolites, 4 metapelitic schists, 2 gneisses) that were selected from a rock suite of more than 150 samples. Whole rock analyses were performed using X-ray fluorescence (XRF) and inductively coupled plasma mass spectrometer (ICP-MS) methods at the Institut des Géosciences de l’Environnement, Université Grenoble Alpes, France, and at Actlabs laboratories in Ontario, Canada (4Litho-Research analytical package). About 1 kg of each sample was crushed using a steel-jaw mill and was pulverized to 200 mesh. A known amount of sample was mixed with lithium metaborate and fused on a gas heater. The resulting glass discs were used for XRF analyses of major oxides. After digestion in acid and dilution they were used for minor and trace elements analyses using an ICP-MS. International standards were used for calibrations. The uncertainties for the major oxides are better than 2 % and for the minor and trace elements better than 5 %. Mineral chemical analyses were done using a Japan Electron Optics Ltd (JEOL) JXA-8530 F Superprobe at the Institut für Mineralogie, Universität Münster, Germany. The acceleration voltage during analyses was 15 kv, with 15 nA specimen current and counting times of 10 s for the peak and 5 s for the background (except for Na and K for which 5 s peak and 2.5 s background counting time was applied). Natural and synthetic standards were used for calibration. The raw data were processed by the ZAF software. The quality of electron-probe microanalysis (EPMA) data were checked regarding the total oxides analysed and the formula unit calculated for the minerals. 40Ar/39Ar dating on muscovite and hornblende separates from the metamorphic rocks was performed at UMR Géosciences Azur, Nice, France. The samples were crushed to a 500–800 μm size fraction and cleaned in an ultrasonic bath. Muscovite grains were carefully hand-picked under a binocular microscope to select only grains without evidence of alteration or inclusions. These grains were packaged in aluminium foil and irradiated along with hornblende neutron fluence monitor Hb3gr, with an age of 1072 Ma (Turner et al. Reference Turner, Forbes and Dillon1971), in the McMaster University nuclear reactor, Hamilton, Canada. Argon isotopic interferences on K and Ca were determined by irradiation of KF and CaF2 pure salts from which the following correction factors were obtained: (36Ar/37Ar)Ca = 0.000288 ± 0.000016; (39Ar/37Ar)Ca = 0.000727 ± 0.000041; (40Ar/39Ar)k = 0.00969 ± 0.00038; (38Ar/39Ar)k = 0.01297 ± 0.00045. All samples were analysed with adevice consisting of: (a) an IR-CO2 laser of 100 kHz used at 5–15 % during 60 s, (b) a lens system for beam focusing, (c) a steel chamber, maintained at 10−8–10−9 9 bar, with a drilled copper plate, (d) an inlet line for purification of gases including two Zr–Al getters and (e) a multi-collector mass spectrometer (Argus VI from Thermo-Fisher). The ARGUS VI spectrometer has a mass resolution of 225–250 and a measured sensitivity of 7 × 10–14 mol V–1 at 200 μA trap current. The high-mass Faraday detector is fitted with a 1011 Ω resistor and is used specifically for measuring the 40Ar+ beam intensity. The Faraday detectors used for other Ar isotope measurements are fitted with 1012 Ω resistors. Direct measurement of collector noise for ten separate runs gave averages of 4 × 106 V on the 1011 Ω circuit and 1 × 105 V on the 1012 Ω circuit. Collector gain calibration is performed by the computer-controlled application of predetermined voltages to each collector. Over the course of 1 year, the relative gain between collectors did not exceed 19 ppm. The compact discrete dynode electron multiplier channel was used for single collector peak jumping measurements. System blanks were measured every three experiments. The blanks typically accounted for less than 0.06 % of the 40Ar, 0.6–3.81 % of the 38Ar, and 0.12–0.71 % of the 36Ar. Mass discrimination for the mass spectrometer was monitored by regularly analysing air pipette volumes. We used ArArCalc © software v2.5.2 for data processing (Koppers, Reference Koppers2002). Decay constants are those given by Steiger & Jäger (Reference Steiger and Jäger1977). Uncertainties on apparent and plateau ages are given at the 1σ level including the uncertainty on the 40Ar*/39Ar ratio of the monitor. The one-sigma errors reported on plateau, isochron and total gas ages include the error on the irradiation factor J. Atmospheric 40Ar was estimated using a value of the initial 40Ar/36Ar of 295.5.
4. Rock descriptions and mineral compositions
The pelitic schists of the study area include garnet–chlorite–muscovite and garnet–biotite–muscovite schists (Table 1). The major minerals in the chlorite-bearing schists are quartz, chlorite, muscovite and garnet, the minor phases are plagioclase and Fe-oxides and accessory phases are graphite and tourmaline. Foliation is well developed in these rocks, and a crenulation cleavage can be observed (Fig. 4a; mineral name abbreviations are from Whitney & Evans, Reference Whitney and Evans2010). The main textures are lepidoblastic and porphyroblastic. Garnet occurs as idioblastic porphyroblasts, up to 1.5 mm across, with inclusions of quartz and muscovite. About 1 mm long plagioclase crystals are occasionally present as porphyroblasts. Fine- (0.5 mm) to relatively coarse (1.5 mm)-grained biotite in the biotite–garnet–muscovite schist is extensively altered into chlorite. Quartz, biotite, muscovite and garnet are the major phases and plagioclase, secondary chlorite, opaque minerals, tourmaline and zircon are the minor and accessory phases. The main lepidoblastic texture of the groundmass of this rock type is formed by parallel aligned mica flakes. The pervasive schistosity is deformed by secondary deformational phase, reflected by microfolds (Fig. 4a). Garnet porphyroblasts up to 2 mm across have inclusions of muscovite and quartz. They show symmetrical pressure shadows. Quartz is the main mineral in the shadow (Fig. 4b). Petrographical studies indicate retrograde changes of biotite and garnet into chlorite. Also chlorite is formed on the microfolds limbs and hinges of the second deformation (Fig. 4a). These observations testify for an amphibolite (formation of garnet and biotite) and overprinting greenschist (mainly chlorite formation) polyphase metamorphism for the studied rocks. Mineral compositions are relatively constant within individual samples but are relatively different among the studied samples. In the analysed samples, garnet is almandine-rich (Table 2) with formula of Alm52.4-81.5Sps0.9-26.1Grs24.2-0.7Prp1.2-14.7 (Fig. 4c). Garnet in sample ZS-2 N, which is a semipelitic rock with high quartz content, shows the highest spessartine and the lowest almandine contents (Fig. 4c). Apart from this sample, garnet in other samples shows very limited variations in the major oxide contents across the crystals, indicating lack of significant chemical zoning. White mica is mainly muscovite-rich with considerable phengite and low paragonite end-member contents occasionally (Table 3). Margarite component was not recognized (Fig. 4d). No aluminosilicate polymorph was found in the Allahyarlu samples. The fabric of the gneisses is defined by alternating mica- and garnet-rich and quartz–feldspar-rich layers, respectively. The main minerals are quartz, plagioclase, garnet, muscovite and biotite. The minor phases include chlorite, tourmaline, epidote, titanite and opaque minerals, and zircon is the accessory phase (Table 1). Graphite can be found in some samples. The main textures are granoblastic, formed by quartz and feldspar, within the microlithon domains of the rock and lepidoblastic formed by parallel mica flakes in the mica-rich parts of the rock, which compose the schistosity domains. Garnet (up to 3 mm across) appears as relatively large crystals with inclusions of biotite, muscovite and quartz. Muscovite flakes are relatively large (up to 1 mm) in some samples. Retrograde products are chlorite after biotite and garnet and sericite after feldspar. The ratio of quartz to other minerals is high, which along with the presence of graphite in some samples indicates a paragneiss nature for the studied samples. The chemistry of garnet and white mica is very close to those in the schists (Fig. 4a, b). Amphibolites can be divided into garnet-free and garnet-bearing varieties (Table 1). Hornblende and plagioclase are the main phases. Titanite, calcite, rare biotite, epidote, Fe-oxides and zircon constitute the minor and accessory phases. The main texture of the rocks is nematoblastic, formed by parallel alignment of the hornblende prisms (up to 4 mm long), making the rock lineation. This is the pervasive deformational phase in the amphibolite samples (Fig. 5a). In some samples fine-grained (<0.5 mm) quartz is present in low modal amounts. Garnets occur in garnet amphibolites as small grains (∼0.5 mm) with inclusions of hornblende, plagioclase and Fe-oxides. Samples with garnet lack epidote. Rare quartz occurs along with plagioclase (up to 2 mm) as a light envelope wrapping the garnet crystals (Fig. 5b). Amphibole in amphibolites is calcic (Table 4) and shows a compositional range from ferro-tschermakite to magnesio-hornblende (Fig. 6a). Based on Na+K versus Si in the formula unit, amphibole compositions in samples ZS-1-14 and ZS-43 range from hornblende to tschermakite types, while amphibole in sample ZS-3-B ranges from edenite to pargasite (Fig. 6b). The garnet composition (Table 2) is Alm56.7-59.6Sps1.3-6.2Grs24.2-28.8Pyp10-14.5 (Fig. 6c), and plagioclase ranges from pure albite to andesine (Table 5; Fig. 6d). Plagioclase composition in sample SZ-1-14 is An29.6-37.07Ab61.2-69.92Or0.0-0.8, in sample ZS-3-B is An0.5-31.4Ab68.0-99.2Or0.0-0.6 and in sample ZS-43 is An3.4-33.6Ab65.7-95.9Or0.5-1.2. Primary chlorites in textural equilibrium with garnet are Fe- and Mg-rich, with Fe varying from 1.38 to 2.24 in the formula unit (based on 14 oxygen atoms) while Mg number is from 1.64 to 2.31 atom per formula unit. Ca and Mn contents are negligible (Table 6).
Table 1. Mineral assemblages (mineral name abbreviations after Whitney & Evans, Reference Whitney and Evans2010)

++ Major phase; + minor phase; o accessory phase. * EPMA. ▴ Whole-rock analysis. ♦ 40Ar/39Ar dating.

Fig. 4. (a) Foliation in muscovite schist. The main foliation (S1) is folded by S2. (b) Garnet with pressure shadow structures in textural equilibrium with metamorphic chlorite (both in cross-polarized light). (c). Garnet composition in garnet schist (samples ZS-2 N, ZS-18-19, ZS-28, ZS-13 and ZS-42) and gneiss (samples ZS-1-15 and ZS-1-12), which is almandine-rich. (d) Composition of plagioclase in the amphibolite samples. Plagioclases in sample ZS-3-B are albite to andesine, in sample ZS-1-14 are andesine and in sample ZS-43 they are albite to andesine.
Table 2. Representative garnet analyses in the studied rocks (Am: amphibolite; Sch: schist; Gns: gneiss). The formula unit is calculated based on 12 oxygen atoms
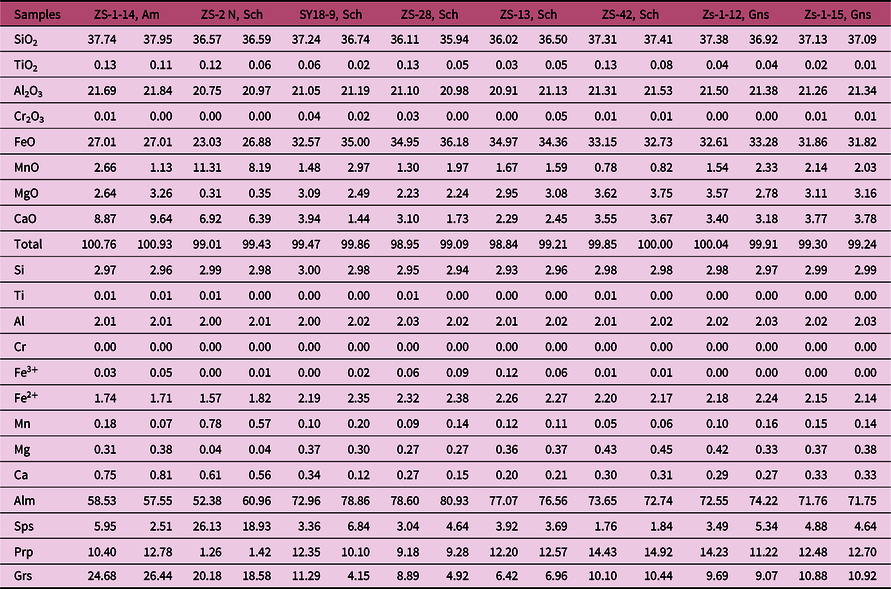
Table 3. Representative muscovite analyses in the studied rocks (Sch: schist; Gns: gneiss). The formula unit is calculated based on 11 oxygen atoms


Fig. 5. Photomicrographs of (a) amphibolite and (b) garnet amphibolite from the Allahyarlu area (both in plain polarized light).
Table 4. Representative amphibole analyses in the studied rocks (Grt Am: garnet amphibolite; Am: amphibolite). The formula unit is calculated based on 13 cations (CaNaK) and 23 oxygen atoms


Fig. 6. Composition of amphibole (a, b), garnet (c) and feldspar (d) in the studied amphibolites.
Table 5. Representative plagioclase analyses in the studied amphibolite samples (Grt Am: garnet amphibolite; Am: amphibolite). The formula unit is calculated based on 8 oxygen atoms

Table 6. Representative chlorite analyses in the studied garnet schist samples. The formula unit is calculated based on 14 oxygen atoms

5. Metamorphic P–T estimates
The P–T estimates based on different methods and calibrations indicate a relatively wide range of metamorphic conditions for the Allahyarlu metamorphic rocks. Since all studied rocks contain hydrous minerals such as muscovite, chlorite and amphibole, we assumed water saturation condition for the metamorphism. Pelitic rocks lack aluminosilicate polymorphs, which hinders application of the most reliable thermobarometers for these rocks. In the amphibolites, there is no suitable mineral paragenesis that would allow determining pressure precisely. The rocks show no indications for partial melting. This suggests a maximum T limit for the metamorphism of c. 650 °C. Since metapelitic and metabasic rocks share similar structures and textures and are closely associated in the field, we consider it likely that they share the same metamorphic history.
5.a. Temperature estimates for garnet mica schists
Quartz, garnet, muscovite, plagioclase and chlorite are in textural equilibrium in the studied samples. Biotite is altered into chlorite. Due to the lack of suitable mineral paragenesis for pressure estimates in the pelitic rocks, nominal pressures are used to calculate temperature. Thermodynamic modelling and calibrations of the Grt–Ms thermometer were studied by Krogh & Raheim (Reference Krogh and Raheim1978), Green & Hellman (Reference Green and Hellman1982), Hynes & Frost (Reference Hynes and Frost1988) and Wu et al. (Reference Wu, Wang and Yang2002). This thermometer is based on Fe–Mg exchange between muscovite and garnet in thermodynamic equilibrium and can be applied to rocks in the temperature range 480–700 °C and pressures of 3 to 14 kbar (Wu et al. Reference Wu, Wang and Yang2002). It is applicable if the Mg content of muscovite is less than 0.13 and the Fe content is more than 0.04 atom per formula unit based on 11 oxygen atoms. Our samples meet these criteria (Tables 2, 3). The garnet solid solution model is from Holdaway (Reference Holdaway2000), and muscovite is treated as a symmetric Fe–Mg–AlVI ternary solid solution. Wu & Zhao (Reference Wu and Zhao2006) proposed two equations for temperature calculations, one for garnet without ferric iron and another for garnet with 50 % ferric iron. An asymmetric Quaternary solid solution is considered for garnet in the calibration. The studied garnets do not have considerable ferric iron in their compositions, therefore we have used model (A) of Wu et al. (Reference Wu, Wang and Yang2002). Temperatures are estimated for two samples for nominal pressures of 2 to 9 kbar. The core compositions of garnet are used. The range of temperature is 506 to 558 °C, with an average of 532 °C. Wu et al. (Reference Wu, Wang and Yang2002) consider a ± 5 °C uncertainty for this thermometer. Chlorite appears both as replacing garnet and biotite and as relatively large flakes parallel to the main foliation (Fig. 4a). The composition of the second type of chlorite, considered to be in equilibrium with garnet, is used for temperature calculations using the Grt–Chl thermometer. This thermometer is based on the Fe–Mg exchange between chlorite and garnet in the presence of quartz (Dickinson & Hewitt, Reference Dickinson and Hewitt1986; Ghent et al. Reference Ghent, Stout, Black and Brothers1987; Grambling, Reference Grambling1990). We used the calibration by Grambling (Reference Grambling1990), with an assumed ±8 °C uncertainty. Nominal pressures of 3, 4, 6 and 8 kbar are used for T calculations. Due to limited pressure dependence of this method (Grambling, Reference Grambling1990), the calculated temperatures for different pressures are identical. The T range is 525–586 °C with an average of ∼556 °C. This temperature is similar to T estimates from the Grt–Ms thermometry above, indicating lower amphibolite facies peak metamorphic conditions. To estimate the peak P–T conditions of the pelitic rocks, sample ZS-13 with the highest Al2O3 content (more pelitic than psammitic) was used for calculation by the pseudosection method by means of the Domino/Theriak software (De Capitani & Brown, Reference De Capitani and Brown1987) in the MnCN-KFMASH system. The internally consistent dataset of Berman (Reference Berman1988) was used for the calculations. The pseudosection is shown in Figure 7a. Grt + Chl + Pl and Grt + Chl + Bt fields on the pseudosection are narrow, considering the temperature changes. Muscovite content in white mica (Fig. 7b), and almandine content in garnet (Fig. 7c) are calculated. Based on EPMAs, the white mica muscovite content is 0.74 mole %, and the garnet almandine content is 0.76 mole %. These isoplets are intersected in two points (Fig. 7d), indicating a temperature and pressure range for metamorphism (stars in Fig. 7a, d). Pressure of 4.5–5 kbar and temperature of c. 530–570 °C can be considered for peak metamorphism of the pelitic samples using these diagrams.

Fig. 7. (a) Pseudosections for one pelitic schist (ZS-13); (b, c) calculated isochems for muscovite (b) and almandine (c) contents in the minerals; (d) intersection of muscovite in white mica isochem of 0.74 mole % and almandine in garnet isochem of 0.76 mole % indicates pressure of c. 4.5 kbar and temperature of 530 to 570 °C.
5.b. P–T estimates for the amphibolites
Hornblende, garnet and plagioclase are the main minerals in textural equilibrium in the amphibolites. Several thermobarometers are applied to these rocks. Hornblende–plagioclase (Hbl–Pl) thermometer (Blundy & Holland, Reference Blundy and Holland1990; Schmidt, Reference Schmidt1992, Blundy & Holland, Reference Blundy and Holland1994; Stein & Dietl, Reference Stein and Dietl2001) is calibrated based on edinitic and tschermakitic substitutions in amphibole. Blundy & Holland (Reference Blundy and Holland1994) calibrated this thermometer with edenite + albite = richterite + anorthite and edenite + quartz = tremolite + albite reactions. Since quartz is absent or occurs in very low amounts in our amphibolite samples, we have used the quartz-free version of the thermometer (Blundy & Holland, Reference Blundy and Holland1994). The range of temperature for three samples is 601 to 862 °C. Samples ZS-1-14 and SZ-3B show relatively higher temperatures. Titanite is present in the studied amphibolite samples. This shows that Ti was saturated in the rock systems and Ti content in hornblende is mainly controlled by temperature (Raase, Reference Raase1974; Ernst & Liu, Reference Ernst and Liu1998). Ti in Hbl thermometry of Otten (Reference Otten1984) was used to further constrain the metamorphic temperature of the amphibolite samples. This thermometer is P-independent and gives temperatures of 581–617 °C. Grt–Pl thermometry (Graham & Powell, Reference Graham and Powell1984; Perchuk et al. Reference Perchuk, Aranovich, Podlesskii, Lavrant’eva, Gerasimov, Fed’kin, Kitsul, Karsakov and Berdnikov1985) gives temperatures of 513 to 581 °C, and Grt–Hbl thermometer (Krogh, Reference Krogh2000) indicates 600 °C for garnet amphibolite metamorphism. Pressure estimates for the Allahyarlu amphibolites are problematic owing to the lack of suitable mineral paragenesis. We consider a pressure of c. 5 kbar estimated from the pelitic rocks as realistic for the amphibolites, since the two rock types occur together in the field, showing similar deformation and metamorphic events, but this might be a minimum estimate owing to the presence of garnet in this lithology. The range of temperature estimated for the pelitic rocks in the area is 505–586 °C for different samples. These temperatures are close considering the uncertainties in the T estimation. Temperatures estimated for the amphibolites are at the same range (513–670 °C). We regard the highest temperatures from the Hbl–Pl calibration for samples ZS-1-14 and ZS-3B as unrealistic, since these temperatures correspond to the granulite facies, while no granulite facies minerals (e.g. orthopyroxene) have been found in the studied samples. Based on thermobarometry results for both mafic and pelitic rocks, an average pressure of c. 5 kbar and an average temperature of c. 600 °C can be considered for the metamorphism in the Allahyarlu complex. Considering an average density of 2700 kg m−3 for the upper crustal rocks, a lithostatic pressure of 1 kbar corresponds to depth of burial of ∼3.75 km. An average pressure of 5 kbar for the Allahyarlu metamorphic rocks is therefore equivalent to ∼18 km depth of burial. Taking 600 °C as the average temperature, the geothermal gradient for the area was ∼33 °C km−1 during metamorphism, which might develop in response to a collisional context in the continental crust or to a thick magmatic arc setting (e.g. Jamieson et al. Reference Jamieson, Beaumont, Fullsack, Lee, Treloar and O’Brien1998).
The results for P–T estimates on the studied rocks are summarized in Table 7.
Table 7. Pressure–temperature estimates for the garnet schist and amphibolite samples

6. Amphibolite whole rock chemistry
Eight representative amphibolite samples were analysed for major and trace elements (Table 8). SiO2 contents of the analysed samples form a very narrow range from 49.1 to 51.3 wt %. Al2O3 contents are 13.74–15.31 wt %, and MgO varies from 5.1 to 7.33 wt %. The Fe2O3 (total) content is 9.16–10.26 wt %. These chemical features point to a mafic (basaltic) composition of the protolith. TiO2 content of the rocks is 1.30–1.85 wt %, Na2O content is 2.14–3.01 wt %, while K2O content is 0.49–0.90 wt %. Considering the whole rock geochemistry of the studied amphibolites, relatively high TiO2, MgO and Fe2O3 (total) contents versus very low K2O and low SiO2 contents may testify for an igneous (ortho-amphibole) nature of the protolith (e.g. Moghazi et al. Reference Moghazi, Hassanen, Hashad and Mohamed2001). We have used the whole rock chemistry of the amphibolite samples to constrain the nature of the magmatic series and the tectonic setting of the protolith. Immobile and relatively mobile elements during greenschist to amphibolite facies metamorphism (Pearce & Cann, Reference Pearce and Cann1973; Coish, Reference Coish1977; Floyd & Winchester, Reference Floyd and Winchester1978; Rollinson, Reference Rollinson1993) are used in this regard. The results from these two types of elements are comparable. The studied samples are enriched in large-ion lithophile elements (LILE) compared to high field strength elements (HFSE). Ta, Nb, P and Ti (all HFSE) show negative anomalies, and heavy rare earth elements (HREE) contents show negligible variation.
Table 8. Whole-rock chemistry of the amphibolite samples. Major oxides in mass % and the trace and rare earth elements in ppm
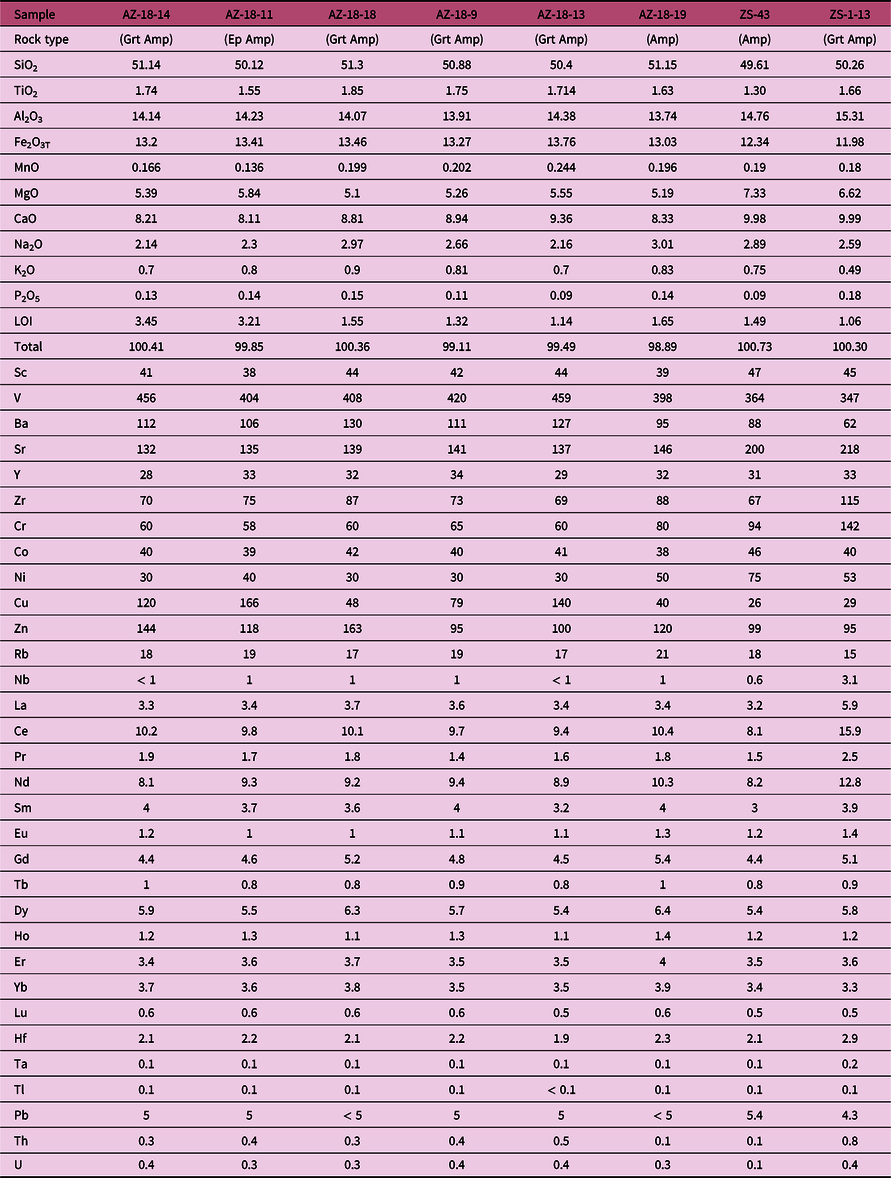
7. The age of metamorphism
Muscovite separated from two gneiss samples (ZS-1-12 and ZS-15) and hornblende separated from three amphibolite samples (ZS-3b, ZS-43a and ZS-43b) were used for 40Ar–39Ar dating. The resulting data are provided in the Appendix.
7.a. Sample ZS-1-12, muscovite
This sample is well defined by a 12-step spectrum. The central portion of the spectrum representing more than 91 % of the released 39Ar gives a well-defined plateau age of 333.7 ± 0.8 Ma. The isochron correlation gives slightly older age (338.2 ± 2.0 Ma), and the inverse isochron shows a similar within-error age (334.5 ± 1.3 Ma) to the plateau age (Fig. 8). Therefore, given the very homogeneous behaviour of this sample, it is in good agreement with a crystallization of the muscovite at c. 334 Ma. The total fusion age of the sample is also similar within error (338 ± 0.3 Ma), pointing to a very low alteration for this sample.

Fig. 8. 39Ar–40Ar plateau ages (c. 325 and 338 Ma) for muscovite separates from the gneiss samples.
7.b. Sample ZS-15, muscovite
This sample also shows a homogeneous spectrum, and a well-defined plateau age with ten heating steps. The central portion of the spectrum, representing 96.6 % of the released 39Ar, gives a plateau age of 324.0 ± 0.8 Ma. The total fusion age of this sample is of 323.6 ± 0.2 Ma, which is similar within-error to the plateau age (Fig. 8). The isochron correlations also correspond very well with this age (normal isochron age 324.0 ± 1.3 Ma; inverse isochron age 323.0 ± 1.9 Ma). Therefore, given the consistency of the released Ar–Ar signal in this sample, we interpret this age as a geologically meaningful age, which corresponds to the crystallization of the muscovite at c. 326 Ma.
7.c. Sample ZS-3b, hornblende
This sample gave eight heating steps. It shows a bell-shape Ar release pattern starting with lower ages (c. 93 Ma) and culminating at ages of 323–325 Ma in the central part of the Ar–Ar spectrum. Thus, this sample shows a more complex thermal history than the muscovites. Initial crystallization age was partly preserved in the core of the grain, as emphasized by similar Ca/K ratios obtained by EPMA in amphibole core and in 323–325 Ma age steps by spectrometric analysis (Fig. 9). These high Ca/K ratios correspond to the higher temperature steps apart from the melting step, which still preserved a younger age component and a lower Ca/K ratio. The lower step ages are in agreement with a mixture of amphibole with a <100 Ma phyllosilicate, as shown by Ca/K ratios which appear to be lower than amphibole rims (Ca/K = 24). Therefore, these ages are regarded as mixed ages with no geological significance. The two older steps agree with a minimum age of 325 Ma, close to the age of the high-temperature metamorphic amphibole. The lower-temperature steps correspond to the contribution of some small alteration phases due to a recrystallization stage with a maximum age of 93 Ma.

Fig. 9. 39Ar–40Ar plateau ages (c. 174 to 248 Ma) for amphibole separates from the amphibolite samples.
The age pattern of amphiboles is not due to a core–rim variation towards actinolite. If this was the case, the corresponding Ca/K ratios would be much higher, as actinolite has higher Ca/K ratio than hornblende. Besides, actinolite has a very low K content and generally contains very little argon.
7.d. Sample ZS-43, hornblende
The Ar release pattern of this sample is similar to that of sample ZS-3b, with ten steps. It shows a bell shape starting with lower ages (minimum c. 122 Ma) and culminating at an age of 288 Ma in the central part. Thus, this sample also indicates a complex thermal and crystallization history. As for sample ZS-3b, a correlation is found in the Ca/K versus age plot, highlighting a tendency towards higher Ca/K ratios in the higher age steps (Fig. 9). However, the highest Ca/K values are still lower than the values analysed by EPMA. Thus the step age of 288 Ma is interpreted as a minimum age for the initial metamorphic growth of the amphibole, and appears to have been significantly lowered in this sample by the contribution of high-K phase similar to sample ZS-3b. Extrapolating the ages to the corresponding EPMA values returns an age of ∼350 Ma, which is coherent with the minimum age of sample ZS-3b amphibole. Accordingly, this sample has been strongly altered and recrystallized during a second event, with a maximum age of c. 122 Ma.
7.e. Sample ZS-43b, hornblende (ZS-43 duplicate)
This experiment revealed similar Ar–Ar spectra to the previous sample. As above, the highest age step also preserves the highest Ca/K ratio (Ca/K = 33), and thus the corresponding age of 283 Ma is a minimum age for the initial metamorphic growth of the muscovite. The recrystallization and/or retrograde of this mineral is shown by the contribution of a younger component with lower Ca/K ratios contribution, with a maximum age of 120 Ma (Fig. 9). According to the Ca/K ratios versus age plot, an initial age of 320–330 Ma could be hypothesized, which falls exactly within the range of muscovite plateau ages and of the maximum sample ZS-3b amphibole age. Based on the above ages, the main metamorphic event recorded in the Allahyarlu metamorphic rocks is well defined by muscovite, which has been less severely altered than the amphiboles. The muscovite provides ages in the range 326–334 Ma. This is the minimum closure age but the maximum age on the spectrum. This age is similar to the minimum age of sample ZS-3b hornblende, suggesting contemporaneous crystallization of amphibole and muscovite during the HT peak. Ages younger than 120 Ma (sample ZS-X43) and 93 Ma (sample ZS-3b) can be attributed either to partial resetting of amphibole during a retrograde event or a mixture of different reservoirs for argon. This episode could be responsible for the widespread greenschist facies changes observed in the samples.
8. Discussion and conclusions
In order to find the nature of the protolith for the amphibolites, major oxides and trace elements are used. All samples plot in the igneous protolith or ortho-amphibolite fields in diagrams of Na2O/Al2O3 versus K2O/Al2O3 (Garrels & Mackenzie, Reference Garrels and Mackenzie1971), MnO versus TiO2 (Werner, Reference Werner1987), MgO–CaO–FeO (Walker et al. Reference Walker, Joplin, Lovering and Green1959) and Zr versus MgO (Irvine & Baragar, Reference Irvine and Baragar1971) in Figure 10 (all Fe is considered as FeO). Considering an igneous origin for the amphibolite protolith, the original magma type is characterized. The amphibolite samples with SiO2 contents of 49.61 to 51.15 wt % and N2O + K2O of 2.84 to 3.64 are similar to gabbro and gabbro–diorite or basalt and basaltic andesite in composition (Fig. 11a). The parental magma of the amphibolite protolith was metaluminous with a tholeiitic nature (Fig. 11b, c and d). It is similar to oceanic basalt (Fig. 12a), with tholeiitic composition transitional between mid-oceanic and island arc basalt (Fig. 12b, c, d). Rb, Hf, Ta and Th contents of the studied samples indicate an island arc setting for the protolith (Fig. 12e and f). V and Ti contents in mafic rocks are proxies to discriminate between different tectonic settings of mafic magmatism (Shervais, Reference Shervais1982). Vanadium has three oxidation states, which are stable in reduced (V3+) and oxidized (V4+ and V5+) conditions, while Ti can be found only as Ti4+ in magma. Therefore variation of V concentrations against Ti concentrations is indicative of oxygen fugacity changes in magma. Shervais (Reference Shervais1982) showed that a Ti versus V diagram can discriminate tholeiites formed in relation to an arc, mid-ocean ridge basalt (MORB) and ocean island basalts. Also this diagram allows discriminating fore-arc and back-arc basin basalts. The reason for this is that the crystal/liquid partitioning coefficient for V varies with oxygen fugacities from >1 to <<1. According to Pearce & Cann (Reference Pearce and Cann1971, Reference Pearce and Cann1973), the f O2 for magmas formed in the oceanic environment increases in the order island arcs > back-arc basins > ocean islands > MORB. Discrimination diagrams involving Ti, V, Zr, Th and Co are used to distinguish the tectonic setting for the parental rocks of the amphibolites (Fig. 13). The samples plot within or close to the back-arc basin basalt fields in these diagrams. Trace element compositions of the samples are plotted on spider diagrams normalized to normal (N-) MORB (normalizing values are from Sun & McDonough, Reference Sun, McDonough, Saunders and Norry1989). The studied samples show distinct positive anomalies in Rb, K and Pb and negative anomalies in Th, Nb, Ta (TNT) (Fig. 14a). These features clearly show a subduction-related system for the formation of the protolith of the amphibolites. The spider patterns are compared with a typical enriched and depleted back-arc basin basalt (Fig. 14a) and typical island arc basalt (island arc basalts in Guguan, Mariana arc; Pearce et al. Reference Pearce, Stern, Bloomer and Fryer2005; Fig. 14b). The patterns in Figure 14 show enrichment in LILE (such as Rb, K, Pb, Sr and Ba), compared to HFSE. Some HFSE such as Ta, Nb, P and Ti show negative anomalies. LILE are mobile elements, released from the downgoing slab or associated sediments at relatively low temperatures, causing heterogeneity in the overlying mantle wedge (Stern et al. Reference Stern, Morris, Bloomer and Hawkins1991; Pearce et al. Reference Pearce, Stern, Bloomer and Fryer2005; Pearce & Stern, Reference Pearce, Stern, Christie, Fisher, Lee and Givens2006), while all subduction-mobile elements including MREE, Pb, P, Th and Rb are released by super-critical fluids at higher temperature and depth (Woodhead, Reference Woodhead1989; Elliott et al. Reference Elliott, Plank, Zindler, White and Bourbon1997; Pearce & Stern, Reference Pearce, Stern, Christie, Fisher, Lee and Givens2006). Considering enrichment of the samples in shallow subduction components, along with some enrichment in total subduction components, it seems that the magma-forming protolith of the amphibolites was enriched at shallow to medium depth of a subduction system. The samples show trace elements patterns similar to both island arc and back-arc basin basalts. Negative anomaly of Nb along with slight enrichment in LREE and LILE for the samples are features indicating arc-related magmatism for the protolith of the rocks. Ta–Nb–Th negative anomalies and Pb positive anomaly can indicate involvement of continental crust or sedimentary material in the petrogenesis of the protolith (Saunders et al. Reference Saunders, Storey, Kent, Norry, Storey, Alabaster and Pankhurst1992; Nagudi et al. Reference Nagudi, Koeberl and Kura2003). All these features are in favour of a back-arc sialic setting for the formation of the studied mafic rocks.

Fig. 10. Whole rock chemistry of the amphibolite samples indicates an igneous (ortho-amphibolite) protolith for them.

Fig. 11. The protolith of the amphibolite samples had a basalt to basaltic andesite lithology (a) and metaluminous (b) and tholeiitic (c, d) nature.

Fig. 12. The initial magma forming the protolith of the amphibolites was an oceanic basalt (a) and had island arc to MORB affinities (b, c, d). They were formed in a volcanic arc setting (e, f).

Fig. 13. A back-arc setting can be considered for the studied amphibolites based on immobile and trace elements contents.

Fig. 14. Spider diagrams for the studied samples. The samples show similarities to both enriched and depleted back-arc basalts (a) and are more similar to typical island arc basalts, indicating shallow subduction components (b). The normalization values are from Sun & McDonough (Reference Sun, McDonough, Saunders and Norry1989).
Pelitic and semi-pelitic rocks studied here represent continental material, metamorphosed in the amphibolite facies; P–T estimates for both meta-sedimentary and meta-igneous rocks are consistent with a medium P–medium T regional gradient in response to collisional processes affecting continental crust or to a thick magmatic arc setting. The whole-rock chemistry of the amphibolites of the study area indicates a subduction-related affinity of the protolith and a possible back-arc sialic setting. In the continuation of the Allahyarlu–Hovai Fault, to the east of the Ahar Block, Rossetti et al. (Reference Rossetti, Monié, Nasrabadi, Theye, Lucci and Saadat2017) described HP rocks (blueschists to eclogites), which formed in the Early Carboniferous (c. 350 Ma, Ar–Ar dating). The formation of the Allahyarlu metamorphic complex is therefore found to be slightly younger but coherent with a metamorphic context bordering the Palaeotethys subduction zone. By analogy, to the west, in the Georgian Transcaucasus, a similar domain of high-temperature metamorphism is dated at 320–330 Ma (Mayringer et al. Reference Mayringer, Treloar, Gerdes, Finger and Shengelia2011; Rolland et al. Reference Rolland, Pernicek, Kaymakci, Sosson, Barrier and Avagyan2012), and is found directly south of a 350 Ma suture (Perchuk & Philippot, Reference Perchuk and Philippot1997). However, it is still difficult to correlate these occurrences as the Cenozoic deformation is quite complex and has offset the sutures due to strike-slip faults like the Araks or Talesh faults (Fig. 1). The geochemistry of igneous rocks from the study area (our unpublished data) is not indicative of a continental collision setting. These rocks show arc-related features and some adakitic signatures. Our findings are more compatible with a model suggesting a long-lived active continental margin history for the southern margin of Eurasia, likely brought about by a northward subduction of Palaeotethys as in the Caucasus region (Rolland et al. Reference Rolland, Sosson, Sh and Sadradze2011). In conclusion, the association of magmatic and metamorphic rocks of the Allahyarlu area is more in agreement with an active continental-margin magmatic arc during the Variscan orogeny and does not show any clear evidence of a continental collision. Figure 15 depicts a simple model for the tectonic setting of the Allahyarlu Variscan metamorphic rocks, where the MP–MT gradient could be related to a phase of active margin thickening due to flat-slab subduction (Fig. 15). In summary, the studied area is characterized by subduction of the Palaeotethys oceanic crust beneath the southern margin of Eurasia, which formed a thickened active continental margin including volcanic arc. The possible steep slab caused extension behind the volcanic arc and created an ensialic back-arc basin, in which protolith of meta-sedimentary rocks deposited. Also mafic rocks intruded within this extension zone, which made amphibolites later. The extensional basin transformed to a compressional one by changing of the steep slab into a flat slab. This caused metamorphism of the sedimentary and mafic igneous rocks in the amphibolite facies c. 326–334 Ma ago. The ophiolitic rocks, which are younger than the metamorphic rocks (c. 247 Ma; Z. Salimi, unpub. PhD thesis, Univ. Tabriz, 2019), were emplaced in the area. Further, the metamorphic rocks experienced a late greenschist facies deformation event, indicated by younger ages (<97 Ma) of amphibole. This may be related to a phase of tectonic reactivation due to an accretionary event which occurred in relation to the Neotethyan domain. The metamorphic and ophiolitic rocks were folded in a small anticline, then were covered by widespread and thick tertiary volcanic rocks.

Fig. 15. A schematic model for the formation of the Variscan metamorphic rocks of the Allahyarlu area in NW Iran.
Acknowledgements
This paper is a part of the PhD dissertation of Z.S., which is supported by University of Tabriz. We would like to thank Leila Rezaei and Mahleqa Rezaei Bargoshadi for their help with the field work. Thanks are also due to Jasper Berndt and Beate Schmitte for their help with the EMP analyses. Invaluable comments and suggestions by Federico Rossetti and an anonymous referee and great editorial contributions and help from Kathryn Goodenough are highly appreciated.
Appendix Summary of obtained Ar–Ar data
