INTRODUCTION
Despite the small land area, the absence of large land mammals or trees, the presence of active volcanoes, and relentless winds, maritime-adapted hunter-gatherers inhabited the Islands of Four Mountains (IFM) for millennia (Hatfield et al., Reference Hatfield, Bruner, West, Savinetsky, Krylovich, Khasanov and Vasyukov2016). Archaeological investigations have revealed that the Unangax (Aleut people) settled the Fox Islands group, located east of the IFM, ca. 9 ka (Laughlin, Reference Laughlin1975; Davis and Knecht, Reference Davis and Knecht2010) and settled the Andreanof Island group, located to the west of the IFM, by 6–7 ka (O’Leary, Reference O’Leary2001; Savinetsky et al., Reference Savinetsky, West, Antipushina, Khassanov, Kiseleva, Krylovich and Pereladov2012). Thus, a reasonable expectation is that the IFM provided important intermediate waypoints between these larger island groups (Fig. 1A) during initial human colonization of the Aleutians in the early to mid-Holocene (e.g., West et al., Reference West, Crawford and Savinetsky2007). Unexpectedly, the archaeological record of the IFM does not substantiate the direct physical presence of people until ~3.7 cal ka BP (Hatfield et al., Reference Hatfield, Bruner, West, Savinetsky, Krylovich, Khasanov and Vasyukov2016). Yet Kuzmicheva et al. (in Reference Kuzmicheva, Smyshlyaeva, Vasyukov, Khasanov, Krylovich, Okuno, West, Hatfield and Savinetskypress) interpret low stable nitrogen isotopic compositions of soil as evidence of human impact on IFM bird populations by ~6.9 cal ka BP, a time frame for settlement more consistent with expectations raised by well-dated archaeological sites on adjacent island groups. Could the >3000 yr gap between the geochemical and physical records of human habitation result from geomorphic controls constraining landscape stability? We provide the first investigation of environmental change in the IFM attributable to climate-linked geomorphic processes of glacial fluctuation, paraglacial sedimentation, and coastal erosion and link these processes to the IFM archaeological record.

Figure 1 (color online) (A) The Islands of Four Mountains (IFM) are between the Andrienof Islands and the Fox Islands in the central Aleutians. The pioneering work of R. Black (black dots) focused on the western part of Umnak, shown here, particularly near the prehistoric sites of Chaluka and the Anangula Blade site, as well as the currently inhabited village of Nikolski. (B) In the IFM, Chuginadak Island is composed of the Cleveland and Tana (black outlines are the extents of Fig. 3A and 4A5) volcanoes, which are connected by a narrow isthmus. Carlisle volcano (black outline extent of Fig. 3) is located to the north of Cleveland. This work focused primarily on Carlisle Island and Tana volcano.
Two important external controls on human settlement patterns are climate and geomorphic processes. They influence when human settlements are established and where they are located. This is particularly important during the Late Anangula phase (7000–4000 yr BP) when Aleutian human occupation styles converged on the use of semi-subterranean houses (Davis and Knecht, Reference Davis and Knecht2010). Changes to either climate or geomorphic processes also affect when settlements are abandoned and how well abandoned settlements are preserved. Climate influences the habitability of maritime settlement locations directly by temperature, wind, and precipitation. Climate also indirectly influences locations by the frequency and magnitudes of stream discharge and mass movements. Because of the small size of the IFM, there are few perennial water sources, seemingly a key resource in the location of mid- and late Holocene village sites in the Aleutian Islands (Corbett, Reference Corbett1991; Frohlich, Reference Frohlich2002). Climate change may also trigger ecosystem changes that affect human settlement and foraging patterns such as types and abundances of food resources (e.g., Savinetsky et al., Reference Savinetsky, West, Antipushina, Khassanov, Kiseleva, Krylovich and Pereladov2012).
Geomorphic processes influence both the spatial distribution and stability of landforms suitable for human settlements (Rapp and Hill, Reference Rapp and Hill2006) and are key factors contributing to the preservation and visibility of the archaeological record (Mandel, Reference Mandel1995). The Aleutian Islands, with their limited low-gradient terrain for settlement and complex dynamics of sedimentation and erosion are no exception. In the Aleutian Islands, the growth and retreat of glaciers and sea level fluctuations are recognized as important factors on human migration (Mason and Jordan, Reference Mason and Jordan2002; Misarti et al., Reference Misarti, Finney, Jordan, Maschner, Addison, Shapley, Krumhardt and Beget2012) and settlement locations (Black, Reference Black1976; Winslow and Johnson, Reference Winslow and Johnson1989). Determining changes to the complex coastal configuration in this geologically dynamic region is important to understanding how well the archaeological record represents human choices (Winslow, Reference Winslow1991; Jordan, Reference Jordan2001). Unexplored potential factors influencing human settlement dynamics in the Aleutians are high rates of erosion and sedimentation associated with paraglaciation. Landscapes experience paraglacial instability following glacial retreat. In mountainous regions, instability is the result of oversteepened hillslopes of glacial sediment and bedrock that become prone to rockfall and debris flows that transport voluminous material to valley bottoms (Church and Ryder, Reference Church and Ryder1972). The duration and spatial variability of paraglacial denudation and sedimentation has not been well documented in the Aleutian Islands, leading to uncertainty as to the relative importance of paraglaciation in overall island morphology and potential influence on human occupation. Ballantyne (Reference Ballantyne1992) suggests that landscape adjustment to paraglacial conditioning overwhelms fluvial systems for millennia, and in volcanic landscapes, the effects following the last glacial maximum (LGM) may persist throughout the early Holocene (Friele and Clague, Reference Friele and Clague2009; Cossart et al., Reference Cossart, Mercier, Decaulne and Feuillet2013, Reference Cossart, Mercier, Decaulne, Feuillet, Jonsson and Sæmundsson2014). Furthermore, hydrothermal alteration, common in the Aleutians (e.g., Hildreth et al., Reference Hildreth, Fierstein, Lanphere and Siems2001), may also increase rates of weathering and erosion (Gaillardet et al., Reference Gaillardet, Louvat and Lajeunesse2011). Paraglacial sedimentation associated with both the LGM (28–9 ka in Alaska; Kaufman et al., Reference Kaufman, Young, Briner and Manley2011) and Neoglaciation (3–4 ka in Alaska; Barclay et al., Reference Barclay, Wiles and Calkin2009) would have influenced the landscape conditions encountered by people as they initially established village settlements in the Aleutian Islands.
The IFM island group includes, from west to east, Seguam, Chagulak, Yunaska, Herbert, Carlisle, Chuginadak, Kagamil, and Uliaga Islands (Fig. 1A). Evidence for glaciation in the IFM, however, has never been investigated in the field. Our observations and measurements from the 2014 and 2015 multidisciplinary expeditions focused primarily on the volcanic peaks of Carlisle, Cleveland, and Tana, the latter two forming Chuginadak Island (Fig. 1B). The islands have experienced Quaternary volcanism and mass wasting; the products of these geologic processes form the deposits that flank the peaks and provide flat surfaces suitable for human settlement. We investigate the hypotheses that the islands were extensively glaciated at the LGM, that paraglacial processes contributed to fan development, and that hydrothermal alteration creates heterogeneity in landforms and rates of paraglacial processes. We also investigate whether these geomorphic processes have affected human settlement patterns.
BACKGROUND
Climate in the IFM
The oceanic climate of the Aleutian Islands provides abundant rain during the summer and snow in the winter (mean annual precipitation = 1072 mm; Umnak Island). During winters, the climate is significantly influenced by the location of the Aleutian low, a stationary low-pressure system often centered on the Aleutians. The thermal mass of the Pacific Ocean moderates temperature variability in the Aleutians with an ~8°C difference between summer and winter (mean annual temperature = 1.3°C). Despite the relatively warm temperatures, abundant rainfall, and fertile soil, the IFM are treeless because of the persistent strong winds. The vegetation of islands consists of dense shrubs including crowberry (Empetrum nigrum), grasses (Poaceae), and sedges (Cyperaceae) (e.g., Kuzmicheva et al., in Reference Kuzmicheva, Smyshlyaeva, Vasyukov, Khasanov, Krylovich, Okuno, West, Hatfield and Savinetskypress).
Holocene climate conditions in the Aleutian Islands were variable, and paleoclimatic records are inconsistent with respect to the magnitude of well-known climate periods. For example, the Holocene climatic optimum (a globally recognized warm period 9–5 ka) is documented in some areas and not in others in the Aleutians (Heusser, Reference Heusser1973, Reference Heusser1990; Mann et al., Reference Mann, Crowell, Hamilton and Finney1998; Lozhkin et al., Reference Lozhkin, Anderson, Eisner and Solomatkina2011). During the late Holocene, cooler sea surface temperatures associated with the Neoglacial period may have allowed sea ice to form in some areas of the eastern Bering Sea (Caissie et al., Reference Caissie, Brigham-Grette, Lawrence, Herbert and Cook2010), including the Fox Island group. Seasonal sea ice would have direct impacts on the distribution of marine mammals and other marine fauna and, therefore, on the subsistence economies and foraging patterns of Aleutian hunter-gatherers. In Unalaska Bay in the Fox Island group, occupations dated between ~4.5 ka and 3 ka document the use of ice-obligate species such as walrus and ringed seals alongside the remains of temperate species such as Steller sea lions (Eumetopias jubatus), fur seals (Callorhinus ursinus), and harbor seals (Phoca vitulina) that are typical of later Aleutian subsistence economies (Davis, Reference Davis2001; Crockford and Frederick, Reference Crockford and Frederick2007; Crockford, Reference Crockford2012). The presence of cold-tolerant Saffron cod (Eleginus gracilis) in an archaeological context dated to ~7 ka, and diatom assemblages from peat deposits on Adak Island suggest that the central Aleutians experienced cold conditions during the initial mid-Holocene (Savinetsky et al., Reference Savinetsky, West, Antipushina, Khassanov, Kiseleva, Krylovich and Pereladov2012).
Glaciation in the Aleutians
At the LGM, the Cordilleran Ice Sheet extended into the both the Alaska and Aleutian ranges. Most of the Alaska Peninsula, adjacent continental shelf, and some extent of the Aleutian Islands were covered in ice (Kaufman et al., Reference Kaufman, Young, Briner and Manley2011). The Fox Island group, including the large islands of Unalaska and Umnak, were extensively glaciated with large ice caps that covered the volcanoes and extended across the Aleutian platform (Thorson and Hamilton, Reference Thorson and Hamilton1986; Kaufman and Manley, Reference Kaufman and Manley2004). The limited geologic mapping of glacial deposits is a primary constraint on interpreting the extent of glaciation in the central and western Aleutians (Thorson and Hamilton, Reference Thorson and Hamilton1986; Kaufman et al., Reference Kaufman, Young, Briner and Manley2011).
There is disagreement as to the timing of deglaciation throughout the Aleutians. The deglaciation of Sanak Island and retreat of the Alaska Peninsula Glacial Complex from the southeastern portion of the continental shelf was complete by ~17 ka (Misarti et al., Reference Misarti, Finney, Jordan, Maschner, Addison, Shapley, Krumhardt and Beget2012). The low elevation of Sanak (~530 m) and its proximity to the Alaska Peninsula suggests that the deglaciation histories of Recheshnoi (1984 m) and Vsevidof (2149 m) volcanoes on Umnak Island provide better models for understanding the glacial history of the IFM. On Umnak, deglaciation is based on organic matter at the base of tephra and soil sequences that mantle till deposits (Byers, Reference Byers1959; Black, Reference Black1974). Black (Reference Black1976) interpreted these 6–10 ka ages as providing close constraints on the timing of deglaciation. His chronology is corroborated by vegetation records on Umnak and Unalaska Islands that indicate the formation of peat deposits ca. 10 cal ka BP and by ages of initial human settlement of the Fox Island group ca. 9 ka (Laughlin, Reference Laughlin1975; Davis and Knecht, Reference Davis and Knecht2010). These ages, however, are significantly younger than deglaciation in the Gulf of Alaska, 12–15 cal ka BP (Molnia, Reference Molnia1986). Thorson and Hamilton (Reference Thorson and Hamilton1986) suggest that this discrepancy may be the result of an erosional unconformity between glacial till and younger soil sediments. Alternatively, the early Holocene persistence of glaciers in the maritime Aleutians may result from wetter winter conditions (Hu et al., Reference Hu, Brubaker and Anderson1995) caused by the development of a secondary, low pressure system in the Gulf of Alaska, which in turn produced more sea-ice cover during the early Holocene (Katsuki et al., Reference Katsuki, Khim, Itaki, Harada, Sakai, Ikeda, Takahashi, Okazaki and Asahi2009).
The onset and magnitude of the Neoglacial period in the Aleutians is also poorly constrained (Black, Reference Black1976; Barclay et al., Reference Barclay, Wiles and Calkin2009; Lozhkin et al., Reference Lozhkin, Anderson, Eisner and Solomatkina2011; Savinetsky et al., Reference Savinetsky, West, Antipushina, Khassanov, Kiseleva, Krylovich and Pereladov2012). Even if Aleutian conditions remained relatively cool and wet through the early and mid-Holocene (Caissie et al., Reference Caissie, Brigham-Grette, Lawrence, Herbert and Cook2010), there was increased terrestrial precipitation and glacial advance associated with strengthening and repositioning of the Aleutian low over the Aleutian Islands during the Neoglacial (Calkin et al., Reference Calkin, Wiles and Barclay2001; Katsuki et al., Reference Katsuki, Khim, Itaki, Harada, Sakai, Ikeda, Takahashi, Okazaki and Asahi2009; Lozhkin et al., Reference Lozhkin, Anderson, Eisner and Solomatkina2011). Throughout the Aleutians, glaciers advanced during the Neoglacial, ~4.5–2.7 ka (Calkin et al., Reference Calkin, Wiles and Barclay2001; Barclay et al., Reference Barclay, Wiles and Calkin2009; Badding et al., Reference Badding, Briner and Kaufman2013). During this advance, glaciers progressed 1–2 km downvalley of current glacier termini (Detterman, Reference Detterman1986; Thorson and Hamilton, Reference Thorson and Hamilton1986). End moraines on the flanks of Recheshnoi (1984 meters above sea level [m asl]) and Vsevidof (2149 m asl) volcanoes on Umnak Island occur 1–2 km downvalley from current glacial termini (Byers Reference Byers1959) and, in two locations, are dated to ~3 ka (Black Reference Black1974). These Neoglacial advances did not reach the coastline, and therefore, did not have a direct impact on human groups. Rather, glacial runoff streams likely provided an abundant supply of freshwater for Unangan settlements (Black, Reference Black1974).
In the Aleutians and elsewhere in Alaska, Little Ice Age (LIA) moraines are documented near current glacier termini (Barclay et al., Reference Barclay, Wiles and Calkin2009). There was a significant LIA advance at ~AD 1750, though recent observations, in conjunction with maps from the early to late AD 1900s, suggest that glaciers are retreating rapidly (Molnia, Reference Molnia2008). Recent glacial activity has been monitored by satellite surveys since the advent of Landsat in 1971; Molnia (Reference Molnia2008) found that at least 10 islands in the central and eastern portion of the Aleutian Islands contain glaciers. Importantly, there is no documented presence of glaciers in the IFM, save for a single reference to a small glacier in the caldera of Herbert Island (Molnia, Reference Molnia2008). Unimak Island has glaciers on the southwest part of the island on Pogromni Volcano, Faris Peak, and Westdahl Peak. In Unalaska, glaciers are present on the flanks of Makushin Volcano. On adjacent Umnak Island, nine glaciers flowing off of Mounts Vsevidof and Recheshnoi were observed in 1959 and 1977. A significant amount of retreat is recorded throughout the Aleutians, but the exact amount and timing is not documented (Molnia, Reference Molnia2008).
On islands adjacent to the IFM, glaciers usually cap volcanic peaks where summit elevations are about 2000 m asl and have more ice accumulation on northern quadrants. Within the Okmok (Umnak Island) and Herbert calderas (in the IFM) glacial ice is concentrated on the north-facing walls (Molina, 2008). In the 1950s, glaciers on Mounts Vsevidof and Recheshnoi extended farther downslope on the north side (to as near as 60–90 m asl) than the south (150–300 m asl; Byers, Reference Byers1959). Northern slopes also have more ice if there are topographic lows such as valleys that increase the drainage area of the slope. These can be produced by sector collapse and preferential erosion of weaker, hydrothermally altered rock or unconsolidated deposits (e.g., Reid et al., Reference Reid, Sisson and Brien2001). For example, on Snowy Mountain (on the Alaska Peninsula), a massive late-Holocene sector collapse destroyed part of its summit where hydrothermal alteration of lava to clay had previously weakened the edifice. This resulted in the accumulation of snow and ice, which developed into a glacier, furthering eroding the hydrothermally altered volcano (Hildreth et al., Reference Hildreth, Fierstein, Lanphere and Siems2001).
Volcanic history of the Carlisle, Cleveland, and Tana volcanoes
Carlisle Island consists primarily of a single composite volcano of conical shape (elevation 1620 m; Fig. 1B). Although some early historical sources reported eruptions from Carlisle volcano, Miller et al. (Reference Miller, McGimsey, Richter, Riehle, Nye, Yount and Dumoulin1998) concluded in fact that many of these may have mistaken the identity of Mount Cleveland volcano for Carlisle. Given that their summits are separated by 10.6 km and that fog and low clouds obscure both frequently, the reports of Holocene volcanic activity of Carlisle volcano require further substantiation. A mid–late Holocene peat bog on the northeast quadrant of Carlisle Island, located ~5 km from the summit, (~10.7 km north of Cleveland) contains two significant tephras, one a lapilli fall ~1050 cal yr BP and one fine-grained ash likely from the eruption of Okmok caldera ~2000 cal yr BP (Okuno et al., Reference Okuno, Izbekov, Nicolaysen, Sato, Nakamura, Savinetsky and Vasyukov2017). Based on glass chemistry and isopach distribution, the ~1050 cal yr BP lapilli tephra is interpreted as an eruption of Cleveland volcano (M. Okuno and P. Izbekov, personal communication, 2017). An ~18-cm-thick lapilli tephra near the base of the bog was deposited ~6030 cal yr BP, but it lacks attribution to a specific volcanic vent because extant field observations are not conclusive. In sum, no clear evidence of proximal deposits from Carlisle volcano was observed in the mid–late Holocene stratigraphically intact bog profile. Nonetheless, two circular pits at Carlisle’s summit may indeed be explosion craters from late Holocene or historic eruptions (Waythomas, Reference Waythomas2015). Twenty-eight samples of lava flows and tephras from Carlisle indicate that the bulk of the rock is basaltic andesite to dacite in composition (56.3–64.4 wt% SiO2) with the exception of a single primitive mafic enclave (44.8 wt% SiO2; K. Nicolaysen and P. Izbekov, unpublished).
Chuginadak is composed of two volcanoes—the conical Mount Cleveland (1730 m asl), which is connected by a narrow isthmus to the highly dissected Tana volcano (1170 m asl; Fig. 1B). In contrast to Carlisle volcano, the evidence of frequent historic and prehistoric eruptions of Cleveland volcano is incontrovertible (Dean et al., Reference Dean, Dehn, Papp, Smith, Izbekov, Peterson, Kearney and Steffke2004; Pekar et al., Reference Pekar, Nicolaysen, Bridges and Dehn2005; De Angelis et al., Reference De Angelis, Fee, Haney and Schneider2012; Neal et al., Reference Neal, Izbekov and Nicolaysen2015; Okuno et al., Reference Okuno, Izbekov, Nicolaysen, Sato, Nakamura, Savinetsky and Vasyukov2017; Werner et al., Reference Werner, Kern, Coppola, Lyons, Kelly, Wallace, Schneider and Wessels2017). Miller et al. (Reference Miller, McGimsey, Richter, Riehle, Nye, Yount and Dumoulin1998) did not include Tana in their 1998 Catalog of the Historically Active Volcanoes of Alaska, because the earliest historical references omit mention of volcanic activity, and there has been no activity observed by satellite. There are documented active hot springs, fumaroles, and mud pots on the north and northeastern flanks of Tana, and initial sampling reveals a range of pH and temperatures (Evans et al., Reference Evans, Bergfeld, Neal, McGimsey, Werner, Waythomas and Lewicki2015) suggesting that volcanic activity of Holocene age is likely. Tana has several dendritic drainage basins not present on the other islands, the largest (basin area: ~8 km2) of which is located on the eastern flank of Tana (Fig. 2).

Figure 2 (color online) (A) Landsat 8 imagery of the Tana volcano was collected on November 19, 2017. (B) Red, Green, and Blue (RGB) composite (6,5,4) and band ratios (7/4, 6/5, 5/3) were used for image classification. Exposed soil and hydrothermally altered bedrock are exposed on the southern side of the island, especially in the east Tana valley. (C) An RGB composite (5,4,3) of a November 19, 1975, Landsat 8 image compared with similar bands (3,2,1) from a November 25, 1975, Landsat 2 image (D) of the Tana volcano highlights the variability in yearly snow cover on the Islands.
METHODS
Our team focused its investigation on Carlisle and Chuginadak Islands, because the most extensive investigation of the local human settlement history is from these islands (O’Leary, Reference O’Leary1993a, Reference O’Leary1993b; Hatfield et al., Reference Hatfield, Bruner, West, Savinetsky, Krylovich, Khasanov and Vasyukov2016). Fieldwork was split into two field seasons during the summers of 2014 and 2015. We report observations from several quadrants on the Carlisle, Cleveland, and Tana volcanoes; most of our detailed descriptions and measurements focused on the large eastern drainage of Tana and a prominent sediment fan on Carlisle. The term “sediment” is preferred to “alluvial” because the fans comprise a variety of sediment types including debris flow, mudflow, fluvial, cultural, till, and tephra (Derbyshire and Owen, Reference Derbyshire and Owen1997). Two village sites investigated by our team in 2014 and 2015, one on Carlisle Island (AMK003) and one on Chuginadak Island (SAM014), are located on sea cliffs where coastal processes eroded coves into the base of unlithified fan deposits. In each case, the earliest occupation levels are directly above debris flow deposits and date to ~3700 cal yr BP (Hatfield et al., Reference Hatfield, Bruner, West, Savinetsky, Krylovich, Khasanov and Vasyukov2016).
During the 18-day field season in 2014, we sampled and measured the stratigraphy of two sediment fans: one on Tana and one on Cleveland. The sediment fan on Tana is located at a village site, and we investigated the transitions between debris flow, tephra, alluvial, and cultural deposits. Because of time constraints, the stratigraphy of the sediment fan on Cleveland was described, but thickness measurements were not made. Descriptions of a sediment fan on Carlisle were conducted at the location of a village site and upgradient near the head of the fan during the 16-day field season in July–August 2015. We obtained sediment samples of the thickest units of the Tana and Carlisle fans and of overlying stream overbank and human influenced deposits. All samples were sieved to ≤2 mm and investigated for grain-size analysis using a Mastersizer at Central Washington University.
During 2015, our team discovered, described, and mapped the first reported glaciers and moraines on Tana volcano and investigated solifluction terraces and other paraglacial features. To characterize the role of rock type in shaping landform morphology, we surveyed moraines on both Carlisle and Tana (Figs. 3–6). We used a Trimble Geo 7x GPS (vertical accuracy ~0.5 m) to collect GPS points and lines of well-defined lateral, medial, and terminal moraines. In the east Tana valley, in what we called the proximal moraine complex, a group of well-defined moraines within a half kilometer of the current glacier terminus were mapped in detail (Fig. 4). We mapped the crests of the moraines; if the moraine crests were not well defined, the top of the moraine was estimated. We surveyed cross sections of sharp-crested moraines composed primarily of unaltered dacite and more rounded moraines composed of heavily hydrothermally altered rock (Fig. 5). Additionally, we surveyed the current glacier terminus for future studies. A suite of moraines with more rounded crests and large solifluction lobes were also surveyed 4 km downvalley (Fig. 6). The surveys were imported into ArcGIS and interpreted in conjunction with the recently released ArcticDEM (~0.5 m resolution; Fig. 6). ArcticDEM was also used to characterize lava flow and fan morphology. Landsat 2 and 8 (Fig. 2) imagery was used to identify regions of hydrothermal alteration and perennial snow cover. Bathymetry in the IFM (Zimmermann et al., Reference Zimmermann, Prescott and Rooper2013) was joined with SRTM (Shuttle Radar Topography Mission) elevation data to estimate changes in land area of the islands attributable to changes in sea level.

Figure 3 A prominent sediment fan on the southeastern flank of Carlisle (A) is the location of a prominent village site, Ulyagan. A perennial stream (blue solid line) is located 0.5 km from the village. Near the village, an ephemeral stream (blue dotted line) has incised 4 m into the fan surface (B). The stream was likely abandoned by channel avulsion caused by a debris flow or landslide near the head of the fan (E, F). The perennial stream has incised the fan surface providing fresh exposures of the stratigraphy (black dot in panel A, Fig. 9). The distal portion of the fan is eroded by wave action along the coast. There has been 300–500 m of erosion (D). There is evidence for both glacial erosion and deposition on Carlisle. Lava flows are extensively modified by glacial erosion including a dissected plug at the summit (C). Flows contain vertical fractures (G) uncommon to fresh unmodified lavas that flank the north side of Cleveland. Black lines with circles are mapped moraine crests. Moraines are sharp crested (F, H) and lodgment till (I) preserved at high and low elevations on the island (blue dots in panel A). Glacial till deposits on Carlisle are coarse grained and contain abundant angular clasts. (For interpretation of the references to color in this figure legend, the reader is referred to the web version of this article.)

Figure 4 (A) Digital elevation model and shaded relief of the east Tana valley. Black lines with circles are moraines. Red line is current glacier terminus visible in oblique imagery of the east Tana valley (E). Two sets of moraines that close the terminus of the glacier are more sharp crested (B) than moraines farther downvalley (C and D). Bedrock is extensively altered in the east Tana valley, and clasts are susceptible to frost shattering (F), and recently deglaciated slopes are loaded with highly erodible material (G). (For interpretation of the references to color in this figure legend, the reader is referred to the web version of this article.)

Figure 5 Moraine morphology in the east Tana valley. Cross sections are from differential global positioning systems (DGPS) survey and are higher resolution (~0.5 m) than the ArcticDEM (2 m) cross sections in Figure 4. The diffusion constant is an order of magnitude greater in the moraine composed of hydrothermally altered material.

Figure 6 Oblique aerial photograph of Neoglacial moraine in the east Tana valley (A); view is oriented west–northwest and documents abundant paraglacial deposits. The unvegetated recently deglaciated upper basin produces voluminous sediment that is deposited in fill terraces (A). Steep slopes of exposed glacial sediments are also actively eroding into the bottom of the valley. Moraines are eroding by solifluction where orange patches are actively moving and thus vegetation cannot colonize (A). The moraine is composed of hydrothermally altered material with abundant clay and iron oxides (B). GPS surveys of solifluction lobes indicated substantial diffusion of material. The risers of the solifluction lobes range 0.3–0.8 m, and the treads are 5–10 m in length (C). (For interpretation of the references to color in this figure legend, the reader is referred to the web version of this article.)
Hydrothermal alteration is apparent in the east Tana valley (Figs. 2B and 4–6). To assess the mineral alteration and the effects on moraine morphology, we collected till samples from two moraines in the upper Tana valley that showed visual, textural, and mineralogical differences (Fig. 7). To characterize the effect of rock type on moraine morphology, we selected moraines of different ages and lithologies in the east Tana valley. We sampled two older moraines: one composed primarily of unaltered dacites and high silica andesites and another composed of heavily hydrothermally altered dacites and high silica andesites. The younger inset moraines are composed of extensively hydrothermally altered rock. One sample was composed primarily of altered rocks, and the second sample had minimally altered lavas. We also sampled a hydrothermal breccia preserved in till. This sample was cut and thin sectioned for study with a petrographic microscope, a FEI Quanta 250 environmental scanning electron microscope (SEM) at Whitman College. The nature of the clays in the tills was determined using an X-ray diffractometer (XRD) (Table 1). The spatial variability of alteration was documented using Landsat imagery at lower elevations where snow, ice, and shadows did not cover the surface (Fig. 2B).

Figure 7 (color online) (A) Rounded Little Ice Age moraine composed of altered volcanic rock in the center of the east Tana valley. (B) Scanning electron microscope analyses of a clast from this moraine indicate secondary minerals rich in aluminum. (C) Photomicrograph of the same clast indicating significant amounts of altered clay-rich material.
Table 1 Comparison of the minerality of clasts from rounded moraines composed of hydrothermally altered material and sharp crested moraines composed of unaltered rock.

a Mineral abundance determined by X-ray diffraction.
b All values expressed as weight percent.
RESULTS
Morphology of the Islands
Carlisle Island is approximately circular with a radius of ~3 km and with five notable protrusions where lava flows protect overlying, unconsolidated volcaniclastic deposits. Snowfields fill valleys up to the summit (1590 m); however, at the time of our field investigations, summer melt had removed all snow at the summit by mid-August of 2014. With the exception of a satellite vent on the west flank, the topography of the island suggests that most eruptions emanated from the summit vent and that the main composite cone covered earlier vents. Field observations of Carlisle’s summit (Fig. 3C) show a pale exposed plug that appears to be a silicic summit dome from which surrounding material has eroded. In general, unconsolidated deposits at the surface include distal fine-grained tephras from adjacent volcanoes, and tills, colluvium, and debris flows sourced from geomorphic processes on Carlisle itself (Fig. 3). Notably on the west flank of Carlisle, solifluction terraces display lichen-covered pebble- to cobble-sized vesicular clasts and rare centimeter-sized pieces of relatively fresh gray pumice interpreted to be from the AD 2001 eruption of Mount Cleveland.
The two volcanoes that comprise Chuginadak Island are morphologically distinct. Tana volcano is 6 km wide and has a summit elevation of 1180 m. The complex morphology of the Tana volcano results from multiple eruptive centers including a small summit caldera containing a lake, a young eruptive fissure and two cinder cones on its west flank, and, to the south, multiple lava-dike sequences of Black Peak, a small volcano halved by landslides and coastal erosion. A broad, flat platform, with lavas exposed in sea cliffs, extends from the southern side of the island. The largest valley on the island is a 1.3 km wide U-shaped trough on the eastern margin. The western side of Chugindak Island is the conical and symmetrical Cleveland volcano (elevation = 1721 m). There are undissected lava flows with preserved levees on the northeastern and eastern flanks. There are recent volcanic debris flows on the west and south flanks and at least five distinct satellite vents including three volcanic domes (south and southeast) and a prominent cinder and spatter cones (west and east). The summit slopes are nearly undissected, and the lower flanks are more irregular with some dissection.
Glacial features
There is both erosional and depositional evidence of glaciation of the islands. Along the northeastern coast of Carlisle, a narrow ~1.5-km-long peninsula has preserved quenched and invasive lava structures including pseudopillow fracture and platy joints that suggest an ice-contact environment (Conway et al., Reference Conway, Townsend, Leonard, Wilson, Calvert and Gamble2015). This observation suggests island-wide ice cover during the Pleistocene. More evidence for complete glaciation of Carlisle includes lodgment tills on the southeast and western flanks (Fig. 3), linear ridges of eroded lava flows (Fig. 8), U-shaped valleys, and poorly polished andesite with vertical fractures consistent with glacial unloading (Cossart et al., Reference Cossart, Mercier, Decaulne, Feuillet, Jonsson and Sæmundsson2014).
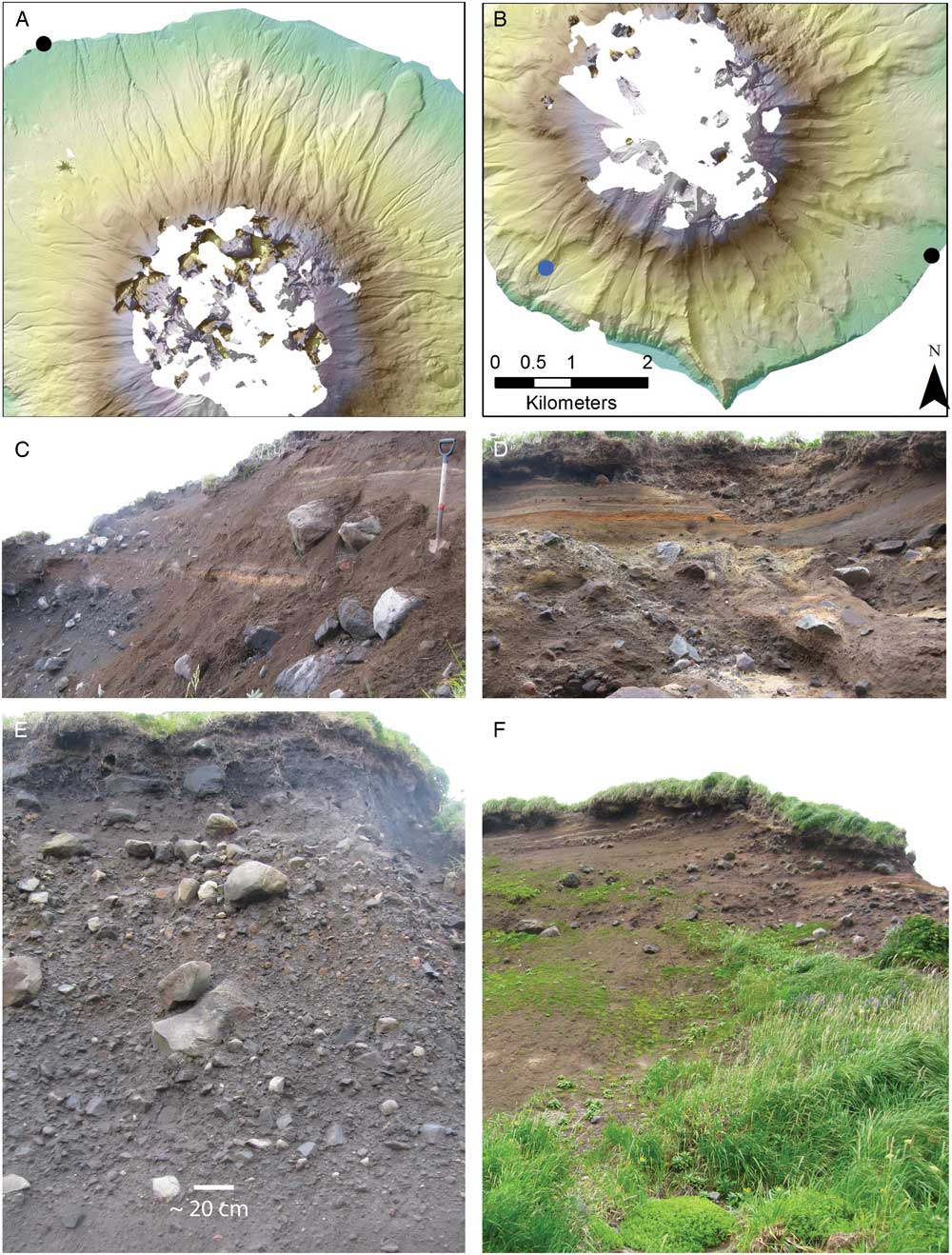
Figure 8 Comparison of the morphology and sediment fan stratigraphy of the Cleveland (A) and Carlisle (B) volcanoes. On Cleveland, there are many unmodified lava flows with preserved lava levees. On Carlisle, the volcano flanks are highly dissected, and linear ridges are the result of glacial erosion of lava flows. A single unmodified lava flow (blue circle) is apparent on the southwest flank of Carlisle. Comparison of the stratigraphy of coarse-grained deposits on the Cleveland (C, E) and Carlisle (D, F) volcanoes. The sediment fan on Carlisle (black dot in panel B) is composed of dominantly coarse-grained debris flow deposits mantled with fine-grained alluvium, tephra, and cultural materials. The stratigraphy of debris flow deposits on the more active Cleveland volcano is different. A beach cliff (black dot in panel A) on the north flank of Cleveland volcano exposes ~30 m of block-and-ash flows, debris flows, and tephras. (For interpretation of the references to color in this figure legend, the reader is referred to the web version of this article.)
Chugindak Island also has many glacial features. The large eastern valley contains a glacier located at the top of the 5-km-long valley that drains to the south/southeast. Tana Glacier, here described and named, is currently composed of three lobes (Fig. 5). The south lobe is located on the south side of the cirque wall, having a bergschrund located a quarter of the way up the cirque wall. This lobe of Tana Glacier has well-defined recessional moraines. The central lobe of the Tana Glacier is situated in the middle of the cirque; its current terminus extends farthest downvalley. The third lobe is located in the north end of the cirque, with a bergschrund located halfway up the cirque wall. The full extent of this lobe is difficult to determine because of seasonal snow cover, yet it is responsible for numerous moraines on the north side of the valley. Small cavities in the cirque headwall, in addition to separate moraine sequences, suggest that former lobes of the Tana Glacier have receded very recently. Currently, the ice covers an area of approximately 0.1 km2.
Extensive glaciation has dissected the Tana volcano as demonstrated by cirques, sculpted lava flows, arêtes, and U-shaped valleys. Five valley headwalls have well-defined cirque morphology (Figs. 2 and 4). There is evidence for solifluction and landsliding that have altered the morphology of moraines and other glacial features. Solifluction lobes are observed on slopes and moraines, some with ~1 m risers and ~3-m-long treads (Fig. 6).
Sediment fan and debris apron stratigraphy
On the north flank of Tana, sediment fans and debris aprons are exposed in sea cliffs with 20–30 m of relief (Fig. 9) and host at least one prehistoric village site. The majority of the cliffs expose thick sections of debris flow deposits. The debris flow deposits are composed of angular and subangular clasts of mixed rock types whose surfaces show varying degrees of hydration and alteration. The fine fraction of the deposits is dominated by poorly sorted sands and displays a broad peak in grain-size distribution (Fig. 8). These deposits are likely nonvolcanic in origin. There are also a few thinner volcanic debris flows, which are characterized by angular and subangular clasts of unweathered black lava clasts in the coarse fraction; these consist primarily of coarse sand in the fine fraction. The top of the section is mantled by laterally continuous, fine-grained tephras and by overbank deposits. The fine fraction of the cultural, tephras, and overbank deposits are finer than for the debris flow and have clay- to silt-sized matrices (Figs. 8 and 9).

Figure 9 Debris apron stratigraphy on Tana. (A) The debris fan on the north flank of the Tana volcano. Most of the layers are matrix supported and contain subrounded to subangular boulders in a brown matrix. The boulders are up to a meter in diameter and display more than one lithology. Thinner lenses of mud, gravel, and pebble-sized clasts are likely hyperconcentrated flow deposits. (B) The median particle size of the debris flow deposits is coarser than the ash and cultural layers.
A sediment fan on the southeastern flank of Carlisle, nearest to Cleveland volcano, is the location of a prehistoric village site (Fig. 8). An extensive vertical sequence of fine-grained tephras and soils overlies debris flow deposits. The majority of the fan thickness is composed of coarse-grained debris flow deposits. The deposits are composed of angular to subrounded clasts of mixed rock types. The matrix of the deposits is mixed fine-coarse sand and gravel. Similar to Tana, these deposits are likely nonvolcanic origin. Fine-grained layers of tephra, overbank, and cultural deposits mantle the debris flow deposits, similar to the Tana stratigraphic section. The fine-grained deposits are thickest at the distal portion of the fan and thin at the head of the fan (Fig. 10). Within the upper sequence of tephras there are two thinner debris flows. This sequence does not show evidence of proximal tephras definitively sourced from Carlisle, and many exposed tephras may derive from Cleveland (Neal et al., Reference Neal, Izbekov and Nicolaysen2015). Near the village site, the stream has incised 3 m in to the fan surface, and a fill-cut terrace is preserved. The perennial stream on the fan has incised ~5 m near the head of the fan (Fig. 3). The sequence of deposits is similar to the village site; however, the thickness of the fine-grained layer is less and confined to a scour channel (Fig. 10).

Figure 10 On the Carlisle fan, the fine-grained layers are thicker near the toe of the fan, which is where cultural material has been identified. Near the head of the fan fine-grained layers are preserved in scour channels of coarse-grained deposits. The fine-grained layers are composed of overbank and tephra deposit. The underlying coarse-grained deposits are matrix-supported polymicts that are likely the result of debris flows that are sourced to the steep flanks of Carlisle.
A sediment fan on the east flank of Cleveland is exposed at the shoreline (Fig. 8). Just above the break in slope at the beach, the fine-grained ashy matrix contains homogeneous blocks of dark lava with curving fractured faces meeting at sharp angles, likely a volcanic debris flow deposit. The overlying debris flow has a matrix that is browner and contains multilithologic blocks that coarsen upward boulders up to a meter in diameter. The change in matrix color and an increasing proportion of blocks showing hydrothermal alteration signals a second depositional event though the boundary is diffuse. This deposit is interpreted as debris flow deposits of nonvolcanic origin. Near the top, the slope steepens because of an increase in cohesive fine-grained material. The lower layer has a paler ash matrix and a relatively distinct contact with an 8-m-thick unit of dark-gray ashy matrix, monolithologic blocks that are typically clast-supported and contain plagioclase-phyric glassy andesite. Blocks are 10–60 cm and have jointing consistent with origination from a volcanic dome collapse (i.e., mafic-intermediate block-and-ash flow). The next layer in the stratigraphy is a ~1-m-thick tephra of fine ash to coarse lapilli overlain by a 1-m-thick probable block-and-ash flow. Above this is an 11-cm-thick sequence of fine tephras that range from a fine-medium lapilli tephra with reddish scoria to a yellow layer with orange pumiceous lapilli that fines upward to a buffy ash. The next in sequence is a thin (~35 cm) debris flow with multilithologic, subrounded boulders. The remainder of the ~4-m-thick section is a sequence of ashes and tephras that range from 3- to 30-cm-thick until the uppermost 75 cm of brownish soil. This sequence on the north flank of Cleveland has considerably more layers to which a volcanogenic origin may be attributed, and some of these are quite coarse, in keeping with the proximity to a vent with frequent Holocene activity. The Cleveland sedimentary sequence is very different relative to the sediment sequences exposed on the north flank of Tana and southeastern flank of Carlisle.
Hydrothermal alteration
Classified Landsat data indicate that much of the exposed bedrock in the east Tana valley is hydrothermally altered (Fig. 2). The moraine on the southern margin of the valley is composed of less altered rock and has a relatively sharp crest mantled with dark volcanic clasts and a dark brown-yellow matrix of the till (Figs. 4A and 7; sample HLT-1). Moraines in the center of the valley are composed entirely of hydrothermally altered rock and have rounded crests mantled with clasts that have pale-yellow weathering rinds and the till has distinct bright orange-yellow matrix (Fig. 4F). The clast from the less altered moraine is composed of primary volcanic glass groundmass (~60%) and primary volcanic minerals (clinopyroxene: 3%; anorthitic feldspars: ~20%) and a small percentage of clay (smectite ≤6%, Table 1). In contrast, the more rounded moraine, with an orange-yellow matrix is composed less of volcanic minerals (clinopyroxene not detected; anorthitic feldspars ≤11%) and more of clay including smectite (~40%), kaolinite (~5%), and alunite (~2%). The moraine consisting of altered volcanic material also had higher amounts of quartz and cristobalite; because poorly crystalline opal is indistinguishable from volcanic glass shards, the amorphous glass measured by XRD may consist of both primary and secondary silica. In the east Tana valley, field observations of opal clasts and an opalized dike, as well as a highly altered volcanic breccia, further demonstrated the degree of hydrothermal alteration in the eastern summit region of Tana volcano. Petrography of a pale breccia clast indicates that there are fragments of clasts with relict volcanic textures and growth of clays, quartz or cristobalite, and adularia in veins (Fig. 7A). Backscattered electron images by SEM reveal evidence of dissolution/replacement of Fe-Ti oxides that have intact subhedral mineral morphology and show the fine-grained texture and high aluminum content of the clay portion of the clast matrix, as well as crosscutting veins containing clay and amorphous silica (Fig. 7B). These replacement textures and secondary minerals suggest the presence of argillic, phyllic, and advanced argillic alteration (Burnham, Reference Burnham1962; Robb, Reference Robb2005). Argillic alteration occurs between 100°C and 240°C, producing smectite, illite, silica, pyrite, and calcite via hydrogen metasomatism (Robb, Reference Robb2005). Advanced argillic alteration occurs at 100–300°C and produces clays like kaolinite and smectite from feldspars (Robb, Reference Robb2005). The abundance of smectite suggests mainly argillic alteration, common near the surface of hydrothermal systems, and this would account also for the abundance of amorphous silica and cristobalite in the altered moraine clasts. Currently, active fumaroles and hot springs on the upper north and east flanks of Tana as well as mineralization near the summit caldera lake support an extensive Holocene to present hydrothermal system (Evans et al., Reference Evans, Bergfeld, Neal, McGimsey, Werner, Waythomas and Lewicki2015).
DISCUSSION
Glaciation of the Islands
No material suitable for radiocarbon dating was found associated with the glacial features in the IFM because trees have not been endemic throughout the Holocene; thus, we correlate the timing of glacial advance and retreat events with studies of other glaciated islands in the Aleutians. On Tana, moraines, U-shaped valleys, lavas with crude glacial polish and rare striations, and cirque headwalls are located on all aspects of the island with current glaciers limited to the east Tana valley. Moraines and glacial polish are exposed along the flanks of Carlisle Island indicating ice cover on the conical volcano. These extensive glacial features on both Carlisle and Tana volcanoes indicate significant ice capping the peaks of the IFM during the LGM. The degree of glaciation of Cleveland is impossible to ascertain because of its significant Holocene eruptive deposition. As with other Aleutian Islands, end moraines associated with the LGM advance in the IFM were likely deposited farther out on the continental shelf because of lower eustatic sea level.
Moraines with rounded crests in the east Tana valley are likely Neoglacial in age as they terminate ~2 km downvalley of the current glacier (Figs. 4 and 5). The moraines associated with this advance have rounded crests and large solifluction features (Figs. 4 and 6) compared with the moraines in close proximity to current ice. Correlating moraines with glacial advances in the Holocene is more difficult on Carlisle because the peak lacks current glaciers as a reference point. If, however, the Carlisle volcano was capped with ice at the LGM (as lodgment till observed at high elevations along the margin of this island suggests), then the lateral moraines at the head of the southeast fan, which hosts village AMK003, are likely Neoglacial in age (Fig. 3). The sharp-crested morphology of the moraines also suggests late Holocene deposition. Currently, the IFM experiences abundant winter precipitation and a mean annual temperature close to 0°C; thus even a slight decrease in temperatures or increase in winter precipitation could trigger a glacial advance.
In the east Tana valley, moraines near the current glacier terminus are consistent with LIA glaciation and suggest that the three distinct pairs of breached terminal moraines represent fluctuations in ice volume in the past ~1000 yr (Fig. 4). The proximal moraine complex is likely related to glacial advances between AD 1180 and 1320 (early LIA advance), AD 1540 and 1710 (middle LIA advance), or 1810 and 1880 (late LIA advance). Veniaminov (Reference Veniaminov1984) also recorded that the entire Bering Sea and all local straits were ice covered for a month in the winter of 1810, concurrent with the late LIA advance.
Other than the small glacier in the summit caldera of Herbert Volcano, the only current glacial activity in the IFM is found in east Tana valley. The presence of these glaciers in the IFM is unusual because of their elevation. The Tana Glacier is at 761 m in elevation, significantly lower elevations than other Aleutian Island glaciers (Thorson and Hamilton, Reference Thorson and Hamilton1986). The valley is oriented to the east–southeast, whereas most glaciers in the Aleutians usually are located on the north sides of volcanoes (Molnia, Reference Molnia2008). The presence of ice in the east Tana valley is likely the result of the large basin, which produces more ice than is typical on conical volcanic islands. Why should the largest basin be on Tana volcano’s east flank instead of its north flank? We propose the nature of the bedrock at the summit determined the preferential development of the east Tana valley and facilitated the creation and preservation of LIA moraines.
Hydrothermal alteration implications for landscape stability
The extant glaciation, large catchment area, and unusual valley morphology of the east Tana valley is unique in the IFM. We hypothesize that the greater extent of glacier erosion and the better development of LIA moraines is linked directly to the anisotropy of bedrock characteristics near the summit. Existing fumaroles and hot springs on Tana volcano are located high on the north flank near the region of summer snowfields and on the coastal cliffs of the east flank of the volcano. The rarity of eruptive deposits younger than the Neoglacial moraines, the presence of an active hydrothermal system (Evans et al., Reference Evans, Bergfeld, Neal, McGimsey, Werner, Waythomas and Lewicki2015), and the wide distribution of hydrothermally altered lava bedrock and clasts (current paraglacial load) all suggest hydrothermal alteration has featured in Tana’s eastern summit region for thousands of years. The hydrothermally produced clays are prone to shrink-swell physical weathering and overall decrease rock strength. The differential distribution of hydrothermal alteration has created a landscape that can be more easily eroded than portions of the island composed of unaltered rock. Moreover, the size and orientation of the east Tana valley suggest its origin is likely partially attributable to a sector collapse, not necessarily associated with an eruption, and perhaps exacerbated by the high degree of hydrothermal alteration that reduced rock strength. After sector collapse, glacial erosion extensively modified the east Tana valley. The weak hydrothermally altered rock is easily eroded, and glacial action created U-shaped valleys, rounded bedrock crests, cirques, and unusually well-preserved Neoglacial and LIA moraines.
In the east Tana valley, the effects of hydrothermal alteration on moraine morphology are pronounced. LIA moraines have very rounded crests indicating erosion through diffusionary processes of soil creep and solifluction (Fig. 6). Typical diffusion coefficients range between 10−2 and 10−4 m2/yr for typical alluvium in a wide range of climates (Hanks et al., Reference Hanks, Bucknam, Lajoie and Wallace1984; Putkonen and Swanson, Reference Putkonen and Swanson2003). Interestingly, the moraines in the east Tana valley demonstrate calculated diffusion coefficients on the low end of the typical range, potentially related to the coarse material of the moraines. Moreover, the moraine composed of heavily hydrothermally altered dacite has a diffusion coefficient that is an order of magnitude greater than the moraine composed of unaltered dacite. This high rate of diffusion is evident also downvalley on the highly rounded Neoglacial moraines (Fig. 6).
Geomorphic processes and human settlement
Middle and late Holocene village sites in the Aleutian Islands are located on relatively level surfaces that are commonly geomorphic surfaces such as sediment fans, debris aprons, wave-cut platforms, and ground moraines. Unlike settlements on Umnak Island and elsewhere in the Aleutians, IFM islands are small in area with little flat terrain at sea level. Prehistoric and historic settlements generally occur tens of meters above current sea level, potentially an advantage in an area of seismogenic tsunami events (Griswold et al., Reference Griswold, MacInnes and Higman2018). Late Holocene eustatic sea level fluctuations are significantly less than 10 m and thus may not have been a significant factor in settlement in the IFM (cf. Black, Reference Black1976). The village sites investigated in this study, however, are located on sediment fans or debris aprons that are directly adjacent to steep, unstable volcanic slopes, which may be a control on settlement particularly as the semi-subterranean house styles of the last 7000 yr (Davis and Knecht, Reference Davis and Knecht2010) require significant fine-grained sediment accumulation on lavas and sediment fans.
The Ulyagan village site (AMK003) on Carlisle is located on one of the largest sediment fans in the IFM (Fig. 3). The site is located adjacent to an ephemeral channel, yet there is a perennial channel ~2 km from the site. At Ulyagan, the ephemeral stream has incised and created a fill-cut terrace, which would have required significant stream power to incise and widen the floodplain at this location. The size of the incision, the presence of a fill-cut terrace, and the settlement site suggest that the ephemeral channel was once the perennial channel on the fan and had more consistent discharge. Deposition at the head of the fan has increased aggradation at the location of the ephemeral channel, and the primary channel has now incised farther to the southwest, away from the settlement. This channel avulsion may explain why Ulyagan is situated at a location that currently lacks a perennial water supply. Reworking of paraglacial fan sediments after the LIA may have produced the channel avulsion prior to the arrival of the Russians in 1759–1762 (Glotov’s voyage; Veniaminov, 1840) and the establishment of Russian era long-houses in Ulyagan village (West et al., Reference West, Crawford and Savinetsky2007; Hatfield et al., Reference Hatfield, Bruner, West, Savinetsky, Krylovich, Khasanov and Vasyukov2016). If so, other factors must have promoted the continued use of a village site even as the water supply became less certain. Although the timing of the channel avulsion is as yet unconstrained, the occupation of the village lasted until Russian depopulation of the IFM following the Aleut revolt (Hatfield et al., Reference Hatfield, Bruner, West, Savinetsky, Krylovich, Khasanov and Vasyukov2016).
The village site Tana$$\hat {\rm x}$$ Aguna
$$\hat{\rm x}$$ (SAM014) on Chuginadak Island is also situated on a sediment fan. The stratigraphy associated with Tana
$$\hat {\rm x}$$ Aguna
$$\hat {\rm x}$$ village is similar to that beneath Ulyagan village: thick debris flow and lahar deposits veneered by layers of fine-grained alluvium, tephra, and cultural material. For the fans on both islands, the lowermost fine-grained layers directly overlying thick debris flows have similar ages of ca. 3700 cal yr BP (Hatfield et al., Reference Hatfield, Bruner, West, Savinetsky, Krylovich, Khasanov and Vasyukov2016), which also dates the time of earliest occupation of each site. The concurrence of Neoglacial ages with the distinct changes in grain size and type of deposit suggests a switch in geomorphic processes from a system of debris flow deposition to one where overbank flooding and tephra deposition are more common.
A potential cause for the switch from predominantly coarse-grained debris flows to layered fine-grained sediments is a change in the sediment supply to the fans by a late Holocene decrease in eruptive frequency on Tana and Carlisle. Three lines of evidence, however, indicate relative Holocene quiescence of these volcanoes: (1) the peat bog stratigraphy that does not contain evidence of proximal deposits from Carlisle or Tana, (2) the substantial areas of summit erosion and flank vegetation on both volcanoes, and (3) the presence of only three lava flows with intact volcanic morphology on Carlisle, likely deposited after glaciation. The lack of evidence for Holocene eruptions at both locations and the presence of matrix-supported debris flow deposits with subangular clasts of different, weathered lithologies suggest a nonvolcanic origin for the debris flows. Contrastingly, the Cleveland volcano, frequently erupting throughout the Holocene (Neal et al., Reference Neal, Izbekov and Nicolaysen2015), has many postglacial lava flows, and its debris fans consist primarily of clast-supported debris and pyroclastic flow deposits with coarser matrices, more angular clasts, and homogeneous lava lithology (Waythomas, Reference Waythomas2015) suggesting a volcanic origin for the bulk of the sediment in the debris aprons (Fig. 9). Thus, a nonvolcanic process (i.e., climatically driven changes to glacial and paraglacial conditions) may be responsible for the change in sediment fan stratigraphy.
The consistency in age and type of fan sedimentation on Tana and Carlisle may signal a switch in sedimentation on the fans related to the waning effects of paraglaciation after the LGM. Increased glacial ice causes erosion high in drainage basins, but increased sedimentation rates on fans occurs during deglaciation (Ballantyne, Reference Ballantyne1992). In other glaciated regions, broad fan-shaped deposits are built almost entirely of debris flow deposits with little or no fluvial sediments during paraglacial periods (Ryder, Reference Ryder1971; Jijun et al., Reference Jijun, Derbyshire and Shuying1984; Kostaschuck et al., Reference Kostaschuck, MacDonald and Putnam1986, Reference Kostaschuck, MacDonald and Jackson1987; McArthur, Reference McArthur1987; Eyles and Kocsis, Reference Eyles and Kocsis1988). The bulk of fan sediments described on Carlisle and Tana are similar with no evidence of fluvial processes and layering. Thus, the switch is caused by a transition from paraglacial debris flow sedimentation to fan aggradation by overbank and tephra sedimentation, as steep slopes on the flanks of volcanoes shed most of the unstable rock produced during LGM glaciation. Moraines documented on both Carlisle and Tana indicate that the IFM experienced a glacial advance during the Neoglacial period; however, the volume and thickness of ice as documented by moraines in the IFM suggest that this event was relatively minor compared with the LGM. Limited ice thickness and the short period of glaciation suggest that slopes were not loaded with nearly as much unstable rock sediment as they were at the LGM, and thus the fan surfaces were more stable with fewer debris flow events in the late Holocene.
The paraglacial fan environment after the LGM may have resulted initially in landscapes unsuitable for sustained human settlement. Because the islands are small, human settlements on sediment fans and debris aprons directly adjacent to steep volcanic slopes would have been particularly susceptible to hyperconcentrated flows, debris flows, and landslides. These unstable surfaces would be rocky with little vegetation and constantly shifting channels and unpredictable debris flow sedimentation. Additionally, the surface deposits would be dominantly coarse grained and not amenable for digging of pit houses. A modern analog for this environment is the highly unstable, minimally vegetated east Tana valley where glaciers have receded substantially since the LIA (Fig. 3). There is no evidence for human occupation in the east Tana valley, there are unvegetated highly unstable slopes in the upper third of the valley, and the mouth of the valley contains debris flow and outwash deposits (Fig. 4).
Regardless of the exact timing of LGM glacial retreat in the IFM, the paraglacial adjustment period likely extended into the Holocene. Adjustment was likely to have persisted into the mid-Holocene as the effects of paraglaciation on fans persist for thousands of years (Beaudoin and King, Reference Beaudoin and King1994; Friele and Clague, Reference Friele and Clague2009). Thus, the earliest human migrants to the IFM may have had to contend with paraglacial environments. The small islands of the IFM would have provided minimal stable flat surfaces disconnected from slope systems and thus were not settled during the first migration of people across the Aleutians ~9000 ka. Even if sea level was decreased in the early Holocene, there were likely no stable flat surfaces isolated from steep volcanic slopes (Fig. 11). Instead, migration and settlement occurred farther west of the IFM, at Adak Island, which was settled ~7 ka (Savinetsky et al., Reference Savinetsky, West, Antipushina, Khassanov, Kiseleva, Krylovich and Pereladov2012). Adak Island is larger than the islands in the IFM, and the settlement site is located on a broad flat geomorphic surface that is not directly connected to steep volcanic slopes. Perennial settlements in the IFM potentially occurred later and correspond to the switch in sedimentation type that provided fine-grained material suitable for construction of pit houses (Fig. 12). Interestingly, sedimentologic data from St. Lawrence Island also suggest that the mid- to late Holocene is associated with more stable land surfaces (Lozhkin et al., Reference Lozhkin, Anderson, Eisner and Solomatkina2011).

Figure 11 (color online) Comparison of the island morphology of Chuginadak and Carlisle Islands (A) with the southern tip of Umnak Island (B) where early Holocene Unangax settlements are preserved. The Islands of Four Mountains (IFM) digital elevation model was produced by combining SRTM (Shuttle Radar Topography Mission) 1-degree elevation data and smooth sheet bathymetry. Shorelines in the early Holocene were estimated by subtracting the glacial isostatic uplift rate (GIA; Peltier, Reference Peltier2004; A et al., 2013) from eustatic sea level (Lambeck et al., Reference Lambeck, Rouby, Purcell, Sun and Sambridge2014). Any tectonic effects are not accounted for. In this model, there is more exposed coastline along Umnak Island because of the slower rate of isostatic rebound. This estimate is similar to that of Black (Reference Black1976) who estimated that early Holocene sea level was 2–3 m above modern sea level. In the IFM, more of the flanks of the volcanoes are exposed; however, these surfaces are still directly connected to the steep slopes of the volcanoes compared with the sites on Umnak that are flat and not directly connected to the steep slopes of Mounts Vsevidof and Recheshnoi.

Figure 12 Timeline of climate, settlement, and geologic events in the Islands of Four Mountains (IFM) and elsewhere in the Aleutians. Dashed lines indicate inferred ages based on other chronologies in the Aleutians. LIA, Little Ice Age.
Alternatively, the islands could have been settled prior to 3.7 ka, and that evidence has been buried or eroded. Paraglacial and volcanic debris flow deposits are tens of meters thick, and thus mass wasting from the head of the Tana and Carlisle sediment fans may obscure the earliest evidence of human occupation. Future work should investigate contacts between coarse-grained thick deposits in more detail to further test this hypothesis. Older sites may have also been situated on the distal portion of debris aprons and sediment fans as the fan and apron surfaces grade to a base level offshore from the modern coastline. A maximum amount of erosion since the first geochemical evidence of humans can be estimated by using a best fit line of the fan surface (at the Ulyagan village site, AMK003) and extending it to base level (Fig. 3). Because of the relatively small size of the fan, elevation changes on the fan attributable to isostatic adjustment would be minimal, and thus eustatic sea level would be the dominant control on the base level of the fan, which was −4 m at ~7ka (Lambeck et al., Reference Lambeck, Rouby, Purcell, Sun and Sambridge2014). The location of the shoreline is predicted from the intersection of the fan surface with the vertically adjusted sea level. Two estimates of erosion were produced used using different reconstructed fan surfaces. The first surface is reconstructed using the shape of the entire fan indicating a shoreline retreat of ~500 m horizontally because of coastal erosion. The second surface is reconstructed using only the planar surface of the upper and middle portions of the fan, as these may better represent the mid-Holocene surface. The complication this avoids is that the more distal portions of the fan have thicker deposits of younger layered tephras, alluvium, and culture deposits, which significantly reduce the gradient of the distal fan surface. Using the projection of the upper-middle fan surface produces an estimated 300 m of shoreline retreat because of coastal erosion. The 300–500 m of coastal erosion may have filtered the archeological record, removing any evidence of early or middle Holocene sites. Thus, the extant archaeological record may be biased toward younger occupations as a result of taphonomic processes rather than demographic dynamics.
CONCLUSIONS
Glacial and paraglacial processes are significant modifiers of the volcanic landscapes of the IFM, and deposits of these processes point to a physical environment in transition at the time of human occupation. Hydrothermal alteration of bedrock exerts a significant control on island morphology and has promoted the development of a significant glaciated basin, containing a rapidly retreating glacier, on the east side of Tana volcano. At the LGM, glaciers covered most if not all of the IFM, and deglaciation likely occurred in the early Holocene or latest Pleistocene, similar to other Aleutian Islands. Glacier advance during the Neoglacial occurred on both Carlisle and Tana volcanoes. In the east Tana valley, the presence of hydrothermally altered bedrock facilitated the development of distinct LIA and Neoglacial moraines and influenced the moraine morphology through high rates of diffusion that created more rounded crests and large solifluction lobes. If human settlement predating 3.7 ka did occur in the IFM, as suggested indirectly by peat bog nitrogen isotopic compositions (Kuzmicheva et al., in Reference Kuzmicheva, Smyshlyaeva, Vasyukov, Khasanov, Krylovich, Okuno, West, Hatfield and Savinetskypress), the earliest IFM sites may be deeply buried in sediment fan deposits or filtered from the extant record through coastal erosion associated with eustatic sea level rise. Alternatively, the initial westward settlement of the Aleutian Islands may have bypassed the IFM 7–9 ka because small size of the islands and their unstable fan surfaces offered little terrain suitable for long-term settlement. In this case, seasonal exploitation of avian food resources could explain nitrogen signals of human occupation and delayed village establishment until Neoglacial times, ca. 3.7 ka. Regardless, the concurrence of well-dated human cultural features with the presence of inferred LIA moraines supports an inference of human resilience to climate change, even on small subarctic islands.
ACKNOWLEDGMENTS
The professional efforts of the crew of the R/V Maritime Maid (Captain George Rains, engineer Joe Schmidt, able seaman, cook Mike Despars, and able seaman Wesley Jones, as well as Maritime Helicopters pilot Dan Leary and helicopter mechanic Mike Cooper) were integral to our safe and successful field seasons, for which we thank them. The scientific team and logistics were funded by the National Science Foundation (NSF) Office of Polar Programs (OPP#1301927, OPP#1301925, and OPP#1301929) and by the Keck Geology Consortium (REU#1358987) for the participation of undergraduate students HL and LL, and further support was from the U.S. Geological Survey, the Alaska Volcano Observatory, the U.S. Fish and Wildlife Service, the Alaska Maritime National Refuge, the Aleut Corporation, and the Museum of the Aleutians. Digital elevation models provided by the Polar Geospatial Center under NSF OPP awards #1043681, #1559691, and #1542736. We greatly appreciate the associated work of Christie Haupert (CH2M Polar Services) and USGS geologists Christina Neal, William E. Scott, John Lyons, John Power (then scientist-in-charge), Max Kaufman, Kristi Wallace, Michelle Coombs (current scientist-in-charge), and Matthew Heaney. We thank Breanyn MacInnes for assistance with and access to the Mastersizer particle size analyzer at Central Washington University. Finally, this paper benefited from an informal review by Robert J. Carson and two peer reviews. Any opinions, findings, and conclusions or recommendations expressed in this material are those of the author(s) and do not necessarily reflect the views of the NSF.