1. Introduction
Granulite-facies rocks contain a unique record of lithosphere-scale structural and metamorphic history, and granulite terranes reflect major tectonic processes in the deep Earth (Green & Ringwood, Reference Green and Ringwood1967; Bohlen, Reference Bohlen1987; Ellis, Reference Ellis1987; Harley, Reference Harley2008). Granulite-facies rocks and migmatite production are associated with extensional regimes (Pin & Vielzeuf, Reference Pin and Vielzeuf1983; Lardeaux & Spalla, Reference Lardeaux and Spalla1991; Marschall, Kalt & Hanel, Reference Marschall, Kalt and Hanel2003; Schuster & Stüwe, Reference Schuster and Stüwe2008) as well as with compressional regimes, where they are likely related to crustal shortening, high isotopic heat production and coeval erosional unroofing (Gruf Complex, Central Alps: Galli et al. Reference Galli, Le Bayon, Schmidt, Burg, Reusser, Sergeev and Larionov2012; e.g. Himalaya: Searle et al. Reference Searle, Cottle, Streule and Waters2010).
A Carboniferous amphibolite to granulite facies evolution is well established in the Variscan belt (Pin & Vielzeuf, Reference Pin and Vielzeuf1983, Reference Pin and Vielzeuf1988; Marschall, Kalt & Hanel, Reference Marschall, Kalt and Hanel2003). Slices of these Variscan belt granulites have been incorporated in the Alpine chain within the external or axial units (von Raumer, Stampfli & Bussy, Reference von Raumer, Stampfli and Bussy2003; Marotta & Spalla, Reference Marotta and Spalla2007; Schuster & Stüwe, Reference Schuster and Stüwe2008). Within the internal units of the Alpine chain, Permian–Triassic magmatism and amphibolite–granulite metamorphism have also been described (Marotta & Spalla, Reference Marotta and Spalla2007). There are two main groups of interpretations of this post-Carboniferous thermal anomaly: (i) as an effect of the late orogenic collapse of the Variscan belt (Malavielle et al. Reference Malavielle, Guihot, Costa, Lardeaux and Gardien1990; Ledru et al. Reference Ledru, Courrioux, Dallain, Lardeaux, Montel, Vanderhaeghe and Vitel2001) and (ii) as a consequence of lithospheric thinning and asthenosphere upwelling that led to continental rifting (Lardeaux & Spalla, Reference Lardeaux and Spalla1991; Diella, Spalla & Tunesi, Reference Diella, Spalla and Tunesi1992; Müntener & Hermann, Reference Müntener, Hermann, Wilson, Whitmarsh, Taylor and Froitzheim2001; Schuster & Stüwe, Reference Schuster and Stüwe2008; Marotta, Spalla & Gosso, Reference Marotta, Spalla, Gosso, Ring and Wernicke2009).
The aim of the present study is to reconstruct the pre-Alpine tectonometamorphic evolution of the Valpelline unit (Dent Blanche nappe, Western Italian Austroalpine Domain) pre-dating the beginning of the Alpine convergence. The Valpelline unit represents one of these Variscan slices incorporated into the Alpine chain. Rocks from a small side canyon of Valpelline Valley have been selected for this purpose because the pre-Alpine structures and assemblages are well preserved as a result of the poor Alpine deformation affecting this part of the Valpelline unit. Pressure–temperature–time–deformation (P–T–t–d) paths have been derived by combining the sequence of deformational stages with the successive metamorphic imprints. Our results suggest a tectonometamorphic evolution of the Valpelline unit that differs from that described in previous studies.
2. Geological setting
The Alps are a double vergent orogen. They formed since the Cretaceous period as a consequence of the convergence between Europe and the Adria-Africa plates and the closure of an oceanic basin, the Tethys, that was interposed between the two continental passive margins (Malusà et al. Reference Malusà, Faccenna, Garzanti and Polino2011). The axial part of the Alps is formed by fragments of continental- and ocean-derived rocks, which suffered, from the Cretaceous to Oligocene periods, important vertical and horizontal movements and associated high pressure–low temperature (HP–LT) metamorphism owing to subduction and collision processes (Fig. 1). The continental basement units derived from the Adriatic margin are referred to Austroalpine units. The Dent Blanche nappe (Western Italian Alps) belongs to this domain (Fig. 1). This nappe is composed of pre-Alpine continental crust belonging to the African plate that heterogeneously recorded two tectonic and metamorphic stages of evolution: the pre-Alpine and the Alpine imprint (Caby, Kienast & Saliot, Reference Caby, Kienast and Saliot1978; Ballèvre & Kienast, Reference Ballèvre and Kienast1987; Canepa et al. Reference Canepa, Castelletto, Cesare, Martin and Zaggia1990; De Giusti, Dal Piaz & Massironi, Reference De Giusti, Dal Piaz, Massironi and Schiavo2003). The former is characterized by superimposed deformation stages associated with high temperature and high to intermediate pressure metamorphic imprints (granulite facies and amphibolite–granulite facies). The Alpine cycle is related to a low temperature and intermediate pressure metamorphic imprint (blueschist–greenschist facies) associated with superimposed mylonitic foliations (Roda & Zucali, Reference Roda and Zucali2008). As classically defined, the Dent Blanche nappe comprises a Palaeozoic basement and lenses of Mesozoic metasedimentary cover (i.e. the Roisan Zone and the Mt Dolin unit). The basement includes the Arolla Series and the Valpelline Series (Argand, Reference Argand1906; Diehl, Masson & Stutz, Reference Diehl, Masson and Stutz1952) from since-named units (Manzotti, Reference Manzotti2011).

Figure 1. (a) Tectonic map of the Alps (modified after Marotta & Spalla, Reference Marotta and Spalla2007). (b) Simplified geological map of the Dent Blanche tectonic system (Roda & Zucali, Reference Roda and Zucali2011). (c) NW–SE geological cross-section along the Western Alps: SL – Sesia-Lanzo Zone; MR – Monte Rosa; DB – Dent Blanche; PI – Penninic; SB – Grand Saint Bernard; MB – Mont Blanc; LPN – Lower Penninic; H – Helvetic; MO – Molasse (Zucali, Reference Zucali, Forster and Fitz Gerald2011).
The Arolla unit is mainly composed of Permian-age granite, clinopyroxene-bearing granite, diorite, gabbro metamorphosed and deformed during the Alpine tectonometamorphic evolution to orthogneiss, chlorite-white mica-amphibole-bearing gneiss and schist (De Leo, Biino & Compagnoni, Reference De Leo, Biino and Compagnoni1987; Pennacchioni & Guermani, Reference Pennacchioni and Guermani1993; Roda & Zucali, Reference Roda and Zucali2008). Within undeformed igneous cores, 1 m to 10 m sized xenoliths of biotite-sillimanite-bearing gneisses and amphibolites occur as remnants of pre-Alpine high temperature evolution (Diehl, Masson & Stutz, Reference Diehl, Masson and Stutz1952; Pennacchioni & Guermani, Reference Pennacchioni and Guermani1993; Roda & Zucali, Reference Roda and Zucali2008). The Arolla unit primarily records the Alpine tectonometamorphic evolution, whereas the pre-Alpine imprint is restricted to 1 to 10 m scale relics. Radiometric determinations on zircons of the Arolla unit orthogneiss indicate an age of 289 ± 2 Ma for their igneous protoliths (Bussy et al. Reference Bussy, Venturini, Hunziker and Martinotti1998).
The Valpelline unit comprises pre-Alpine high-grade (amphibolite- to granulite-facies) paragneisses with lenses and layers of basic granulites, garnet-clinopyroxene-bearing amphibolites and marbles (Diehl, Masson & Stutz, Reference Diehl, Masson and Stutz1952; Gardien, Reusser & Marquer, Reference Gardien, Reusser and Marquer1994).
The dominant metamorphic imprint occurs under amphibolite to granulite facies conditions, and it is of pre-Alpine age (Gardien, Reusser & Marquer, Reference Gardien, Reusser and Marquer1994). The Alpine imprint is weak and localized along high-strain zones that are tens to hundreds of metres wide. A static greenschist facies re-equilibration related to the development of an Alpine foliation is observed in certain places (Diehl, Masson & Stutz, Reference Diehl, Masson and Stutz1952; Kienast & Nicot, Reference Kienast and Nicot1971; De Leo, Biino & Compagnoni, Reference De Leo, Biino and Compagnoni1987; Roda & Zucali, Reference Roda and Zucali2008). Kienast & Nicot (Reference Kienast and Nicot1971) also described kyanite-chloritoid Alpine assemblages. Hunziker (Reference Hunziker1974) obtained ages of between 200 and 180 Ma for the Valpelline unit using the K–Ar and Rb–Sr methods on biotites. An age of 135 Ma has been derived using the K–Ar method on muscovites, and this age most likely related to a successive Alpine re-equilibration (Hunziker, Reference Hunziker1974; Gardien, Reusser & Marquer, Reference Gardien, Reusser and Marquer1994; Roda & Zucali, Reference Roda and Zucali2008) or may also be interpreted as a mixed age resulting from an incomplete re-equilibration between pre-Alpine and Alpine white micas.
The northeastern part of the Valpelline Valley is characterized by high-grade paragneisses with interlayered basic granulites, Grt-Cpx-bearing amphibolites and marbles (Diehl, Masson & Stutz, Reference Diehl, Masson and Stutz1952; Gardien, Reusser & Marquer, Reference Gardien, Reusser and Marquer1994). Anatectic veins and dykes of garnet-bearing pegmatite are common (Diehl, Masson & Stutz, Reference Diehl, Masson and Stutz1952; Pennacchioni & Guermani, Reference Pennacchioni and Guermani1993). Low- to medium-grade micaschists with interlayered calc-silicate bands occur in the southwestern part (Pennacchioni & Guermani, Reference Pennacchioni and Guermani1993). Metapelites are generally banded, and foliation is defined by the alternation of dark (biotite and garnet) and light (quartz and plagioclase) layers and by the shape preferred orientation (SPO) of biotite and sillimanite (Gardien, Reusser & Marquer, Reference Gardien, Reusser and Marquer1994). Generally, the mineral assemblage of these gneisses consists of garnet, sillimanite, biotite, plagioclase, quartz, rutile, oxide, feldspar, muscovite and cordierite (Diehl, Masson & Stutz, Reference Diehl, Masson and Stutz1952; Gardien, Reusser & Marquer, Reference Gardien, Reusser and Marquer1994). The lithological boundaries are generally parallel to the dominant foliation, which is marked by biotite and sillimanite in gneiss, amphiboles and plagioclase in amphibolite, and calcite and clinopyroxene in marble. This penetrative foliation can be traced for several kilometres along the valley, making this structure a robust chronological benchmark. This foliation is locally bent: folds that range from metres to decametres in size are tight to isoclinal, and they are often associated with the development of a new axial plane foliation.
Thermobarometrical estimates for the Valpelline unit indicate a first stage at T = 700–800°C and P = 9–10 kbar characterized by the growth of kyanite prior to the migmatite stage at T = 800–850°C and P = 6–7 kbar, which follows an amphibolite re-equilibration stage at T = 750–800°C and P = 5–6 kbar (Gardien, Reusser & Marquer, Reference Gardien, Reusser and Marquer1994). The high temperature pre-Alpine evolution ends with a static stage of re-equilibration at T = 650–750°C and P = 3–5 kbar (Gardien, Reusser & Marquer, Reference Gardien, Reusser and Marquer1994; Gardien, Reference Gardien1994). Greenschist facies conditions (T = 450–500°C, P = 3–4 kbar) are recorded in all lithologies but cannot be easily attributed to the pre-Alpine or Alpine cycles (Roda & Zucali, Reference Roda and Zucali2008; Manzotti, Reference Manzotti2011). The similar pressure and temperature conditions recorded by the two units suggest that they underwent a comparable polycyclic evolution. At present, the contact between the Valpelline and Arolla units is notably sharp: the transition occurs in a few tens of metres, and it is marked by Alpine-age greenschist-facies mylonites and cataclasites (Pennacchioni & Guermani, Reference Pennacchioni and Guermani1993).
We focused our study on rocks cropping out in a small canyon (Fig. 2; UTM WGS84 coordinates: 372874–5077575; 373168–5077213), which is 8–10 m wide and approximately 400 m long (between 1080 m and 1300 m altitude). This canyon is situated on the northern flank of Valpelline Valley between the villages of Valpelline and Oyace, close to Thoules. In this area, the older stages of the structural evolution, pre-dating the dominant foliation, are preserved in metre-scale basic granulite boudins wrapped in migmatites.

Figure 2. Lower hemisphere Schmidt (equal area) projections of the structures recognized in the studied area.
3. Approach and methods used
Because the geological history of a metamorphic terrane is generally composed of successive or contemporaneous magmatic, metamorphic and deformational evolutionary stages (hereafter simply referred to as stages), we approached the reconstruction of metamorphic terranes by separating each stage on the basis of its characteristics at meso- and microscopic scales following the approach described by Spalla et al. (Reference Spalla, Zucali, Di Paola, Gosso, Gapais, Brun and Cobbold2005, Reference Spalla, Gosso, Marotta, Zucali and Salvi2010). The relative timing of structures inferred by superimposed fabric elements (Turner & Weiss, Reference Turner and Weiss1963) and the analysis of the mineral assemblages marking each fabric element allow for the definition of the relative timing of structures within each lithology (i.e. phases of deformation, such as D1 and D2) with respect to the metamorphic conditions in which they developed.
Criteria for structural correlation among all stages were applied at 1:100 to 1:400 mapping scales (Turner & Weiss, Reference Turner and Weiss1963; Passchier & Trouw, Reference Passchier and Trouw2005). Data are represented in a structural-metamorphic map in which lithotypes and fabric elements are highlighted (Fig. 8).
Microstructural analysis was used to identify the metamorphic assemblages, to study the correlation between deformation and metamorphism, and to select areas of special interest to be used for chemical microprobe analyses and P–T calculations. Mineral phases have also been chronologically distinguished (e.g. GrtI, GrtII) using as indicators their microstructural positions, optical characters and relative growth microstructures (Vernon, Reference Vernon2004; Passchier & Trouw, Reference Passchier and Trouw2005; Vernon & Clarke, Reference Vernon and Clarke2008). In some cases, the relative chronology of the single mineral phases does not correspond to the stage chronology (e.g. GrtII in stage 3), and in large part, this chronology has been validated by the chemical compositions of the mineral phases and by thermobarometrical estimates. Large- and small-scale processes may be linked by combining these different techniques and exploiting the heterogeneous distribution of metamorphism and deformation. The relative chronology of P–T estimates is subsequently used to reconstruct the P–T–t–d paths, and the tectonometamorphic evolution of the Valpelline unit rocks will be presented thereafter in the pre-Alpine tectonic and geodynamic framework.
Mineral abbreviations are in accordance with Whitney & Evans (Reference Whitney and Evans2010); in addition, white mica is abbreviated as Wm, and opaque minerals abbreviated as Op.
4. Meso- and microstructures and their mineral support
4.a. Rock types and their relationships
The area is dominated by metapelites (migmatite and Bt-bearing gneiss), metabasites (basic granulite and amphibolite), Ol-bearing marbles and Grt-bearing leucosomes (Fig. 3).

Figure 3. Field photographs of metapelites and metabasites of the Valpelline unit. (a) Opx-bearing basic granulite lens in migmatites. In migmatites proximal to the contact with basic granulite a centimetre-thick Fsp-Grt-rich neosome (neo) occurs. (b) Amphibolite: Spre2 is marked by centimetre-thick layers, respectively, of Pl, black-brown Amp and white-green Amp. A leucosome pocket, associated with stage 2, cuts this structure. (c) Ol-bearing marble layer with a weak Spre2 foliation. (d) Granoblastic aggregate of Qz + Grt + Pl + Crd in a leucosome. (e, f) Migmatites: stage 2 melanosomes and leucosomes alternate. Leucosomes, produced by stage 2, contain Qz, Fsp and locally Grt.
Migmatites (Fig. 3a) consist of metatexites in which leucosomes may occur as centimetre-sized spots; granoblastic aggregates of Qz + Kfs + Pl + Grt ± Crd (Fig. 3b) are commonly oriented parallel to the dominant foliations. Locally, the leucosomes may be more prominent and continuous, defining centimetre- to metre-thick layers associated with variably thick melanosomes. Melanosomes are generally flat, and the major constituents are Bt, Grt and Sil. Locally, Grt-rich restites occur with a modal amount of > 50–60%. Bt-bearing gneisses are characterized by fine to medium grains and display a pervasive foliation. Millimetre- to centimetre-thick Qz-Fsp lithons are alternated with millimetre-thick Bt films. Locally, Grt-rich layers are also present and are parallel with Bt films and Qz-Fsp lithons.
Opx-bearing basic granulite and amphibolite occur as metre-scale boudins within migmatite (Fig. 3a, b) or as decametre-scale bodies interlayered with pelitic migmatites and Bt-bearing gneisses. Opx-granulites are characterized by fine to medium grains and show granoblastic texture. These rocks are commonly massive, and a weak foliation marked by the Pl- and Grt-rich layers is only locally observed at the boundaries of the granulite boudins (Fig. 3a).
Grt-bearing leucosomes (i.e. pegmatites, according to Diehl, Masson & Stutz, Reference Diehl, Masson and Stutz1952) occur at the boundaries between migmatites and basic boudins (Fig. 3a, c, d).
The mineralogical compositions and the amounts of each mineral in different lithologies are reported in Table 1.
Table 1. Mineralogical compositions and amount of each mineral in the different lithologies

4.b. Stages of evolution
In the studied area, four evolutionary stages have been recognized at the meso- and micro-scale. The unravelled structural and metamorphic evolution is summarized in the following paragraphs, whereas Table 2 synthesises the superimposed mesostructures and their relative relationships. The orientations of fabric elements are plotted on Schmidt diagrams in Figure 2.
Table 2. Schematic relationships between deformation and metamorphism in the Valpelline Unit

Met – metamorphic; D – deformational; Mag – magmatic.
S – foliation; L – lineation; F – fold; AP – axial plane.
In Figures 4 and 5, the relationships between microstructural evolution and metamorphic growth are summarized. Table 3 reports stable metamorphic assemblages, which are chronologically ordered on the basis of the following microstructural analysis. The subscript associated with each mineral group refers to the relative timing of growth and not to the stages. The map in Figure 8 displays lithological associations and superimposed foliations together with indications of the fabric-supporting minerals.
Table 3. Pre-Alpine and Alpine deformational, magmatic and metamorphic events (D, Mag, Met) of the Valpelline rocks, recognized at the microscale. Metamorphic assemblages marking each fabric are also described


Figure 4. Photomicrographs of observed microstructures of metapelites and metabasites of the Valpelline unit. (a) Basic granulite: polygonal to interlobate aggregate of CpxI, OpxI and PlI (crossed polars). (b) Bt-bearing gneiss: GrtI occurs as an inclusion-rich core within the Spre2 foliation (plane-polarized light). (c) Pelitic migmatite: millimetre-sized rounded grains of GrtII in a melanosome (plane-polarized light). (d) Pelitic migmatite: aggregates of large fibrolitic SilII partly truncate GrtII rims and define the S2 foliation (plane-polarized light). (e) Pelitic migmatite: SilII aggregates partly replace BtII (plane-polarized light). (f) Pelitic migmatite: subhedral crystal of PlII with growth twins (crossed polars). (g) Pelitic migmatite: leucosome with a coarse-grained granoblastic texture, characterized by PlII, KfsII and QzII (crossed polars). (h) Pelitic migmatite: CrdI crystals are replaced by aggregates of Wm (crossed polars).

Figure 5. (a) Contact (C) between pelitic migmatite and a lens of basic granulite (B). (b) Basic granulite at the contact with migmatite: interlobate aggregate of OpxII + CpxII + PlII (crossed polars). (c, d) Pelitic migmatite at the contact with basic granulite: SplI + GrtII and SplI + SilII aggregates (plane-polarized light). (e) Amphibolite: shape preferred orientation of AmpI defines the S3a foliation (crossed polars). (f) Pelitic migmatite: Grt porphyroblasts with WmI-filled fractures (crossed polars).
4.b.1. Stage pre2
Stage pre2 is characterized by structures that are clearly prior to stage 2. This stage is locally recognizable in different lithologies (e.g. boudins of Opx-bearing granulites wrapped within pelitic migmatites (Fig. 3a), amphibolite (Fig. 3b) and Ol-bearing marbles (Fig. 3c)). Stage pre2 likely comprises various events, as locally testified by the different metamorphic assemblages associated with the structures (e.g. amphibole marking a Spre2 foliation in amphibolite and orthopyroxene layers developing in basic granulite). For this reason, we only refer to stage pre2 in reference to all structures pre-dating stage 2. Parts a–c and e of Figure 3 show the geometric overprinting relationships between stage 2 structures (e.g. S2 foliation, boudinage and melt pocket) and those pre-dating them.
4.b.1.a. Meso-scale observations
Dpre2 structures are more evident in Opx-bearing granulite boudins, amphibolites and marbles and mainly consist of a weak centimetre- to millimetre-thick Spre2 foliation. Spre2 in granulites are rare; where present, they are marked by a gentle SPO of Pl, Cpx and Opx.
In amphibolites, the Spre2 foliation is defined by centimetre-thick mineralogical layers alternating between Amp- and Pl-rich. The SPO of Amp also defines this structure (Fig. 3b).
4.b.1.b. Micro-scale observations
In basic granulites, an inequigranular polygonal to interlobate texture is preserved. It is characterized by subhedral crystals of OpI, BtI and ApI and by clustered polygonal to interlobate aggregates of PlI, CpxI and OpxI (Fig. 4a). Euhedral to subhedral OpxI and CpxI are commonly prismatic in shape, ranging from millimetres to centimetres in size with well-defined and straight grain boundaries. BtI occurs as single grains (0.5–1 mm) and locally shows undulose extinction. OpI and ApI occur as millimetre-sized single crystals in close association with OpxI, CpxI and PlI.
In Bt-gneisses, stage pre2 is also preserved; it occurs within metre-sized volumes where aggregates of QzI + PlI + KfsI + BtI + GrtI ± SilI are recognizable. QzI occurs as strained grains or aggregates with undulose extinction and deformation bends. PlI shows a prismatic anhedral to subhedral habit up to several millimetres in size, and it is characterized by deformational features such as undulose extinction and deformation twinning. QzI and PlI form microlithon domains that are elongated to BtI aggregates. BtI and rarely SilI form microfilm domains. GrtI crystals are wrapped by the main foliation marked by the SPO of BtI ± SilI and by QzI elongate aggregates. GrtI occurs as millimetre-sized euhedral grains with inclusions of Qz and BtI (Fig. 4b). ZrnI is commonly found as euhedral prismatic crystals with high relief and birefringence, and they are frequently associated with GrtI. MnzI occurs as rounded, high-relief crystals with high birefringence associated with SilI or as inclusions in GrtI and BtI.
Therefore, the association of BtI + QzI + PlI + KfsI + GrtI + ZrnI + MnzI + RtI + ApI ± SilI defines the relict Spre2 foliation in metapelites (Table 3).
4.b.2. Stage 2
During stage 2, migmatite fabrics develop in metapelites. Contemporaneously, basic granulites and amphibolites partly or completely change their mineral associations; such reactions are localized at the basic boudin boundaries where stage 2 neosomes localize (Fig. 3a; Table 2). This stage is not recorded in marbles, whereas it is maximally developed in metapelites.
4.b.2.a. Meso-scale observations
In pelitic migmatite, D2 structures consist of centimetre- to millimetre-thick S2 foliation, expressed by alternating layers of leucosomes (Fig. 3d) and melanosomes (Figs 3e, f). The leucosomes are disharmonically folded and boudined in the fold limbs. The leucosomes hold their granoblastic structure, even where folded during D2. The melanosomes are variable in width and are composed of Sil, Grt, Bt, Pl and Kfs. The SPOs of Sil and flattened Grt layers define the S2 foliation in melanosomes together with the SPO of Bt (Fig. 3f). Marble, amphibolite and granulite boudins are wrapped by the S2 foliation at decimetre to metre scales. Centimetre-thick leucosomes are also localized along the boundaries between granulite-amphibolite boudins and migmatites (Figs 3a, b). These boundaries are usually parallel to the S2 foliation that it is marked by Bt + Sil-rich layers in migmatites and by the increase in the amount of Grt and Amp within granulite and amphibolite boudins. In amphibolite boudins, Spre2 foliation generally tends to be folded and parallel to the external S2 foliation, especially at their margins, as is also shown by stereographic projection in Figure 2.
4.b.2.b. Micro-scale observations
In pelitic migmatite, melanosomes and leucosomes are easily distinguished by their textures: leucosomes are defined by granoblastic polygonal aggregates of QzII + PlII + KfsII + GrtII + BtII, whereas melanosomes preserve a spaced foliation marked by the SPO of BtII + QzII + GrtI/II + SilII. At the micro-scale, the distinctive character of the melanosomes is the high modal proportion of residual and accessory minerals, such as Grt and Sil. GrtII occurs as millimetre- to centimetre-sized grains, locally zoned (Fig. 4c); the inner part (i.e. GrtI) is rich in inclusions of RtI, QzI, BtI and SilI, whereas the outer part (GrtII) is inclusion-free. Large and rounded inclusions of QzI and BtI are common in GrtI, as are aggregates of BtI + SilI; such relationships may be interpreted as the relics of the older reactant assemblage (Sawyer, Reference Sawyer2008; Kriegsman & Alvarez-Valero, Reference Kriegsman and Alvarez-Valero2010). The rims of GrtII may be partly truncated or dissolved by aggregates of large, fibrolitic SilII (Fig. 4d). S2 foliation, marked by the SPO of SilII, BtII and minor QzII, KfsII and PlII, is generally well developed in melanosomes. BtII has a more homogeneous appearance than BtI: it is more reddish in colour and commonly is not internally strained. BtII may be partly replaced by SilII (Fig. 4e). SilII occurs as prismatic porphyroblasts or as elongate fibrolitic domains defining S2 foliation (Fig. 4e). Euhedral crystals of SilII may occur in association with GrtII rims and BtII and indicate a likely reaction involving SilII as a product of GrtII and BtII. This reaction is also suggested by the presence of SilII aggregates, generally parallel to S2 foliation, at the rims of GrtII; these microstructural relationships are commonly found in melanosomes close to leucosomes containing microstructures that indicate crystallization from melt (see below). PlII (0.5–6 mm) forms subhedral or anhedral crystals, and many of them show growth twins (Fig. 4f), suggesting growth from melt (Vernon, Reference Vernon2004; Sawyer, Reference Sawyer2008), whereas few grains are characterized by undulose extinction, deformation bands and twins. RtII may occur as millimetre-sized single crystals or as inclusions within BtI and BtII. ZrnII and MnzII are also enclosed within QzII and SilII.
Leucosomes display coarse-grained granoblastic textures; PlII, KfsII and QzII constitute the main frame of the texture (Fig. 4g). The interstitial space is filled by QzII, KfsII and BtII, thereby defining thin films. GrtII and BtII crystals that occur in the leucosome are relatively strain-free euhedral grains. Locally, millimetre-sized CrdI porphyroblasts occur, but they have generally been replaced by aggregates of Wm (Fig. 4h). MnzII and ZrnII occur in association with QzII, BtII and GrtII.
Consequently, the assemblages marking the fabrics developed during stage 2 and thus interpreted as stable during this stage are as follows (Table 3): Melanosomes: GrtII + SilII + QzII + PlII + KfsII + BtII + MnzII + ZrnII + RtII
Leucosomes: QzII + PlII + KfsII + GrtII ± CrdI + BtII + MnzII + ZrnII Marble, amphibolite and granulite boudins are wrapped by the S2 foliation at decimetre to metre scales. Centimetre-thick leucosomes are also localized along the boundaries between granulite-amphibolite boudins and migmatites (Fig. 3a, b). These boundaries are usually parallel to the S2 foliation that it is marked by Bt+Sil-rich layers in migmatites and by the increase in the amount of Grt and Amp within granulite and amphibolite boudins. In amphibolite boudins, Spre2 foliation generally tends to be folded and parallel to the external S2 foliation, especially at their margins (Fig. 3e), as is also shown by stereographic projection in Figure 2.
Stage 2 is also recorded at the contact between migmatites and lenses of basic granulites (Fig. 5a). The recrystallization of Opx, Cpx and Pl occurs in basic granulite, developing OpxII + CpxII + PlII + GrtI polygonal or interlobate inequigranular aggregates (Fig. 5b). In the central portions of basic granulite, S2 is expressed by the SPO of Amp and by Pl layers. In the corresponding migmatite, S2 is a spaced foliation marked by GrtII-rich bands alternating with PlII–QzII-rich layers. The SPO of BtII also marks this structure. PlII crystals (2–6 mm) show growth and deformation twins, and the grain boundaries are straight and rational. BtII grains are generally euhedral and define Bt-rich layers that commonly rim GrtII bands and PlII–QzII layers. Spl + GrtII and Spl + SilII aggregates may locally occur (Fig. 5c, d). Spl is found as anhedral crystals (0.5–2 mm) with amoeboid grain boundaries.
4.b.3. Stage 3
Stage 3 is characterized by two superimposed deformational phases that rework the pre-existing structures. Both deformational phases are marked by the same metamorphic minerals, thereby supporting the decision to group them into a single stage.
4.b.3.a. Meso-scale observations
During stage D3a, the S2 foliation is folded, and a new axial plane foliation S3a develops. In migmatites, S3a is marked by the SPO of Bt and Sil aggregates, which are associated with Grt grains and are frequently elongated parallel to this structure. In granulites, S3a is marked by dark, centimetre-thick Amp ± Bt layers and light, millimetre- to centimetre-thick Pl–Cpx layers. In amphibolites, S3a is expressed by alternating millimetre- to centimetre-thick Pl- and Amp-rich layers. In certain cases, S3a is also marked by the SPO of Amp and by layers and lenses constituted by Grt and green-grey Cpx. In marbles, the SPO of carbonate and locally Qz- and Cpx-rich layers usually define this fabric. The orientation of the S3a foliation is generally settled in an area of 25–30 km2 and is roughly parallel to the lithological boundaries (see the stereographic projections in Fig. 2).
Stage D3b is associated with close to isoclinal folds in all lithotypes. In migmatites and amphibolites, the D3b deformation phase may be locally associated with the development of a new axial plane foliation, S3b, which is visible at the decametre to metre scale. Generally, S3a and S3b are marked by the same minerals, and they may be easily recognized only where superimposed relationships are preserved. Associated with this phase of deformation, centimetre- to metre-thick Bt + Grt-bearing leucosomes occur, cross-cutting lithological boundaries and S2, S3a and S3b foliations or locally parallel to S3a or S3b structures. Such geometrical relationships suggest a development during the final stage of D3.
4.b.3.b. Micro-scale observations
D3a structures are well recorded in migmatites. S3a is a spaced foliation marked by BtII + GrtII layers and by QzII + PlII + KfsII lithons. BtII occurs as euhedral crystals with straight and well-defined grain boundaries. QzII occurs as millimetre- to centimetre-sized crystals with undulose extinction. S3a is also marked by the SPOs of BtII and SilII; SilII forms elongate crystals (0.2–10 mm) with straight and sharp grain boundaries or elongate, fibrolitic domains.
In metabasites, S3a is marked by Pl + Qz and by AmpI ± GrtII layers. Pl (PlI and PlII) occurs as subhedral crystals in association with CpxII, locally displaying wavy extinction and deformation twins. AmpI is found as elongated or rhomboid crystals (1–9 mm) that are brown or pale green in colour (Fig. 5e).
Syn-stage 3 leucosomes are defined by the association KfsIII + QzIII +BtIII + GrtIII + RtIII + MnzIII + ZrnIII. Leucosomes display equigranular size distribution with a polygonal shape. BtIII, KfsIII and GrtIII (2–20 mm) have idiomorphic shapes. QzIII has an anhedral shape; the grain size varies from less than 0.5 mm to 1.5 cm. Crystals frequently show undulose extinction, indicating that local deformation occurred after the intrusion. MnzIII and ZrnII occur as millimetre-sized grains with a euhedral shape associated with QzIII, GrtIII and BtIII.
4.b.4. Stage 4
Stage 4 is not perceivable at the scale of the Valpelline unit, and it is generally associated with the static growth of Chl and Wm in migmatites and by the static replacement of Pl by Ep and Wm in basic granulites. At the valley scale, a millimetre-thick foliation (S4) locally develops; this foliation is marked by Wm and Chl and may be also mylonitic (Pennacchioni & Guermani, Reference Pennacchioni and Guermani1993; Roda & Zucali, Reference Roda and Zucali2008). In the studied area, this structure does not develop, but Chl and Wm statically overgrow the old foliations. The late stage 4 is also characterized by the development of brittle, metre-scale structures as faults and cataclasites.
Chl and Ep may also be found as seals of brittle faults. In these zones, faults and fracture systems produce the disaggregation of the protoliths and the loss of the primary mineralogical and fabric characters.
4.b.4.a. Meso-scale observations
The S4 foliation is marked by greenschist-facies minerals in all rock types. In metapelites, this foliation is expressed by Wm and Chl SPOs; in amphibolite, by green Amp SPO; and in marble, by Chl and Cal SPOs. This deformation phase is not homogeneously distributed within the Valpelline unit because it is localized along metres- to tens-of-metres-thick bands, particularly at the contact between the Arolla and the Valpelline units (Pennacchioni & Guermani, Reference Pennacchioni and Guermani1993). S4 foliation is parallel to the contact between the Arolla and Valpelline units, and it is subsequently folded by recumbent folds.
4.b.4.b. Micro-scale observations
In metapelites, WmI fine-grained aggregates and irregularly shaped aggregates of EpI partly or completely replace PlI, KfsI, SilI and SilII. ChlI aggregates and fine-grained WmI partly replace all generations of Grt and Bt. Grt porphyroblasts contain WmI- and ChlI-filled fractures (Fig. 5f). ChlI (0.5–3 mm) also forms elongate aggregates in which crystals frequently show wavy extinction. CalI occurs as anhedral grains (0.5–2 mm) with lobate and well-defined grain boundaries.
In metabasites, pale green AmpII partly or completely replaces CpxI, OpxI and AmpI. ChlI aggregates and fine-grained WmI partly replace AmpI and GrtI. WmI fine-grained aggregates and irregularly shaped aggregates of EpI partly or completely replace PlI and PlII. These newly formed minerals may grow along fractures.
5. Mineral chemistry
Minerals were analysed with an electron microprobe (EMPA: JEOL 8200 Super Probe) and a scanning electron microscope (SEM: Cambridge Stereoscan 360 ISIS 300 Oxford) at the Dipartimento di Scienze della Terra ‘A. Desio’ – Università degli Studi di Milano. The operating conditions were 20 kV accelerating voltage, a filament intensity of 1.70 A and a probe intensity of 280 pA for EMPA and 15 kV accelerating voltage and a sample current of 190 pA for SEM. Natural silicates were used as standards; matrix corrections were calculated with the ZAF procedure. Representative mineral compositions are reported in the online Supplementary Material available at http://journals.cambridge.org/geo (Tables S1a–g). Figure 6 shows chemical variations within selected mineral phases with respect to their microdomain occupancy and evolutionary stage. Mineral formulae have been recalculated using JPT (Zucali, Reference Zucali2005), which uses the general recalculation scheme after Deer, Howie & Zussman (Reference Deer, Howie and Zussman1996).

Figure 6. Graphic representation of the chemical composition of selected mineral phases: (a) biotite; T°C isolines after Henry, Guidotti & Thomson (Reference Henry, Guidotti and Thomson2005); (b) amphibole; (c) garnet; (d) pyroxene.
Biotite. Biotite in gneiss and migmatite commonly coexists with ilmenite and shows a linear increase in Ti content from BtI to BtII. Ti content in BtI ranges from 0.342 to 0.798 atoms per formula unit (a.p.f.u.), and it is between 0 and 1 a.p.f.u. in BtII. Among BtII, it is also possible to separate low-Ti minerals from those with high to very high Ti content (Fig. 6). XMg varies from 0.40 to 0.80 in both BtI and BtII. AlVI content also varies from 0 to 1, and it is closely related with the Ti content variations, as suggested by various studies (Scheurs, Reference Scheurs1985; Henry, Guidotti & Thomson, Reference Henry, Guidotti and Thomson2005). In metabasite (basic granulite and amphibolite), Bt compositions are generally homogeneous: Ti content varies from 0.52 to 0.72 a.p.f.u., AlVI from 0 to 0.184 a.p.f.u., and XMg from 0.52 to 0.65.
Amphibole. In metabasites, AmpI (stages 2 and 3) shows a Ca content between 1.5 and 3.5 a.p.f.u., whereas Si varies between 6.71 and 6.71 a.p.f.u. Ti content varies from 1.40 to 3.20 a.p.f.u. In metabasites (stage 3), AmpII displays an Si content between 7 and 8 a.p.f.u., whereas Ti varies between 0 and 1.0 a.p.f.u.
Garnet. GrtI and GrtII in metapelites are Fe-rich (1.68–2.00 a.p.f.u.) with a high Mg content (0.76–1.09 a.p.f.u.) and lower contents of Ca (0.08–0.48 a.p.f.u.) and Mn (0.02–0.06 a.p.f.u.). The Alm content ranges from 59 to 67%, whereas the Py content varies from 27 to 37%. GrtII rims commonly show an increase in the Mg and Ca contents compared with GrtI cores.
GrtI (stage 2) in basic granulites and amphibolites shows a Ca content varying from 0.43–0.60 a.p.f.u., a Mg content from 0.58–0.76 a.p.f.u. and an Fe content from 1.70–1.86 a.p.f.u.
Orthopyroxene. OpxI (stage 1 in basic granulite) and OpxII (stage 2 in granulite at the contact with migmatitic gneiss) are hypersthenic, with XMg increasing from 0.47 (OpxI) to 0.61 (OpxII). Al in Opx ranges from 0.033 to 0.061 a.p.f.u.
Clinopyroxene. CpxI (stage 1 in basic granulites) and CpxII (stage 2 in granulite at the contact with migmatitic gneiss) are clino-hypersthenic, showing XMg between 0.45 and 0.50. Al in Cpx ranges from 0.048 to 0.061 a.p.f.u.
Feldspars. PlI in metapelite is characterized by a homogeneous Ab content of approximately 0.74. The An content varies in PlI, where it may increase up to 0.32. In metabasite, PlII has a higher An content, which varies from 0.45 to 0.80. Orthoclase occurs in association with PlII (KfsII) and within pegmatite veins (KfsIII).
Spinel. In migmatite (stage 2), spinel is part of a hercynite–spinel–ghanite solid solution, where XSpl varies from 0.25 to 0.39, XHc from 0.39 to 0.52 and XGah from 0.15 to 0.36.
Table S1g in the online Supplementary Material available at http://journals.cambridge.org.geo also reports selected analyses of stage 4 chlorite and white mica. Chl compositions vary in the Mg, Al and Si contents, whereas Wm compositions distinguish two generations that may be discriminated by the variations in the Si, Al, Mg and Fe contents.
6. Estimates of pressure and temperature conditions
Available petrogenetic grids and thermobarometry were used to estimate the physical conditions of metamorphic stages (Fig. 7), as reconstructed by meso- and microstructural analyses. Microstructural analysis allowed for the recognition of reactions that occurred during metamorphic evolution and their relative timing. Mineral pairs and stable associations were used for thermobarometric estimates (Table 4). Pressure and temperature conditions and the reactions deduced by microstructures are compared with data available from petrogenetic grids. Temperatures were estimated using garnet-biotite Fe-Mg exchange thermometry (Bhattacharya et al. Reference Bhattacharya, Mohanty, Maji, Sen and Raith1992; Wu & Cheng, Reference Wu and Cheng2006) and using the Ti content in biotite with the calibration of Henry, Guidotti & Thomson (Reference Henry, Guidotti and Thomson2005). The pressures were estimated using a garnet-aluminosilicate-silica-plagioclase (GASP) barometer for which the calibration of Holdaway (Reference Holdaway2001) was employed, and a using garnet-plagioclase-biotite-quartz (GPBQ) barometer with the calibration of Hodges & Spear (Reference Hodges and Spear1982). Average P–T estimates were calculated using Thermocalc v. 3.33 (Holland & Powell, Reference Holland and Powell1998) in mode 2; the activities of the end-member minerals from chemical analyses were calculated using AX software (Holland, Reference Holland2000) and used as input.
Table 4. Thermobarometric estimates for the Valpelline rocks, with reference to the method used (see text for calibration references)

avPT – Thermocalc v. 3.33 mode 2 calculations using activities from microprobe analysis as input.
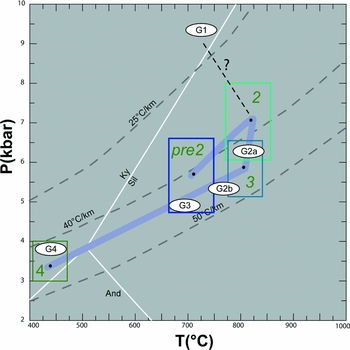
Figure 7. P–T–t–d paths reconstructed on the basis of meso- and microstructural relationships and P–T estimates. G1, G2a, G2b, G3 and G4 stages from Gardien, Reusser & Marquer (Reference Gardien, Reusser and Marquer1994); boxes and chronology from this work. 25–40–50°C km−1 geothermal gradients are shown. Ky, And, Sil stability fields calculated using the thermodynamic database of Holland & Powell (Reference Holland and Powell1998).
Stage pre2 is preserved in different lithologies (Bt-bearing gneisses, Opx-bearing granulites, amphibolites and Ol-bearing marbles), and frequently, this stage is associated with the development of the Spre2 foliation. Thermobarometric estimates on mineral pairs and stable associations for stage pre2 in Bt-bearing gneiss are shown in Table 4; temperatures are between 703 ± 42°C, and pressures are in the range of 4.5 to 6.5 kbar. Similar P–T intervals are shown by P–T pseudosections reconstructed for comparable bulk compositions in the model system Na2O–CaO–K2O–FeO–MgO–Al2O3–SiO2–H2O (NCKFMASH) (e.g. White, Powell & Holland, Reference White, Powell and Holland2001) for the Bt-bearing assemblages in the absence of melt.
During stage 2, a pervasive migmatization affects metapelites, and a centimetre- to millimetre-thick S2 foliation develops, which is marked by the alternation of leucosome and melanosome. Some microstructures recognized in migmatites (e.g. growth twins and SillII associated with GrtII) suggest crystallization and growth processes from a melt, and these microstructures may be a result of melt-producing continuous reactions involving Bt as a reactant (White, Powell & Holland, Reference White, Powell and Holland2001; White & Powell, Reference White and Powell2002), indicating T ≥ 800°C and P > 6 kbar. The rare appearances of garnet + cordierite + orthopyroxene by biotite-melting reactions have been recently investigated using a P–T pseudosections approach (Johnson, White & Powell, Reference Johnson, White and Powell2008) and in natural rocks (Bhandari et al. Reference Bhandari, Pant, Bhowmik and Goswami2011). Bhandari et al. (Reference Bhandari, Pant, Bhowmik and Goswami2011) also observed that garnet grew to the exclusion of cordierite and orthopyroxene in a relatively ferroan bulk-rock composition, whereas garnet and cordierite appeared in more magnesian and aluminous compositional domains. The biotite-melting reactions suggested by the authors for the NCKFMASH system are Bt + Pl + Qz = Grt + Kfs + melt (for garnet alone), Bt + Sil + Pl + Qz = Grt + Crd + Kfs + melt and Bt + Pl + Qz = Grt + Crd+ Kfs + melt (for garnet + cordierite), thereby producing mineral associations similar to those that were stable during this stage and indicating T > 770°C at P > 6 kbar (Johnson, White & Powell, Reference Johnson, White and Powell2008). Gardien, Reusser & Marquer (Reference Gardien, Reusser and Marquer1994) estimated P > 5 kbar at T > 750°C for this stage. Thermobarometric estimates on mineral pairs and stable associations of stage 2 in migmatites result in T = 814 ± 40°C and a P interval of 6–8 kbar (Table 4).
Stages 3a and 3b comprise two superimposed deformational phases that rework the pre-existing structures. Both deformational phases are marked by the same metamorphic minerals (BtII + SilII + GrtII layers and QzII + PlII + KfsII lithons). Gardien, Reusser & Marquer (Reference Gardien, Reusser and Marquer1994) estimated a pressure of 4–6 kbar and temperature of 750–800°C for these stages, characterized by the assemblage Bt + Sil + Pl + Qz ± Grt ± Kfs in metapelite. On the basis of the absence of cordierite in migmatite and using the experimental curve Phl + Sil + Qz = Mg-Crd (Spear & Cheney, Reference Spear and Cheney1989), Gardien, Reusser & Marquer (Reference Gardien, Reusser and Marquer1994) constrained the lower pressure for the same stages at 5.5 kbar. This curve cross-cuts at 6 kbar the experimental curve between medium pressure and low pressure granulites calibrated by Green & Ringwood (Reference Green and Ringwood1967). Thermobarometric estimates on mineral pairs and stable associations of stages 3a and 3b in amphibolite indicate temperatures of 822 ± 31°C and pressures of 5–6.5 kbar (Table 4). Stage 3 is also characterized by the crystallization of the magmatic assemblage Grt + Bt + Kfs + Qz in leucosomes (i.e. pegmatites). This assemblage is related to the melt-producing reactions involving Bt + Sil + Qz + Pl as reactants at T > 800°C and P > 6 kbar (White, Powell & Holland, Reference White, Powell and Holland2001; Johnson, White & Powell, Reference Johnson, White and Powell2008). This P–T estimate agrees with the mesoscopic observations that suggest the contemporaneous development of Grt-bearing leucosomes and of the S2 and S3 foliations.
During stage 4, WmI and EpI replace Pl, following the reaction Pl + H2O = EpI + WmI + Qz (Holland & Powell, Reference Holland and Powell1998). Gardien, Reusser & Marquer (Reference Gardien, Reusser and Marquer1994) described a similar pre-Alpine metamorphic imprint occurring under greenschist facies conditions (T < 500°C and P ≈ 3–4 kbar). Kfs + Sil + H2O = Wm + Qz + L and Bt + Kfs + Sil + H2O = Wm + Qz reactions may also indicate the replacement of Sil, Bt and Kfs by WmI and Qz. During the same stage, Bt and Grt are replaced by ChlI, probably through the reaction Grt + Bt +Sil + H2O = Chl + Wm + Qz (Spear & Cheney, Reference Spear and Cheney1989). The AlIV content in the Chl thermometer (Chatelineau, Reference Chatelineau1988) infers T = 339 ± 16°C in Bt-bearing gneiss and migmatite and T = 455 ± 38°C in amphibolite.
7. Discussion and interpretation
7.a. Tectonometamorphic evolution
The Valpelline unit is characterized by a polyphase evolution from pre-Permian to Alpine age and under granulite to greenschist facies conditions. In the studied area (300 × 50 m), the deformational and metamorphic imprints are heterogeneously distributed in space, thereby producing a chronological and spatially heterogeneous network of structures and metamorphic associations (Fig. 8). In the studied rocks, the pre-Alpine history is characterized by four evolutionary stages.

Figure 8 Structural and metamorphic map of the study area, showing metre-scale relationship between lithologies, superimposed structures and relative fabric-supporting minerals related to the recorded metamorphic imprints. On the map, the dots along the foliations trace the relative chronology of superimposed foliations. Mineral assemblages related to successive fabrics in each rock type are represented by differently coloured trajectories and by different symbols associated with the trajectories (Spalla et al Reference Spalla, Siletto, Di Paola and Gosso2000). Also shown are heterogeneous P–T–t–d memories, registered in adjacent rock domains. The figure shows distribution of domains where structural and metamorphic imprints are better recorded with respect to degree of fabric development. Pencil for scale is 13 cm long; coin is 1 cm in diameter; ruler is 20 cm long; and hammer is 40 cm long.
Stage pre2 comprises a complex structural and metamorphic evolution prior to stage 2. In this stage, we group several phases of deformation and metamorphism recorded in different lithologies (Ol-bearing marbles, Bt-bearing gneiss, amphibolites, and boudins of Opx-bearing basic granulites within migmatites) and characterized by contrasting P–T conditions. A weak foliation (Spre2) is locally recognizable at the meso- or micro-scale.
Thermobarometric estimates from Bt-bearing gneiss give estimates of T = 700 ± 50°C and P = 5.7 ± 1 kbar (Fig. 7).
In a nearby area of the Valpelline, Gardien, Reusser & Marquer (Reference Gardien, Reusser and Marquer1994) recognized earlier stages of a high pressure granulite facies evolution in Bt-bearing gneiss, as indicated by kyanite–rutile–alkali feldspar relics in metapelites (700°C at 9.3 kbar).
Stage 2 is characterized by the growth of Grt in basic granulites, thereby producing the stable assemblage Opx + Cpx + Pl + Grt, whereas in metapelites, migmatitic structures largely develop that are associated with diffuse melt-producing reactions. The temperature (810 ± 40°C) and pressure (7 ± 1 kbar) attained during stage 2 (Fig. 7) indicates a > 35–40°C km−1 geothermal gradient.
Stages 3a and 3b correspond to a progressive deformation (S3a and S3b foliations and isoclinal folds) under amphibolite to granulite metamorphic conditions that is well preserved in metabasites and metapelites, where these stages are associated with the production of Grt-bearing melts (metre-thick leucosome bodies and veins). The temperature (800 ± 30°C) and pressure (6 ± 0.7 kbar) attained during stage 3 (Fig. 7) still indicates a high geothermal gradient > 40°C km−1.
The last recorded stage of the evolution is stage 4. Stage 4 is not pervasive, and it is associated with the static growth of Chl and Wm on Bt. This stage occurs under greenschist facies conditions (T = 430 ± 30°C at P = 3.5 ± 0.5 kbar). During this stage, faults and cataclasites also affect all lithologies, as shown for the surrounding rocks of the Arolla unit (Roda & Zucali, Reference Roda and Zucali2008).
7.b. Age constraints and geodynamic implications
Several considerations may be proposed for the pre-Alpine evolution described above.
Stage pre2 reflects a Variscan high thermal gradient, as inferred from abundant basic granulites and Bt-bearing gneiss. Gardien, Reusser & Marquer (Reference Gardien, Reusser and Marquer1994) recognized earlier stages of a high pressure granulite facies evolution that may have recorded the crustal thickening associated with the Variscan collision thickening. The earlier stages (Gardien, Reusser & Marquer, Reference Gardien, Reusser and Marquer1994) and those found in the present study may indicate that separate tectonic subunits were decoupled during the Variscan. The later juxtaposition of these stages would imply either a collisional extrusion of the lower subunit at the end of the Variscan or a Permian extension during lithospheric thinning and continental rifting. Further studies (i.e. a geochronological study of the kyanite-bearing metapelites found by Gardien, Reusser & Marquer, Reference Gardien, Reusser and Marquer1994) are needed to encompass this stage and might help to distinguish between the two hypotheses proposed.
Stages 2 and 3 are characterized by melt-producing reactions and migmatites and by the emplacement of a large amount of melt along veins and dykes. This evolution and the related thermal gradient may be ascribed to the late-Variscan–Permian high thermal event, which is widely reported in the Variscan history of the European continental crust and generally associated with the post-collisional thinning to Permo-Triassic rifting (Weber, Reference Weber, Hutton and Sanderson1984; Lardeaux & Spalla, Reference Lardeaux and Spalla1991; Schuster & Stüwe, 1991; Bonin et al. Reference Bonin, Braendlein, Bussy, Desmons, Eggenberger, von Raumer and Neubauer1993; Müntener & Hermann, Reference Müntener, Hermann, Wilson, Whitmarsh, Taylor and Froitzheim2001). Generally, the intrusion of large igneous bodies across the pre-Alpine continental crust is also associated with this evolution (Bonin et al. Reference Bonin, Braendlein, Bussy, Desmons, Eggenberger, von Raumer and Neubauer1993; Marotta & Spalla, Reference Marotta and Spalla2007; Marotta, Spalla & Gosso, Reference Marotta, Spalla, Gosso, Ring and Wernicke2009 and references therein). In the case of the Valpelline unit, Permian intrusions occur within the adjacent Arolla unit, which is composed of granitic to gabbroic igneous intrusions of Permian age (i.e. Collon and Cervino gabbros: 250 ± 5 Ma, K–Ar, Rb–Sr (Dal Piaz, De Vecchi & Hunziker, Reference Dal Piaz, De Vecchi and Hunziker1977); 284 ± 0.6 Ma, U–Pb (Monjoie et al. Reference Monjoie, Bussy, Lapierre and Pfeifer2005); Arolla granites: 289 Ma (Bussy et al. Reference Bussy, Venturini, Hunziker and Martinotti1998)). Within the Arolla intrusives, several roof pendants and xenolite fragments of various sizes (a few to hundreds of metres in length) were found. These intrusives are also represented by amphibolites, biotite- and garnet-bearing gneisses and acid granulites (Diehl, Masson & Stutz, Reference Diehl, Masson and Stutz1952; Roda & Zucali, Reference Roda and Zucali2008). The lithological similarities between the Arolla xenolites and Valpelline lithotypes (acid granulites, gneisses and amphibolites) along with the high temperature imprint recorded in the described lithotypes suggest that the Valpelline unit is older than the Permian Arolla intrusions (i.e. 250–290 Ma).
Permian magmatism and HT–LP metamorphism affecting the Alpine continental crust have been related to the late orogenic collapse of the Variscan belt (Malavielle et al. Reference Malavielle, Guihot, Costa, Lardeaux and Gardien1990; Ledru et al. Reference Ledru, Courrioux, Dallain, Lardeaux, Montel, Vanderhaeghe and Vitel2001) or as a consequence of lithospheric thinning leading to continental rifting, as proposed for the Austroalpine and Southalpine domains (Diehl, Masson & Stutz, Reference Diehl, Masson and Stutz1952; Lardeaux & Spalla, Reference Lardeaux and Spalla1991; Diella, Spalla & Tunesi, Reference Diella, Spalla and Tunesi1992; Müntener & Hermann, Reference Müntener, Hermann, Wilson, Whitmarsh, Taylor and Froitzheim2001; Schuster & Stüwe, Reference Schuster and Stüwe2008).
The second hypothesis appears more suitable because at the Carboniferous/Permian boundary, the Palaeozoic plate convergence is replaced by a transtensional to extensional tectonic regime, which promoted the break-up of Pangaea (Marotta & Spalla, Reference Marotta and Spalla2007; Schuster & Stüwe, Reference Schuster and Stüwe2008). In the Austroalpine and Southalpine domains, the Permian radiometric ages have been interpreted as representing metamorphic and igneous markers of earlier stages of Mesozoic rifting (Lardeaux & Spalla, Reference Lardeaux and Spalla1991; Quick et al. Reference Quick, Sinigoi, Negrini, Demarchi and Mayer1992; Dal Piaz, Reference Dal Piaz, von Raumer and Neubauer1993; Thoni & Miller, Reference Thoni and Miller2000), whereas the Triassic ages have been interpreted as either minimal ages of thermal pulses during extension-related decompression (Vavra, Schmid & Gebauer, Reference Vavra, Schmid and Gebauer1999) or resulting from a later regional thermal event (Lu et al. Reference Lu, Hofmann, Mazzucchelli and Rivalenti1997). In the Southalpine domain the stratigraphic sequences also document late Carboniferous to Carnian transtension followed by Carnian to Cretaceous rifting to drifting (Gaetani et al. Reference Gaetani, Gnacollini, Jadoul, Garzanti, De Graciansky, Hardenbol, Jacquin and Vail1998; Muttoni et al. Reference Muttoni, Kent, Garzanti, Brack, Abrahamsen and Gaetani2003).
The high thermal gradient and the extensive production and segregation of melt recorded in the metapelites of the Valpelline unit seem to support the high thermal regime associated with the syn-rifting crustal thinning. Dating of accessory phases (allanite, zircon and titanite) in metasediments amalgamated with the gneisses of the Arolla Series, points to Permian metamorphism between ~ 300 and 265 Ma (Manzotti et al. Reference Manzotti, Rubatto, Darling, Zucali, Cenki-Tok and Engi2012). Absolute age data for the Valpelline unit are too sparse and difficult to interpret (Hunziker, Reference Hunziker1974) for a confident constraining of the tectonic evolution. The only age constraints are generally inferred from lithological similarities and structural relationships with adjacent units (e.g. Arolla) or on the basis of lithological–metamorphic comparisons with other units of the Alpine–European chain, which are characterized by similar lithostratigraphic, structural or metamorphic imprints (Spalla & Marotta, Reference Spalla and Marotta2007; Schuster & Stüwe, Reference Schuster and Stüwe2008; Marotta, Spalla & Gosso, Reference Marotta, Spalla, Gosso, Ring and Wernicke2009). Preliminary U–Pb dating on zircon from a pegmatite in the Valpelline unit indicates the Permian period (~ 270 Ma) for the high temperature metamorphism (Zucali et al. Reference Zucali, Manzotti, Diella, Pesenti, Risplendente, Darling and Engi2011). Reliable age data would be crucial to relate the local thermal and mechanical history to the geodynamics at the lithosphere scale during Variscan to Permian times.
The well-preserved structural and metamorphic history of this small portion of the Valpelline basement makes possible a first attempt at an interpretation of the pre-Alpine geodynamics of this unit. An extension of this approach to adjacent portions of the Valpelline unit will help to shed light on the transition between the late orogenic collapse of the Variscan chain and the lithospheric thinning that promoted the opening of the Tethys Ocean.
Acknowledgements
M. Engi and M. I. Spalla are thanked for discussion and reading of early versions of the manuscript; Andrea Risplendente at Microprobe Lab and Agostino Rizzi at SEM (CNR-IDPA) Lab at Dipartimento di Scienze della Terra ‘A. Desio’ – Università degli Studi di Milano are also thanked for technical support. Marco Malusà is thanked for a constructive review.