Introduction
Nematodes Gnathostomatidae (Railliet, 1895 emend. Nicoll, 1927) are grouped into seven genera (Bain et al., Reference Bain, Mutafchiev, Junker and Schmidt-Rhaesa2014). Of these, the genus Echinocephalus Molin, 1858, harbours 13 species the mature stages of which infect only elasmobranchs, mainly marine rays. These include: Echinocephalus uncinatus Molin, 1858 (type species) parasitic in the dasyatid stingray Bathytoshia centroura (Syn. Trygon brucco Bonaparte, 1834) from Mediterranean sea, Echinocephalus spinosissimus von Linstow, 1905, Echinocephalus multidentatus Baylis & Lane, Reference Baylis and Lane1920, and Echinocephalus southwelli Baylis & Lane, Reference Baylis and Lane1920, parasitic in the myliobatid and dasyatid rays from India and Sri Lanka; Echinocephalus pseudouncinatus Millemann, 1951, parasitic in Heterodontus francisci (Heterodontidae) and Myliobatis california (Myliobatidae) from Mexico; Echinocephalus sinensis Ko, 1975, parasitic in Aetobatus flagellum (Aetobatidae) from the South China Sea and the South Pacific; Echinocephalus pteroplatae Wang, Zhao & Chen, 1978, from the Gymnura japonica (Gymnuridae) from Taiwan; Echinocephalus janzeni Hoberg, Books, Molina-Ureña & Erbe, 1998, parasitic in the dasyatid stingray Styracura pacifica (Syn. Himantura pacifica Beebe & Tee-Van, 1941) from the East Pacific in Costa Rica and Mexico; Echinocephalus overstreeti Brooks & Deardorff, Reference Brooks and Deardorff1988 and Echinocephalus inserratus Moravec & Justine, Reference Moravec and Justine2021 parasitizing dasyatids and heterodontids from the Southwest Pacific; and Echinocephalus caniculus Saad et al., Reference Saad, Suthar, Theisen, Palm and Gargouri2022, parasitic in the scyliorhinid cat shark Scyliorhinus canicula from Tunisia (Baylis & Lane, Reference Baylis and Lane1920; Moravec & Justine, Reference Moravec and Justine2021; Saad et al., Reference Saad, Suthar, Theisen, Palm and Gargouri2022). Reports of Echinocephalus species infecting freshwater elasmobranchs are restricted to Echinocephalus diazi Troncy, Reference Troncy1970 and Echinocephalus daileyi Deardorff et al., Reference Deardorff, Brooks and Thorson1981, both found in South American stingrays of the Potamotrygonidae Garman, 1877. Echinocephalus diazi, however, was described from a ray identified as Potamotrygon hystrix caught in Lake Maracaibo (Troncy, Reference Troncy1970), a large brackish lake connected to the Gulf of Venezuela (Laval et al., Reference Laval, Imberger and Findikakis2005). Later, this gnathostomatid nematode was twice reported in the marine ray Styracura schmardae Werner, 1904 (Syn. Trygon schmardae Werner, 1904; Himantura schmardae Werner, 1904) (Deardorff et al., Reference Deardorff, Brooks and Thorson1981). Echinocephalus daileyi, on the other hand, was found infecting P. hystrix and Potamotrygon circularis (a junior synonym of Potamotrygon motoro (Müller & Henle, 1841) from a freshwater environment from the Amazon and Orinoco River basins (Baylis & Lane, Reference Baylis and Lane1920; Deardorff et al., Reference Deardorff, Brooks and Thorson1981; Moravec & Justine, Reference Moravec and Justine2021). In addition to these two named species, Lacerda et al. (Reference Lacerda, Takemoto and Pavanelli2009) reported Echinocephalus sp. parasitizing the spiral valve of the largespot river stingray Potamotrygon falkneri Castex & Maciel, 1963, from the upper Paraná River, in southeastern Brazil, although these authors did not identify the parasite on a specific level.
Potamotrygonidae harbours two subfamilies: the recently created Styracurinae, which is composed of S. schmardae (type species) and Styracura pacificus Beebe & Tee-Van, 1941 (Syn. Dasyatis pacificus Beebe & Tee-Van, 1941; Himantura pacificus Beebe & Tee-Van, 1941) (Carvalho et al., Reference Carvalho, Loboda and Silva2016); and Potamotrygoninae, a marine-derived lineage composed of 38 exclusively freshwater stingray species distributed in four genera and occurring in the main South American watersheds, with the greatest diversity observed in the Amazon (Lovejoy et al., Reference Lovejoy, Bermingham and Martin1998, Reference Lovejoy, Albert and Crampton2006; Carvalho et al., Reference Carvalho, Lovejoy, Rosa, Reis, Kullander and Ferraris2003; Silva & Loboda, Reference Silva and Loboda2019; Fontenelle et al., Reference Fontenelle, Marques, Kolmann and Lovejoy2021; Loboda et al., Reference Loboda, Lasso, Rosa and Carvalho2021).
The present study reports, using light and scanning electron microscopy, and DNA based phylogenetic approach based on multiple genes (small subunit ribosomal ribonucleic acid (SSU rDNA) and mitochondrial cytochrome c oxidase I (COI)) and dating inference analyses, a new Echinocephalus species infecting the spiral intestines of specimens of the potamotrygonin species P. motoro from the Tapajós River, in the Amazon basin, Brazil.
Material and methods
Sampling and morphological studies
A total of 35 specimens of P. motoro were caught using hook and line or longline in the Tapajós River, in the municipal region of Santarém, in October 2018 (2°26′23.30″S; 54°53′39,67″W and 2°20′34.68″S; 54°53′27.69″W) and October 2021 (2°23′34.41″S; 54°43′57.53″W) (fig. 1). The fish were transported alive to a field laboratory on the shores of the Tapajós River and the methodology was approved by the ethics research committee of the Federal University of São Paulo (CEUA N 4457040219). Capture and genetic heritage access were authorized by the Brazilian Ministry of the Environment (SISBIO n◦ 66053–1 and SisGen n◦ AD28DC2, respectively).
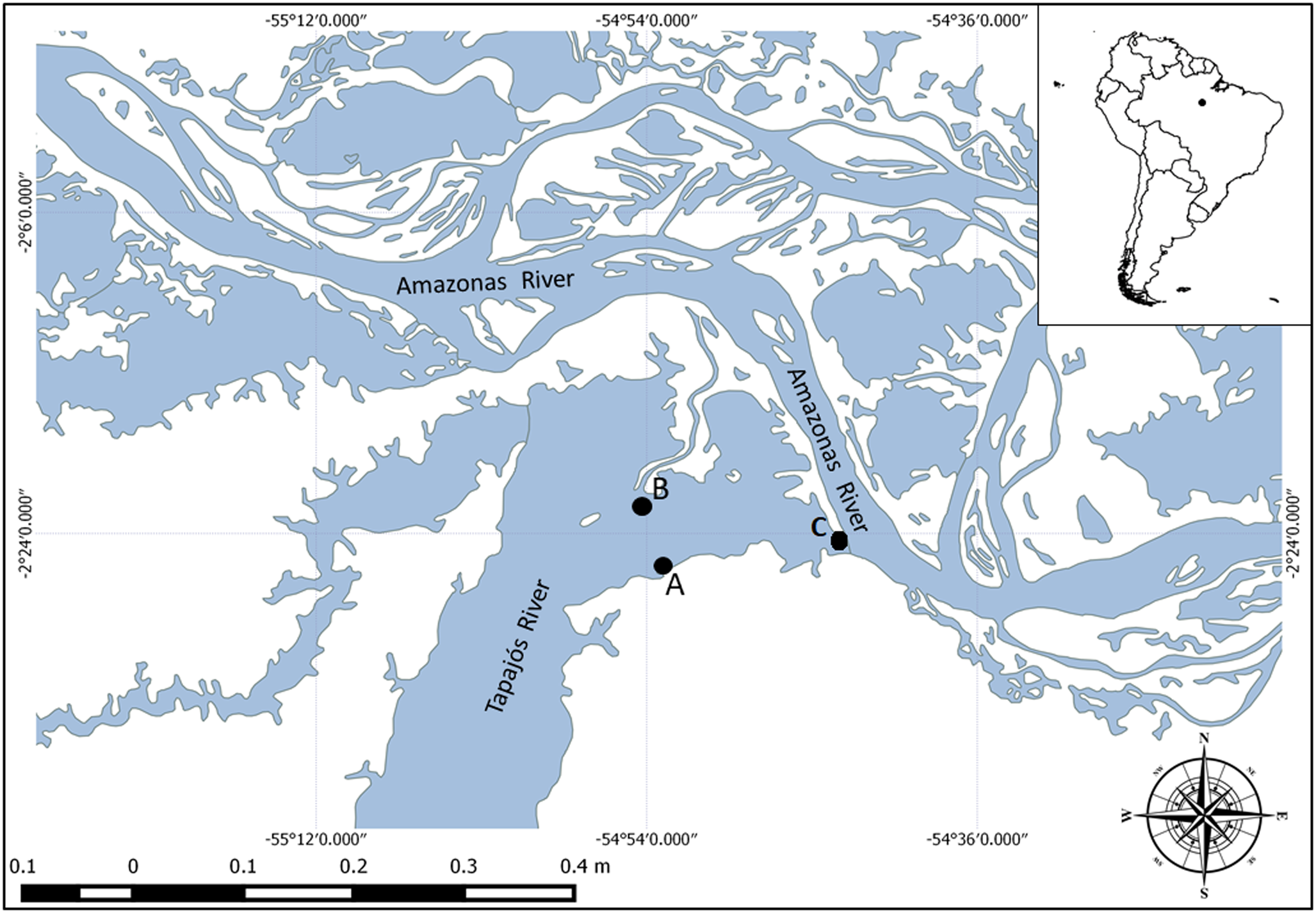
Fig. 1. Map of collection localities in the Amazon basin: Tapajós River, Pará State. (A) 2°26′23.30″S; 54°53′39,67″W; (B) 2°20′34.68″S; 54°53′27.69″W; and (C) 2°23′34.41″S; 54°43′57.53″W.
Nematode specimens (n = 39) found in the spiral intestine of the stingrays were fixed in 96% ethanols for light and scanning electron microscopy analyses and DNA sequencing.
The morphological analyses with light microscopy were based on three males and three females. Specimens were cleared with lactophenol on temporary slides and analysed in a V3 Leica Application Suite computerized system for image analysis with differential interference contrast. The range measurements (n = 6) are provided in micrometres unless otherwise specified. Holotypes and paratypes were deposited in the collection of the ‘Museu de Diversidade Biologica- MDBio’ (the Museum of Biological Diversity) of the Institute of Biology (IB) of the University of Campinas (UNICAMP), Campinas, São Paulo State, Brazil.
For scanning electron microscopy, one specimen of each sex was examined. Samples previously fixed in ethanol were transferred to a glutaraldehyde solution (2.5%) in 0.15 M phosphate buffer (pH 7.3) for 24 h, and then to an osmium tetroxide solution (1%) in the same buffer, for 2 h. The samples were washed in phosphate buffer, dehydrated in graduated ethanol (70, 80, 90, 95 and 100% at intervals of 15 min each), and finally in hexamethyldisilazane (HMDS) for around 5 min, in a methodology adapted from Bray et al. (Reference Bray, Bagu and Koegler1993). The dried samples were glued on a stub using double-sided tape, covered with gold (200 s/40 mA), and examined under a scanning electron microscope Leo Stereoscan S-440 of the São Paulo Federal University (UNIFESP), Campus Diadema.
Identification was based on the key to species of the Echinocephalus genus as proposed by Moravec & Justine (Reference Moravec and Justine2021) and Saad et al. (Reference Saad, Suthar, Theisen, Palm and Gargouri2022).
DNA extraction and amplification
The DNA analysis used nematode specimens and P. motoro muscle tissue (due to difficult morphological identification) previously fixed in ethanol. The worm specimens were cut on the medial section, the anterior portions were retained in absolute ethanol as hologenophores (see Pleijel et al., Reference Pleijel, Jondelius, Norlinder, Nygren, Oxelman, Schander, Sundberg and Thollesson2008) and deposited in the ‘Museu de Diversidade Biologica- MDBio’-IB/UNICAMP, while the posterior portions were used for DNA extraction. Nematode and stingray DNA extraction was performed with the Qiagen D Neasy® Blood & Tissue kit, in accordance with the manufacturer's instructions.
Nematode DNA samples were quantified and visualized on 2% agarose gel. SSU rDNA (800 base pairs (bp) ) and COI (780 bp) fragments were amplified using the Qiagen polymerase chain reaction (PCR) Master Mix kit. For SSU rDNA amplification the primers Nem18SF (5′ CGCGAATRGCTCATTACAACAGC-3′) and Nem18SR (5′-GGGCGGTATCTGATCGCC-3′) were used (Floyd et al., Reference Floyd, Rogers, Lambshead and Smith2005). The reactions in final volumes of 20 μl were constituted of: 7.7 μl of ultrapure water; 10 μl of Master Mix Qiagen 2X; 0.4 μl of Nem18SF (5 μM) primer; 0.4 μl of Nem18SR (5 μM) primer; and 1.5 μl of DNA template. The PCR conditions were 94°C/5 min., (94°C/30seg., 54°C/30seg., 72°C/60seg., repeated 35X) and 72°C/10 min. For COI amplification the primers amplification of the Cox1 gene the primers LCO1490 (5′-GGTCAACAAATCATAAAGATATTGG-3′) and HCO2198 (5′-TAAACTTCAGGGTGACCAAAAAATCA-3′) were used in accordance with Folmer et al. (Reference Folmer, Black, Wr, Lutz and Vrijenhoek1994), for reactions with a final volume of 20 μl, consisting of 7.5 μl ultrapure water, 10 μl of Master Mix Qiagen 2X, 0.4 μl of HCO2198 (5 μM), 0.4 μl of LCO1490 (5 μM), 1.5 μl of DNA and 0.2 μl of Taq DNA polymerase (5 U/μl) (Kappa Biosystems), and processed by the following cycling profile: (94°C/3 min., (94°C/50seg., 54°C/60seg., 72°C/60seg., repeated 35X), 72°C/5 min). The PCR products were visualized on 2% agarose gel for the confirmation of yield. Positive reactions were further purified using the 20% polyethylene glycol (PEG8000) protocol adapted from Dunn & Blattner (Reference Dunn and Blattner1987). Sequencing reactions were performed by the Sanger di-deoxiterminal method and were processed with ABI PRISM® Big Dye™ Terminator V.3 kit (Applied Biosystems™) following the manufacturer's instructions. Reactions were precipitated by the ethanol/ethylene diamine tetra acetic acid method and resuspended in Formamida Hi-Di for application of the ABI3500 genetic analyser (Applied Biosystems™). Sequences were assembled using Sequencher v.5.2.4 (Gene Codes, Ann Arbor, MI, USA) and submitted to GenBank. Fragments of the stingray COI mtDNA, used to confirm taxonomic status, were amplified and sequenced according to Ward et al. (Reference Ward, Zemlak, Innes, Last and Hebert2005).
Phylogenetic analysis and divergence time estimation
Two phylogenetic analyses were performed for the gnathostomatid studied herein, one using sequences of partial SSU rDNA and another the mitochondrial COI gene. The alignments were carried out using the sequences obtained in this study, plus that of Nematoda downloaded from GenBank (supplementary table S1). For COI data analyses the outgroup chosen were Necator americanus (AF303153) and Heligmosomoides polygyrus KJ994544. For SSU rDNA data analyses, the outgroup chosen was Tylocephalus auriculatus Bütschli, 1873 and Plectus aquatilis Andrássy, 1985 according to Nadler et al. (Reference Nadler, Carreno, Mejia-Madrid, Ullberg, Pagan, Houston and Hugot2007). Sequences representing different genes were separately aligned using the default parameters of the Muscle algorithm (Edgar, Reference Edgar2004) implemented in Geneious 7.1.3 (Kearse et al., Reference Kearse, Moir and Wilson2012). To evaluate the occurrence of substitution saturation, each aligned matrix was tested and the index of substitution saturation (ISS) was estimated using DAMBE 5 software (Xia, Reference Xia2013). The number of base substitutions per site between the sequences was calculated. Standard error estimates were obtained using a bootstrap procedure with 2000 replicates. Analyses were conducted using the Kimura 2-parameter model using MEGA5 software (Kimura, Reference Kimura1980; Tamura et al., Reference Tamura, Stecher, Peterson, Filipski and Kumar2013).
The best-fit model for nucleotide evolution in the resulting matrices was determined using the Akaike information criterion in the jModelTest software package (Posada, Reference Posada2008). Phylogenetic analyses were performed using Bayesian inference (BI) and maximum likelihood (ML) inference methods. The BI analyses were implemented in MrBayes 3.2 (Ronquist & Huelsenbeck, Reference Ronquist and Huelsenbeck2003). The model indicated by JModelTest for the COI gene dataset was GTR + I + G. For the SSU gene dataset it was TIM2 + I + G. As this is not implemented in MrBayes 3.2, it was replaced by the closest over-parameterized model available, which was GTR + I + G. BI were run using the following nucleotide substitution model settings for both datasets: lset nst = 6; rates = invariable; ncat = 4; shape = estimate; inferrates = yes; and basefreq = empirical. For the Markov chain Monte Carlo search, chains were run with 10,000,000 generations, saving one tree every 1500 generations. On the burn-in, the first 25% of generations were discarded and the consensus tree (majority rule) was estimated using the remaining topologies; only nodes with posterior probabilities greater than 90% were considered well supported. ML were carried out using RAxML (Guindon & Gascuel, Reference Guindon and Gascuel2003) with bootstrap support values of 1000 repetitions, and only nodes with bootstrap values greater than 70% were considered well supported. The trees were visualized using FigTree v.1.3.1 (Rambaut, Reference Rambaut2009).
For divergence time estimation, a new alignment considering only the SSU rDNA sequences of nematode species were used in BI analysis. For calibrating the molecular clock, the sequence of Oxyxiuris equi (Schrank, 17188) (EF180062) and Dentostomella sp. (AF036590) were used as the representative species of the Order Oxyurida, which has a fossil record for the species Paleoxyuris cockburni (Hugot et al., Reference Hugot, Gardner, Borba, Araujo, Leles, Rosa, Dutra, Ferreira and Araujo2014). Plectus aquatilis (GQ892827) and Tylocephalus auriculatus (AF202155) were used as an external group.
The analysis was performed using the BEAST v2.4.3 software package (Bouckaert et al., Reference Bouckaert, Heled, Kühnert, Vaughan, Wu, Xie, Suchard, Rambaut and Drummond2014), with the species tree inference method and a relaxed lognormal clock, set to the Yule process option (Drummond et al., Reference Drummond, Ho, Phillips and Rambaut2006). Two runs of 100 million chains were carried out and the quality of the convergences was verified using the Tracer 1.7 software package (Rambaut et al., Reference Rambaut, Drummond, Xie, Baele and Suchard2018). Tracing qualities were considered when effective sample size values above 200,000 were observed. The extracts resulting from the trees were created in TreeAnnotator 2.3.1 (Bouckaert et al., Reference Bouckaert, Heled, Kühnert, Vaughan, Wu, Xie, Suchard, Rambaut and Drummond2014) with a burn-in of 10% of the total number of trees generated.
Phylogenetics and divergence time estimation trees generated in this study were visualized in FigTree version 1.3.1 (Rambaut, Reference Rambaut2020).
Results
Fourteen of the 35 examined stingrays, 20 females and 15 males, measuring approximately 31.5 cm (ranging from 17–54 cm) in length and 29.5 cm (ranging from 19–49 cm) in width and (40%) had from 1 to 23 gnathostomatid nematodes in their spiral intestine. Morphological and molecular analyses revealed these to be adult specimens of an undescribed Echinocephalus species. Morphological analysis and DNA based phylogenetic and dating inferences are presented below (figs 2–7).
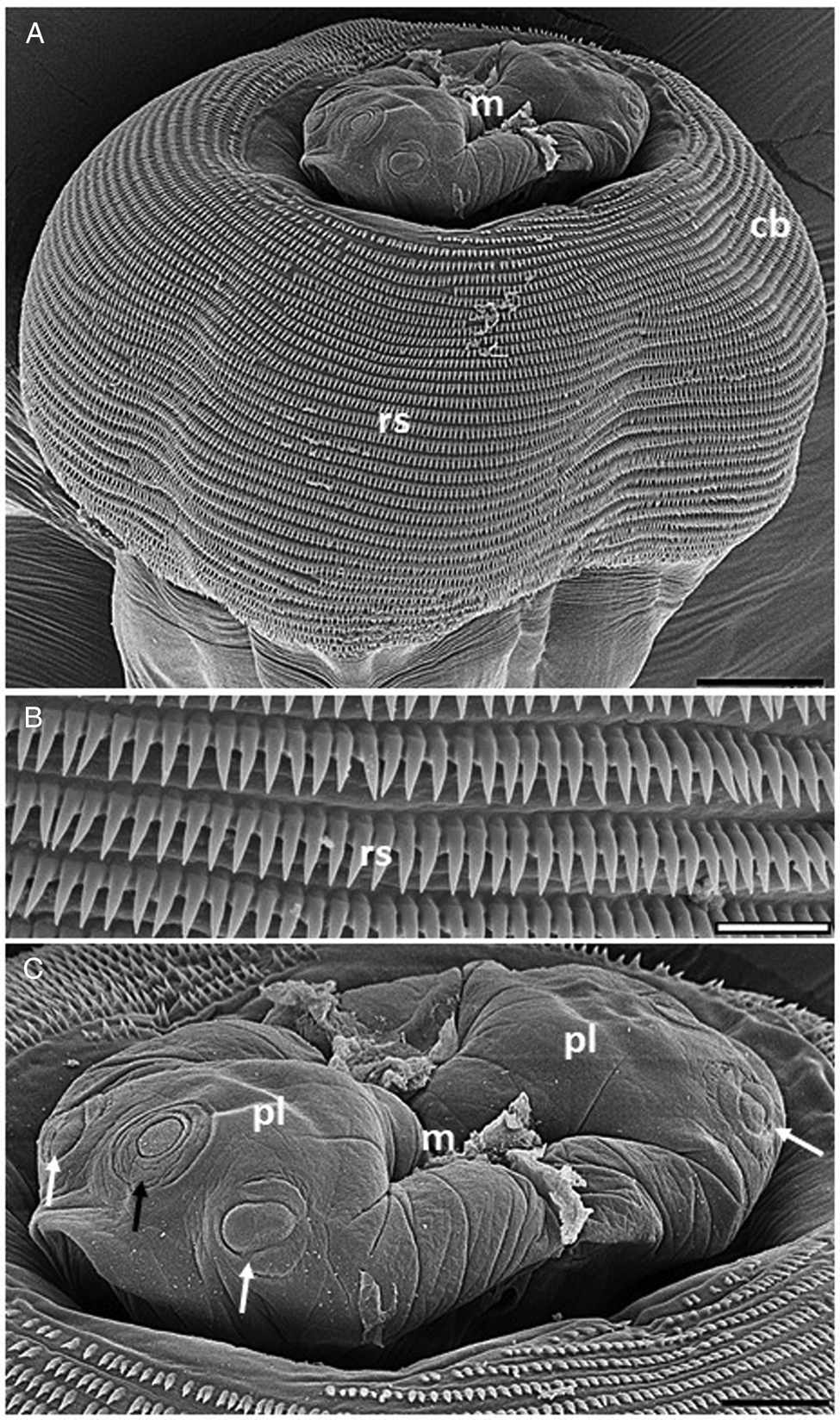
Fig. 2. Electron micrographs of Echinocephalus spinosus n. sp. parasite of spiral intestine of Potamotrygon motoro: (A) male anterior region showing cephalic bulb (cb) with its rows of cephalic spines (rs) and mouth region (m). Scale bar = 50 μm; (B) details of disposition of the cephalic spines. Scale bar = 10 μm; And (C) details of the mouth region (m) showing pseudolabium (pl) with sub-median papillae (white arrows) and amphid (black arrow). Scale bar = 20 μm.
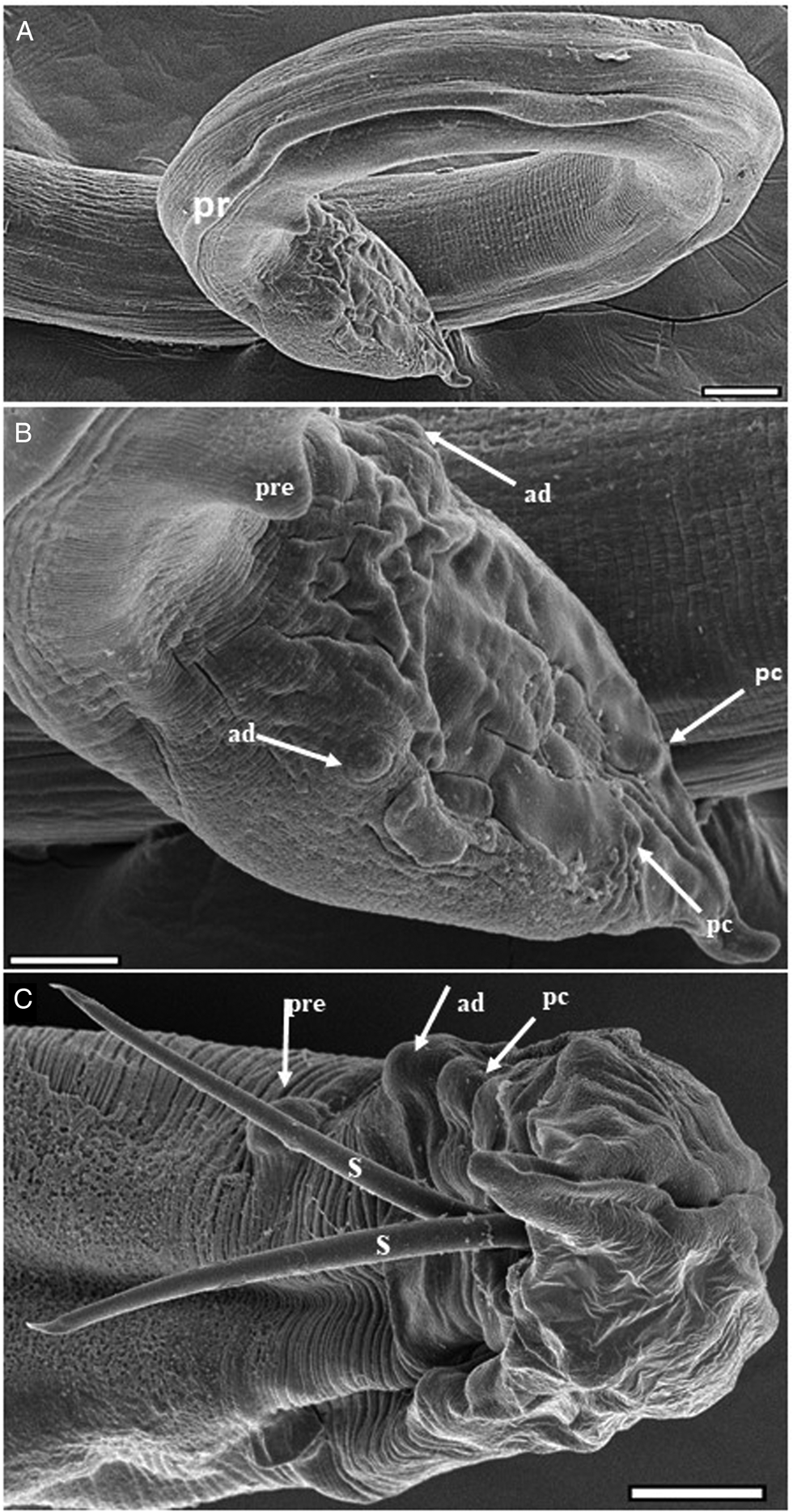
Fig. 3. Electron micrographs of Echinocephalus spinosus n. sp. parasite of spiral intestine of Potamotrygon motoro: (A–C) male posterior region (pr) with (B) showing details of precloacal (pre), adcloacal (ad), and postcloacal (pc) pappilae, and the two externalized spicules (s). Scale bars: A = 100 μm, (B, C) = 50 μm.
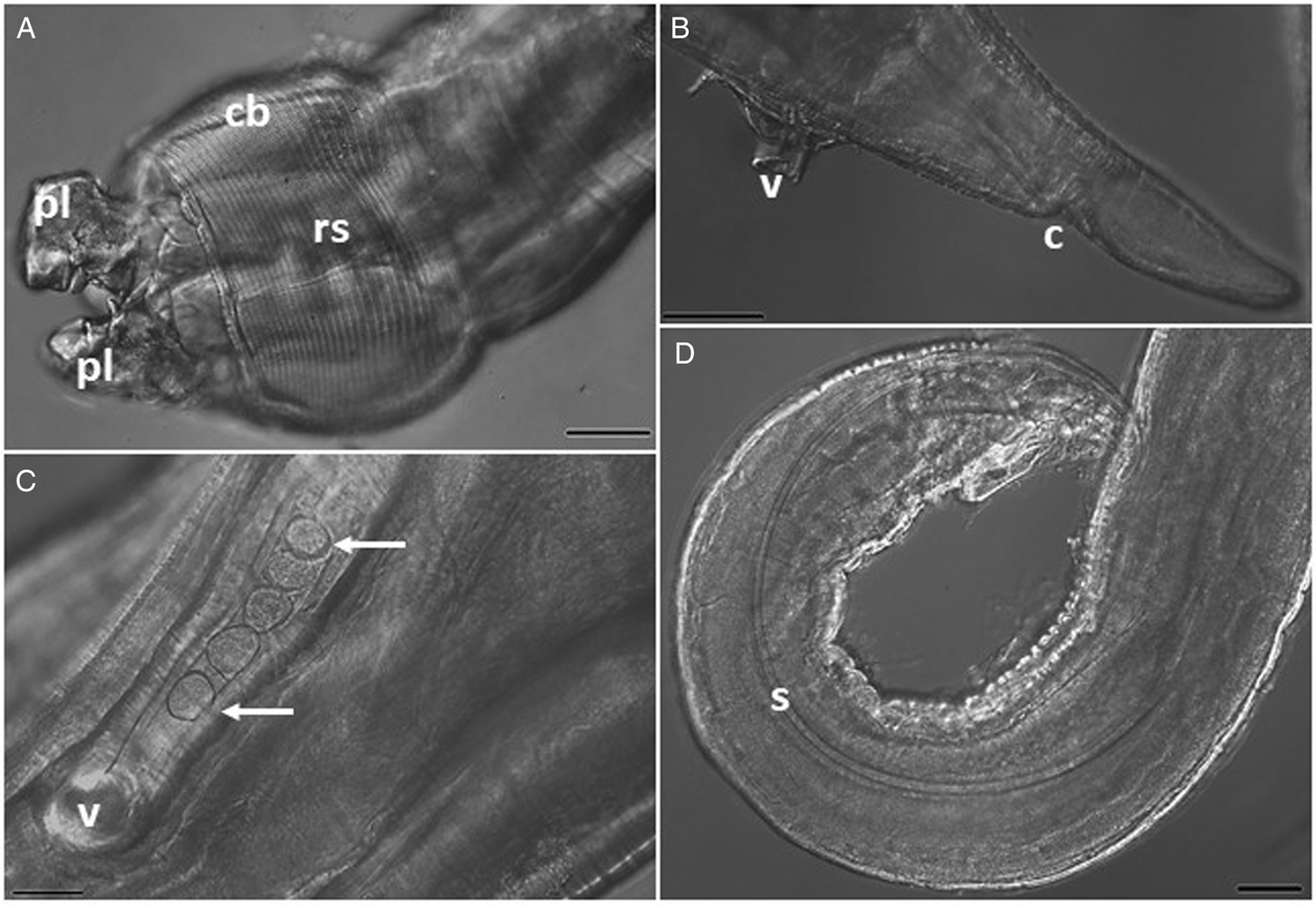
Fig. 4. Photomicrographs of Echinocephalus spinosus n. sp. parasite of spiral intestine of Potamotrygon motoro: (A) male anterior region showing pseudolabium (pl) and cephalic bulb (cb) with its rows of cephalic spines (rs); (B) and (C) female posterior region showing vulva (v) and anus (c) and eggs (white arrows); and (D) male posterior region showing one of the spicules (s). Scale bars: (A, D) = 100 μm, (B) = 200 μm, (C) = 50 μm.

Fig. 5. Schematic drawing of Echinocephalus spinosus. n. sp. parasite of the spiral intestine of Potamotrygon motoro: (A) ventral view of the anterior region showing cephalic bulb and cervical zone of male. Note the muscular and glandular esophagus and cervical sacs; (B) pattern and structure of spines in the middle region of the cephalic bulb; (C) lateral view of the male caudal end; (D) spicule with hyaline tips; (E) egg; and (F) lateral view of female posterior extremity showing the vulva.
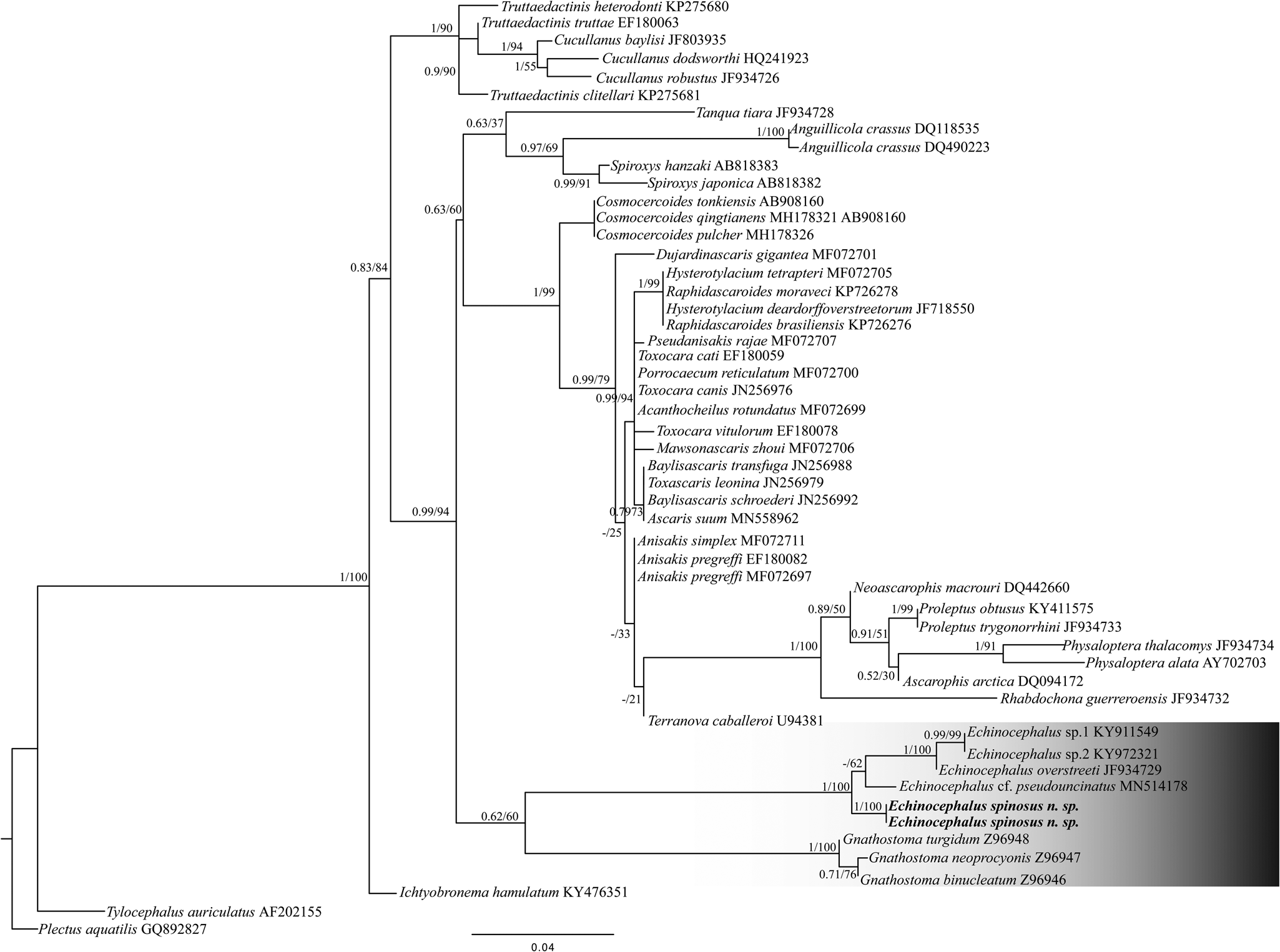
Fig. 6. Maximum likelihood (ML) topology based on partial small subunit ribosomal ribonucleic acid sequences of Nematoda (Spirurina). GenBank accession numbers are indicated next to species names. Numbers above nodes represent supported nodes by posterior probabilities for Bayesian analyses and bootstrap for ML analyses, respectively (posterior probabilities >0.90 and bootstrap scores >70). Branch length scale bar indicates number of substitutions per site.
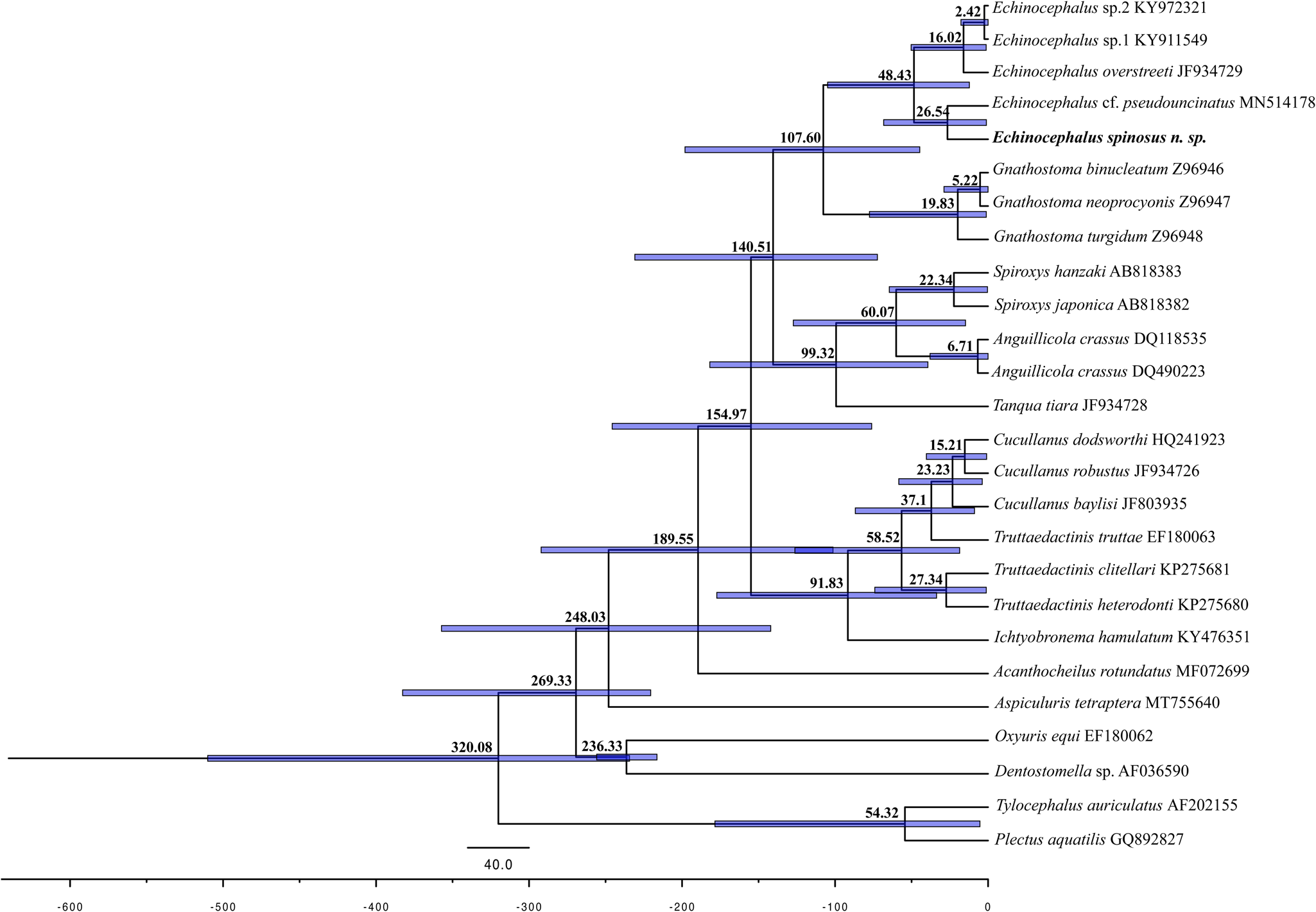
Fig. 7. Estimated divergence time for nematode species. The blue bars in the nodes indicate 95% higher posterior density (HPD) of the posterior Bayesian distribution of molecular time estimates. The geological time scale is in accordance with the International Chronostratigraphic Chart (http://www.stratigraphy.org, v2020/01).
Taxonomic summary
Phylum: Nematoda Diesing, 1861.
Family: Gnathostomatidae Railliet, 1895.
Genus: Echinocephalus Molin, 1858.
Echinocephalus spinosus. n. sp. (figs 2–5)
Host type: Potamotrygon motoro Müller & Henle 1841 (the identification of the host was based on morphology and corroborated by sequencing of the mitochondrial gene COI – GenBank accession number OP246209).
Locality: Tapajós River, municipality of Santarém, state of Pará, Brazil (geographical coordinates 2°26′23.30″S; 54°53′39,67″W, 2°20′34.68″S; 54°53′27.69″W, and 2°23′34.41″S; 54°43′57.53″W).
Types: Deposited in the ‘Museu de Diversidade Biologica- MDBio’-IB/UNICAMP: Holotype (male ZUEC NMA 31); Alotype (female ZUEC NMA 32); Paratypes (ZUEC NMA 33 – males and females).
Hologenophores, Deposited in the ‘Museu de Diversidade Biologica- MDBio’-IB/UNICAMP, under the section number ZUEC NMA 34.
Site of infection: spiral valve–intestines.
Etymology: The Latin epithet spinosus is used as the specific name, based on the large number of rows of cephalic spines observed in this species.
Representative DNA sequences: Two sequences of partial SSU rDNA (GenBank accession number OP256110 and OP256156 and four sequences of COI mtDNA (GenBank accession number OP256158, OP256159, OP281694, and OP270220.
ZooBank registration: The Life Science Identifier for E. spinosus n. sp. is lsid:zoobank.org:pub:62FA0A09-87C8-45AC-A09F-0A55A5B436C7.
Description
General: Large nematodes with transversely striated cuticle, with rough posterior area for males (figs 2A, B, 3A and 5). Cephalic end with two large lateral pseudolabia with trilobed anterior portions (fig. 2A, C); each lobe carries two cuticular thickenings along the outer edges, which intertwine with those of the opposite pseudolabium; medial portion of each pseudolabium elongated dorsoventrally, with a pair of sub-median papillae lateral and an amphids between them in the posterior region of the lobes (fig. 2C). Small triangular interlabia (one dorsal and one ventral) present between the pseudolabia. Posterodorsal and posteroventral region of each pseudolabium and interlabia lack cuticular serrations. Prominent cephalic bulb armed with 38–40 transverse rows of small, elongated spines; rows of spines close together but not overlapping; anterior and posterior rows composed of smaller spines than those in the middle portion (fig. 2A, B). Long oesophagus, 17–20% of body length, wider near its posterior end. Four cervical sacs present, extending posteriorly to about one to two-thirds of the length of the oesophagus. Well-developed deirids located symmetrically, immediately posterior the level of the nerve ring.
Male (holotype) (based on five whole specimens and one tail fragment): Body 55–66 mm long by 0.6–0.9 mm maximum wide. Pseudolabia 154–184 long and 278–309 wide. Cephalic bulb 438–525 long and 605–646 wide, with 38–40 rows of cephalic spines. Nerve ring 0.8–1.0 mm from the anterior end, with 24–43 wide. Cervical sac 1.5–2.2 mm in length and 78–173 in maximum width. Oesophagus 6 to 11% of total body length, 3.6–6.2 mm in length; muscular oesophagus 1.6–3.4 mm in length, by 283–362 in width; glandular oesophagus 2.0–3.2 mm in length, by 378–535 in width. Similar spicules, equal in length, 2.7–3.6% of total body length, 1.3–1.4 mm long by 0.37 mm wide (figs 2, 3 and 5). V-shaped gubernaculum, 77.8 long. Caudal alae curved ventrally, surrounding the cloaca, 45.3 long, supporting nine pairs of caudal papillae; three precloacal pairs of unequal length, with the median pair larger and more lateral; one pair adcloacal; six postcloacal pairs, unequal in length, with the first pair extremely small at the extremity, third pair more lateral and largest, and sixth pair immediately posterior to the cloaca. Phasmids paired near tail end. Modified annulus on the ventral surface near the caudal wing, extending anteriorly. Tail 260 long (figs 3 and 5).
Female (holotype) (based on five whole specimens and two fragmented tails): Body 55–85 mm long by 0.9–1.4 mm maximum wide. Pseudolabia 154–236 in length by 296–378 in width. Cephalic bulb 475–677 long by 587–803 wide, with 38–40 rows of spines. Nerve ring 0.7–1.2 mm from the anterior end, with 37–78 in width. Cervical sacs 1.9–2.8 mm in length by 60–267 in width. Oesophagus 7–11% of total body length, 5.4–6.6 mm in length; oesophageal muscle 2.8–3.6 mm in length by 166–394 in width; glandular oesophagus 2.3–3.4 mm in length by 315–551 in width. Vulva opening 779 and 1088 from the rear end. Didelphic uterus, prodelphic. Eggs with thin, smooth shell, and oval, with 38.1–41.2 long and 32.5–34.1 wide. Tail 365.3 long (figs 2, 4 and 5).
Molecular analyses and phylogenetic study
The rDNA sequencing of specimens of E. spinosus. n. sp. resulted in representative DNA sequences: two sequences of partial SSU rDNA, 465 and 555 bp-long; and four sequences of COI mtDNA, 638, 654, 552, and 616 bp-long. The Basic Local Alignment Search Tool search performed with these sequences did not match any other nematode available in GenBank. Genetic divergence analysis based on SSU rDNA sequences ranged from 1% to 18%, and the divergence among Echinocephalus species varied from 1% to 3% (supplementary table S2). When the COI was used, the genetic divergence varied from 1% to 59%. The divergences between specimens of E. spinosus. n. sp. and the gnathostomatids Gnathostoma spinigerum AB037132 and Gnathostoma binucleatum AB037131 were 20% to 23%, and 1% among specimens of E. spinosus n. sp. (supplementary table S3).
The analysis of the alignment of the SSU rDNA sequences comprising 471 bp characters and 26 sequences (supplementary table S1) in the DAMBE revealed lower ISS values than critical Iss assuming a symmetrical topology (Iss.cAsym) and no saturation in either transitions and transversions in both asymmetrical (Iss.cSym) values, revealing the lack of a saturation signal in the matrix. Both BI and ML phylogenetic inferences converged in similar topologies and recovered the Echinocephalus lineage as the sister of Gnathostoma. In the Echinocephalus lineage, the sequence of E. spinosus. n. sp. arises as a sister of that of Echinocephalus cf. pseudouncinatus (MN514178), which was obtained from larvae stage parasitizing the mollusc Atrina maura from Mexico (Karagiorchis et al., Reference Karagiorchis, Ploeg and Ghafar2022) (fig. 6). The divergence time analysis revealed that E. spinosus n. sp. diverged from its ancestor at around 26.54 Ma, with a confidence interval of 31.54–21.54 Ma (fig. 7).
Discussion
Within the gnathostomatids, as far as we know, the occurrence of adult stages of species of the genus Echinocephalus are restricted to elasmobranch hosts, mainly rays/stingrays (Moravec & Justine, Reference Moravec and Justine2021). Only E. diazi and E. daileyi have so far been described in freshwater hosts, both from potamotrygonin stingrays from the north of South America. However, there is a lack of consensus over the real freshwater occurrence of E. diazi, as it was initially described from a species identified as P. hystrix, caught in the estuarine Lake Maracaibo (Troncy, Reference Troncy1970), and subsequently twice reported infecting the marine ray S. schmardae (Deardorff et al., Reference Deardorff, Brooks and Thorson1981), a myliobatiform now recognized in the subfamily Styracurinae in Potamotrygonidae (Carvalho et al., Reference Carvalho, Loboda and Silva2016). This suggests that E. diazi is a marine, or at least, marine/brackish organism. Thus, Deardorff et al. (Reference Deardorff, Brooks and Thorson1981), when describing E. daileyi from the Amazon and Orinoco River basins, attested to it being the first member of the genus Echinocephalus reported to infect stingrays inhabiting a freshwater environment, sensu stricto. Following this conception, E. spinosus n. sp., now described infecting P. motoro from the Tapajós River, is only the second Echinocephalus species found parasitizing a stingray habiting a sensu stricto freshwater environment, also in the north of South America.
Based on the keys proposed by Moravec & Justine (Reference Moravec and Justine2021) and Saad et al. (Reference Saad, Suthar, Theisen, Palm and Gargouri2022), E. spinosus n. sp. and E. daileyi differ in the number of transverse rows of spines in the cephalic bulb, with 30–32 for E. daileyi, and 38–40 for the new species, and in spicule length, with 1.4–2.4 mm in E. daileyi and 1.3–1.9 mm in E. spinosus n. sp. When compared to E. diazi, E. spinosus n. sp. differs in the number of transverse rows of spines in the cephalic bulb (respectively, 25 and 38–40 rows), in having shorter spicules (respectively, 2 and 1.3–1.9 mm), and in number of pairs of preanal papillae (two and three, respectively). Regarding the species described infecting marine hosts, besides of the difference of environment (marine vs. freshwater), they differ from E. spinosus n. sp. by the number of transverse rows of spines in the cephalic bulb, length of the spicules or number of pairs of caudal papillae (Moravec & Justine, Reference Moravec and Justine2021; Saad et al., Reference Saad, Suthar, Theisen, Palm and Gargouri2022).
Accurate identification of species is essential for studies of diversity (Blasco-Costa et al., Reference Blasco-Costa, Faltynkova, Georgieva, Skrnisson, Scholz and Kostadinova2014), and molecular analyses can provide important insights in this area. Thus, in addition to morphology, we also provided molecular analysis based on SSU rDNA (fig. 6) and COI (supplementary fig. S1, supplementary table S3) sequences of E. spinosus n. sp. These data showed that the interspecific nucleotide variation between species of Echinocephalus varied from 1% to 3% for the SSU rDNA (supplementary table S2). Unfortunately, there were no Echinocephalus spp. sequences available for the COI gene to compare our data to (supplementary fig. S1). Previous molecular phylogenetic studies using the SSU ribosomal gene have demonstrated a high degree of sequence similarity among a subset of the Echinocephalus species (van Megen et al., Reference Van Megen, Van den Elsen, Holterman, Karssen, Mooyman, Bongers, Holovachov, Bakker and Helder2009; Abdel-Ghaffar et al., Reference Abdel-Ghaffar, Bashtar, Mehlhorn, Abdel-Gaber, Al Quraishy and Saleh2013). Abdel-Ghaffar et al. (Reference Abdel-Ghaffar, Bashtar, Mehlhorn, Abdel-Gaber, Al Quraishy and Saleh2013) used the portion of the SSU gene + internal transcribed spacer (ITS) and verified Echinocephalus carpiae Abdel-Ghaffar et al., Reference Abdel-Ghaffar, Bashtar, Mehlhorn, Abdel-Gaber, Al Quraishy and Saleh2013), described from larvae obtained from Cyprinus carpio from Egypt, had divergence of 7% to E. overstreeti. Later, based on SSU rDNA Karagiorchis et al. (Reference Karagiorchis, Ploeg and Ghafar2022) demonstrated that the difference was of only 3.1%, and proposed E. carpiae as a junior synonym of E. overstreeti. As expected, the SSU rDNA gene is more conserved than the ITS region, and presented lower divergence, being robust for the separation of species, and several authors have used it to distinguish species and infer phylogenies of nematodes (Dare et al., Reference Dare, Nadler and Forbes2008; Dubey & Shine, Reference Dubey and Shine2008; Langford & Janovy, Reference Langford and Janovy2013; Karagiorchis et al., Reference Karagiorchis, Ploeg and Ghafar2022).
Phylogenetic analyses carried out by Laetsch et al. (Reference Laetsch, Heitlinger, Taraschewski, Nadler and Blaxter2012) showed E. overstreeti emerging as a sister lineage of Anguillicola spp. (Anguillicolidae). Nonetheless, when adding four SSU rDNA sequences of Echinocephalus species downloaded from GenBank, plus that provided in the present study, our analyses recovered a lineage of gnathostomatids with Echinocephalus species arising as a sister of Gnathostoma spp. However, another gnathostomatid, Tanqua tiara von Linstow, 1879, appears as a sister species of the lineage composed of the Anguillicola and Spiroxys species (Anguillicoliidae), corroborating the absence of the monophyly of gnathostomatids observed by Laetsch et al. (Reference Laetsch, Heitlinger, Taraschewski, Nadler and Blaxter2012) (fig. 6). This profile seems to be reflected in the radiation of these nematode lineages into distinct groups of definitive hosts –that is, mammals (Gnathostoma), squamates (Tanqua), and marine and freshwater elasmobranchs (Echinocephalus), as well as the diversity of their intermediate and paratenic hosts (Laetsch et al., Reference Laetsch, Heitlinger, Taraschewski, Nadler and Blaxter2012).
Our phylogeny using COI also recovered E. spinosus n. sp. and Gnathostoma spp. as being closely related, but without good resolution and bootstrap and posterior probability support (supplementary fig. S1). These results may be related to the high genetic divergence of this marker (supplementary table S3) and the lack of other related sequences, specially of the gnathotomatid genera available in the GenBank database. As proposed by Laetsch et al. (Reference Laetsch, Heitlinger, Taraschewski, Nadler and Blaxter2012), future studies providing new COI sequences of gnathostomatids and of other related nematodes may bring further insights into this marker in the study of its evolution.
To the best of our knowledge, there are no reports of freshwater Echinocephalus species in other continents. Despite this being only the second description of an Echinocephalus species parasitizing Neotropical potamotrygonins (the first was E. daileyi), it can be hypothesized that the radiation of this gnathostomatid lineage in South America followed sensu stricto freshwater stingray. This hypothesis is endorsed by the report of Echinocephalus sp., not identified at a species taxonomic level, parasitizing the largespot river stingray P. falkneri from the upper Paraná River basin, in the south of South America (Lacerda et al., Reference Lacerda, Takemoto and Pavanelli2009).
Compared to bony fishes, which have comparable species richness in marine and freshwater habitats (Levêque et al., Reference Levêque, Oberdorff, Paugy, Stiassny and Tedesco2008), few cartilaginous fish species – mainly freshwater stingrays – were able to colonize permanently freshwater environments (Nelson et al., Reference Nelson, Grande and Wilson2016). According to Kirchhoff et al. (Reference Kirchhoff, Hauffe, Stelbrink, Albrecht and Wilke2017), stingrays colonized freshwater environments independently at least four times, and marine incursions had a pivotal role in their adaptation to this habitat. Neotropical potamotrygonins, meanwhile, are exclusively freshwater stingray, occurring in the main South American watersheds, with the greatest diversity in the Amazon (Carvalho et al., Reference Carvalho, Lovejoy, Rosa, Reis, Kullander and Ferraris2003; Silva & Loboda, Reference Silva and Loboda2019; Fontenelle et al., Reference Fontenelle, Marques, Kolmann and Lovejoy2021; Loboda et al., Reference Loboda, Lasso, Rosa and Carvalho2021).
During its geological history, South America has been the setting for several marine incursions (Räsänen et al., Reference Räsänen, Linna, Santos and Negri1995; Lundberg et al., Reference Lundberg, Marshall, Guerrero, Horton, Malabarba and Wesselingh1998), which were the scenario for the emergence of organisms of marine derived lineages, now endemic to freshwater habitats of this continent (i.e. anchovies, drum fish, stingrays, dolphins, manatees, sponges, crustaceans, molluscs and parasites) (Nuttall, Reference Nuttall1990; Cassens et al., Reference Cassens, Vicario and Waddell2000; Hamilton et al., Reference Hamilton, Caballero, Collins and Brownell2001; Boeger & Kritsky, Reference Boeger and Kritsky2003; Bloom & Lovejoy, Reference Bloom and Lovejoy2017; Cavalcanti et al., Reference Cavalcanti, Santos-Silva and Primeiro2019; Adriano et al., Reference Adriano, Zatti and Okamura2021; Oliveira et al., Reference Oliveira, Corrêa, Adriano and Tavares-Dias2021; Zatti et al., Reference Zatti, Adriano, Araújo, Franzolin and Maia2022). In this context of marine derived lineages, several studies have hypothesized the emergence of South American stingrays (Lovejoy et al., Reference Lovejoy, Bermingham and Martin1998, Reference Lovejoy, Albert and Crampton2006; Carvalho et al., Reference Carvalho, Maisey and Grande2004; Adnet et al., Reference Adnet, Salas Gismondi and Antoine2014; Bloom & Lovejoy, Reference Bloom and Lovejoy2017; Kirchhoff et al., Reference Kirchhoff, Hauffe, Stelbrink, Albrecht and Wilke2017). Fontenelle et al. (Reference Fontenelle, Marques, Kolmann and Lovejoy2021) generated a time-calibrated phylogeny including 35 Neotropical freshwater stingrays and showed that the divergence between the freshwater potamotrygonins and the marine Styracurinae was estimated at 26.4 Ma (ranging 32.1–20.6 Ma). These data place the origin of the potamotrygonins into the late Oligocene to early Miocene, when the western Amazon was dominated by the Pebas wetlands – an epicontinental marine/freshwater system covering more than one million km2, from where the diversification of potamotrygonins would have occurred (Fontenelle et al., Reference Fontenelle, Marques, Kolmann and Lovejoy2021). This time estimation is corroborated by the recent find of stingray teeth fossils from the Oligocene–Miocene deposits, from Contamana, Peru (Chabain et al., Reference Chabain, Antoine, Altamirano-Sierra, Marivaux, Pujos, Gismondi and Adnet2017), the age of which matches the molecular results presented by Fontenelle et al. (Reference Fontenelle, Marques, Kolmann and Lovejoy2021).
Corroborating the late Oligocene to early Miocene origin of potamotrygonins, our time-calibrated phylogenetic analysis revealed the divergence between the freshwater E. spinosus n. sp. and its ancestor to be 26.5 Ma (ranging 31.5–21.5 Ma), placing the freshwater Echinocephalus lineage origin into the same geological scenario revealed by Fontenelle et al. (Reference Fontenelle, Marques, Kolmann and Lovejoy2021) for potamotrygonins. Associated with the results of Chabain et al. (Reference Chabain, Antoine, Altamirano-Sierra, Marivaux, Pujos, Gismondi and Adnet2017) and Fontenelle et al. (Reference Fontenelle, Marques, Kolmann and Lovejoy2021), our data indicate that the Pebas wetlands seems to have provided adequate conditions for the transitions from marine to freshwater life of this host–parasite complex. Later, the co-radiation of stingrays/Echinocephalus occurred in the continent. Studies on gnathostomatid parasites of South American freshwater stingrays from different watersheds could result in fascinating new insights into the co-radiation/co-speciation of this host–parasite complex in the context of the geological history of South America.
In conclusion, the present study demonstrates that combining morphological-light and scanning electron microscopy, and DNA based phylogenetic analyses are suitable for the diagnosis of gnathostomatids. The time-calibrated phylogeny analysis provided support for a scenario of the co-emergence of freshwater potamotrygonins/Echinocephalus during a geological time that corresponds to the period of occurrence of the Pebas wetlands in the western Amazon.
Associated with recent biogeographical studies into potamotrygonins, the discovery of new sensu stricto freshwater Echinocephalus species suggests that the origin of the freshwater potamotrygonins/Echinocephalus was followed by the co-radiation of this host–parasite complex across the continent, opening avenues for further evolutionary studies involving this fascinating and complex host–parasite–geological history.
Authors’ contributions
M.I.M. and L.L.C, conceived the initial idea for the study and undertook sampling, performed morphological, and molecular analysis . M.I.M., M.S.B.O. and E.A.A., performed phylogenetic analyses and time estimation . M.I.M. and L.L.C., participated in the writing of the draft manuscript confection. Project administration, supervision, funding acquisition, revision and the organization of the manuscript was conducted by E.A.A. All authors read the manuscript and gave final approval for publication.
Supplementary material
To view supplementary material for this article, please visit https://doi.org/10.1017/S0022149X22000554.
Acknowledgement
We thank Dr Nadayca Mateussi for help identifying the fish host.
Financial support
This study was partially supported by the regular project grants from São Paulo Research Foundation (FAPESP) (grants # 2018/24980-8 and 2019/17427-3) and by the Coordination for the Improvement of Higher Education Personnel (CAPES), Brazil, Finance Code 001. M. I. Muller was supported by a postdoctoral scholarship from the São Paulo Research Foundation (FAPESP) (grants # 2017/16546–3 and 2021/06692-8). E. A. Adriano received a research productivity grant from the Brazilian Fostering Agency CNPq (grant # 304687/2020-0).
Conflicts of interest
None.
Ethical standards
None.