1. Introduction
The periodic merger and break-up of supercontinents has now been recognized as the key factor that profoundly affects the Earth system, including climate, mineralization and the evolution of life (e.g. Stern, Reference Stern2008; Gaucher et al. Reference Gaucher, Sial, Halverson, Frimmel, Gaucher, Sial, Halverson and Frimmel2009). The South China block, one of the oldest (up to Palaeoarchaean) and biggest (up to 2500 km across) continental blocks in China, comprises the Yangtze block and the Cathaysia block. It has been widely attractive to geologists because of its widespread and intensive Neoproterozoic magmatism, which has been correlated with the evolution of the Rodinia supercontinent (Zhou et al. Reference Zhou, Yan, Kennedy, Li and Ding2002; Li et al. Reference Li, Li, Ge, Zhou, Li, Liu and Wingate2003, Reference Li, Bogdanova, Collins, Davidson, Waele, Ernst, Fitzsimons, Fuck, Gladkochub and Jacobs2008; Zheng et al. Reference Zheng, Zhang, Zhao, Wu, Li, Li and Wu2007; Qiu et al. Reference Qiu, Ling, Liu, Kusky, Berkana, Zhang, Gao, Lu, Kuang and Liu2011). However, issues such as the petrogenesis of these Neoproterozoic magmatic rocks and the suggested palaeoposition of the South China block in the Rodinia configuration are highly debated (Zhou et al. Reference Zhou, Yan, Kennedy, Li and Ding2002; Li et al. Reference Li, Bogdanova, Collins, Davidson, Waele, Ernst, Fitzsimons, Fuck, Gladkochub and Jacobs2008; Qiu et al. Reference Qiu, Ling, Liu, Kusky, Berkana, Zhang, Gao, Lu, Kuang and Liu2011, Reference Qiu, Jiang, Lu and Khattak2021; Wen et al. Reference Wen, Evans and Li2016; Song et al. Reference Song, Liu, Meng, Yuan, Feng, Zhou, Romani and Yan2019; Cawood et al. Reference Cawood, Wang, Zhao, Xu, Mulder, Pisarevsky, Zhang, Gan, He, Liu, Qi, Wang, Yao, Zhao, Zhou and Zi2020; Jing et al. Reference Jing, Evans, Yang, Tong, Xu and Wang2021; Wang et al. Reference Wang, Xu, Santosh and Zeng2021; Zhu et al. Reference Zhu, Liu, Zhang, Chen, Xiao, Zhao and Yan2021). Some researchers proposed that the South China block was located between Australia and Laurentia at the centre of the Rodinia supercontinent, and that the Neoproterozoic magmatic rocks in the Yangtze block correspond to the activity of the mantle plume that accompanied the break-up of the Rodinia supercontinent (Li et al. Reference Li, Li, Ge, Zhou, Li, Liu and Wingate2003, Reference Li, Bogdanova, Collins, Davidson, Waele, Ernst, Fitzsimons, Fuck, Gladkochub and Jacobs2008). Alternative models argue against the plume model, and hold the view that the Neoproterozoic magmatic rocks along the western and southeastern margin of the Yangtze block have a subduction-related geochemistry and constitute part of the so called ‘Hannan-Panxi arc’ and ‘Jiangnan arc’ system, respectively, in which the South China block occupied a peripheral position adjacent to India within the Rodinia supercontinent (e.g. Zhou et al. Reference Zhou, Yan, Kennedy, Li and Ding2002; Zhao et al. Reference Zhao, Zhou, Yan and Zheng2011). These controversies are partly due to competing interpretations of the geochemical features of the Neoproterozoic magmatic rocks, and partly due to a lack of understanding of the geodynamic mechanism of the Proterozoic orogenesis.
Recent geological surveys and geochronological studies reveal that the Yangtze block could be made up of distinct tectonic domains or microcontinents that merged during the Neoproterozoic period, based on observations of a continental arc series and ophiolites in the northern part of the Yangtze block (e.g. Qiu et al. Reference Qiu, Ling, Liu, Kusky, Berkana, Zhang, Gao, Lu, Kuang and Liu2011; Peng, S. B. et al. Reference Peng, Kusky, Jiang, Wang, Wang and Deng2012; Hu et al. Reference Hu, Mao, Tian and Li2015; Xu et al. Reference Xu, Yang, Polat and Yang2016; Tang et al. Reference Tang, Wang, Zhou, Wu, Chen, Hu, Zhao, Dong, Yu and Hu2020). However, the evolutionary history of the orogenic processes in the Yangtze block is poorly constrained. Neoproterozoic magmatic rocks are widely distributed in the South Qinling block (SQB) and along the northern margin of the Yangtze block (Zhao & Asimow, Reference Zhao and Asimow2018; Qiu et al. Reference Qiu, Jiang, Lu and Khattak2021). Given that the SQB is thought to be the segment involved in the Qinling–Dabie orogen, systematic investigations of the Neoproterozoic magmatic rocks will thus help us to understand the temporal evolution of the igneous activities and geodynamic processes during the orogeny. Granites can record significant information regarding the nature of orogenesis. A-type granites are a distinctive group of granitoids characterized by high alkali contents and high Fe/Mg ratios, with ages of emplacement apparently unrelated to major orogenic events (Barbarin, Reference Barbarin1999). They commonly formed in an extensional crustal setting, and can be used to address the tectonic processes of specific geological events (Whalen et al. Reference Whalen, Currie and Chappell1987; Eby, Reference Eby1992; Siegel et al. Reference Siegel, Vasyukova and Williams-Jones2018). In addition, post-orogenic A-type granites on ancient cratons may signal the end of an orogeny, and thus can be employed to constrain the time limits of the orogenic processes (Li et al. Reference Li, Lin, Xing, Jiang and Xia2018).
Here we present an integrated geochemical study, including zircon U–Pb ages, zircon Hf isotopic compositions, and whole-rock elemental and Nd isotopic data for the Chengwan granitic pluton from the northern margin of the Suizao terrane in the SQB. The aims of our study are to (1) precisely date the granites and depict their geochemical features; (2) understand their petrogenesis and tectonic setting; and (3) shed new light on the Neoproterozoic tectonic evolution of the SQB.
2. Geological background
The Qinling–Dabie orogen is suggested to have finally formed during early Mesozoic time due to the closure of the easternmost Palaeo-Tethys (Hsü et al. Reference Hsü, Li, Chen, Wang, Sun and Şengör1990). The Palaeozoic Shangdan and the Mesozoic Mianlue sutures separate the SQB from the North Qinling orogenic belt to the north and the Yangtze block to the south, respectively (Fig. 1a) (Meng & Zhang, Reference Meng and Zhang2000).
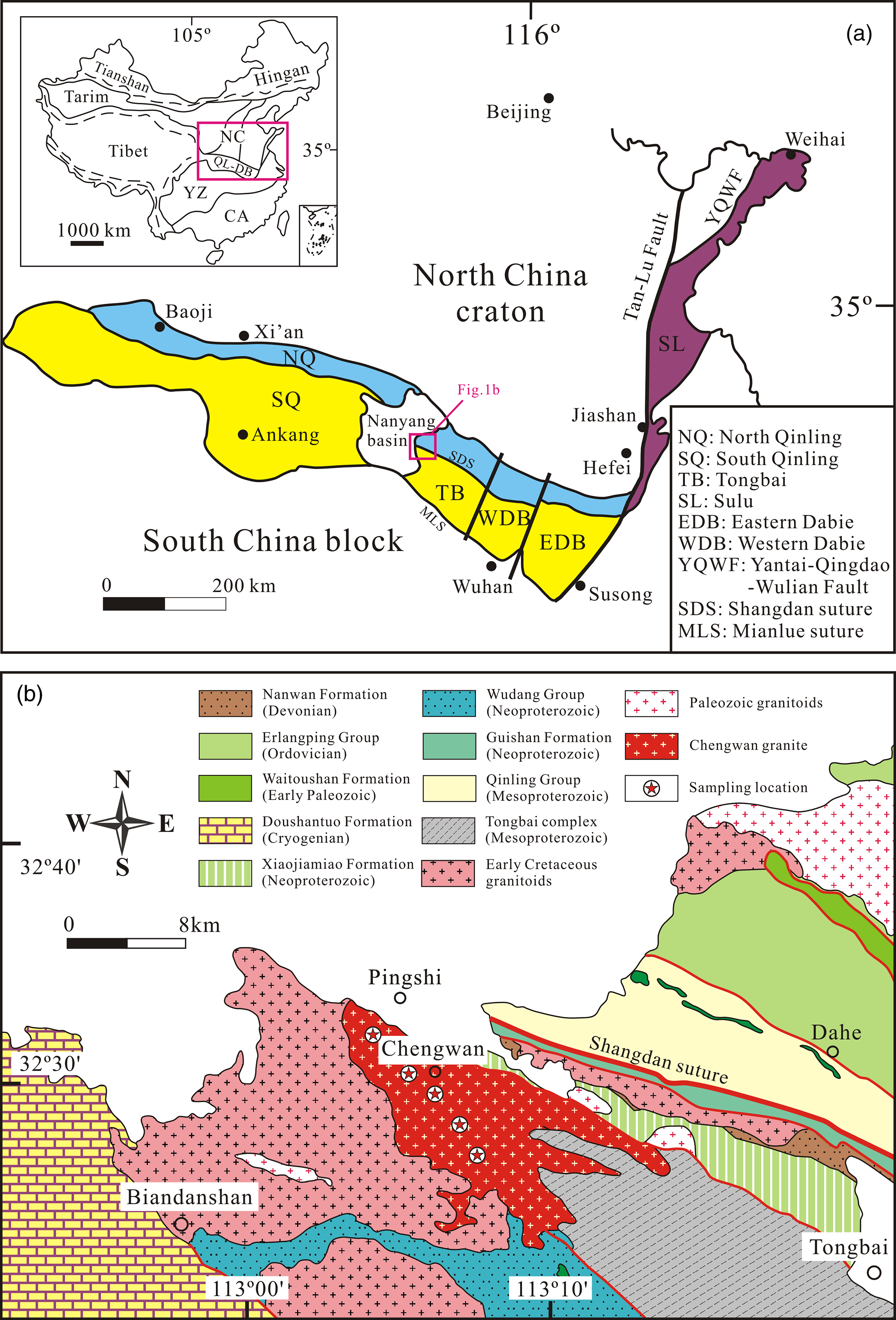
Fig. 1. (a) Schematic geological map for the Qinling–Dabie–Sulu orogenic belt (QL-DB). Insert shows major tectonic divisions of China (modified after Zheng et al. Reference Zheng, Fu, Gong and Li2003). (b) Sketch geological map of the northern Suizao terrane, showing the sampling locations (modified after Li, Reference Li2018). NC – North China Craton; YZ – Yangtze block; CA – Cathaysia block.
The basement of the SQB, represented by the Yudongzi Group and the Douling complex, is a suite of Neoarchaean medium- to high-grade metamorphic rocks consisting of mostly tonalitic–trondhjemitic–granodioritic (TTG) gneisses with a few intercalated amphibolites and metasedimentary rocks (Hu et al. Reference Hu, Liu, Chen, Qu, Li and Geng2013, Reference Hu, Liu, Qu and Chen2019; Wu et al. Reference Wu, Zhou, Gao, Liu, Qin, Wang, Yang and Yang2014; Zhou et al. Reference Zhou, Wu, Li, Zhang, Zheng, Wang and Yang2018). The Suizao terrane is located in the eastern part of the SQB. The oldest rocks documented so far in the Suizao terrane consist mainly of Neoproterozoic metamorphic rocks (the metamorphic enclaves in the Tongbai complex) and volcanic-sedimentary rocks (the Wudang Group) (Liu, X. C. et al. Reference Liu, Jahn, Cui, Li, Wu and Li2010; Cui et al. Reference Cui, Liu, Dong and Hu2012). The Tongbai complex constitutes the core of the Tongbaishan anticline. The majority of the Tongbai complex is strongly deformed granitoids, which make up ˜80 % of the complex. The metamorphic enclaves consist of fine-grained TTG gneisses, amphibolites, calc-silicates and marbles (Liu, X. C. et al. Reference Liu, Jahn, Cui, Li, Wu and Li2010; Cui et al. Reference Cui, Liu, Dong and Hu2012). The Wudang Group has experienced low- to high-greenschist-facies metamorphism. From the bottom upwards it can be divided into two parts. The lower part mainly comprises clastic sedimentary rocks. The upper part is a typical intermediate-silicic volcanic-sedimentary rock formation (Li & Wang, Reference Li and Wang2000). Based on zircon U–Pb dating, the clastic to volcanic sequences of the Wudang Group in the Suizao terrane were formed at ˜770–710 Ma (Xue et al. Reference Xue, Ma and Song2011; Yang, Y. N. et al. Reference Yang, Wang, Li and Li2016; Liu et al. Reference Liu, Zhao, Cawood and Wang2018). Early Neoproterozoic granitoids and diabasic–gabbroic dykes or sills occurred mainly in the southern part of the Suizao area (e.g. Shi et al. Reference Shi, Liu, Zhang, Miao, Zhang and Xue2007; Xu et al. Reference Xu, Yang, Polat and Yang2016; Qiu et al. Reference Qiu, Jiang, Lu and Khattak2021). For example, SHRIMP zircon U–Pb dating on gabbro and granite from the southern part of the Suizao terrane indicates crystallization ages of 947 ± 14 Ma and 876 ± 17 Ma, respectively. Recently, Xu et al. (Reference Xu, Yang, Polat and Yang2016) reported SHRIMP zircon U–Pb ages of 862–858 Ma from three granodiorites and 871 ± 7 Ma from one gabbroic dyke from the Sanligang region in the Suizao terrane. Their geochemical features further imply that they might have formed in an arc-related setting. In addition, late Neoproterozoic mafic–ultramafic intrusions are widely distributed in the Suizao terrane. Recent geochronological studies reveal that they might have formed at ˜650–600 Ma (e.g. Zhao & Asimow, Reference Zhao and Asimow2018; Li et al. Reference Li, Liu, Liao, Gai, Ma and Wang2020; Qiu et al. Reference Qiu, Jiang, Lu and Khattak2021). In contrast, middle Neoproterozoic intrusive rocks are rarely reported in the Suizao terrane, represented by the Chengwan granite investigated in this study (Li, Reference Li2018).
3. The Chengwan granite
The Chengwan granitic pluton lies to the south of the Shangdan suture. It is one of the largest granitic plutons in the Suizao terrane of the SQB, with an outcrop area of over 80 km2 (Fig. 1b). The granite shows tectonic contacts with the surrounding Tongbai complex and the Wudang Group, and is intruded by Palaeozoic syenites (Fig. 2a).

Fig. 2. Photographs of the Chengwan granite. (a) The granite is intruded by Palaeozoic syenite. (b) Pegmatites in the Chengwan granitic pluton. Hammer for scale is 27.5 cm long. (c) Field outcrop of the Chengwan granite. Coin for scale is 2 cm in diameter. (d) Photomicrographs of the Chengwan granite (Kf – potash feldspar; Pl – plagioclase; Q – quartz; Bi – biotite).
The Chengwan pluton is dominated by medium- to coarse-grained monzogranites and minor syenogranites, with a couple of pegmatites occurring as dykes in the pluton (Fig. 2b). No sharp contact can be observed between these granitic rocks (Fig. 2a). Mylonization can be observed at the margin of the pluton, especially near the boundary between the pluton and the Tongbai complex. No mafic enclaves can be found in the Chengwan granite. The granites are light pink in colour, generally exhibiting a granitic texture, and are massive to well foliated with gneissic structures (Fig. 2c). The granitic pluton is well foliated at its northern margins, close to the Tongbai complex, revealing that the fabrics are post-emplacement. The syenogranites and monzogranites consist mainly of potash feldspar (˜50–65 %), plagioclase (10–25 %), quartz (˜20–25 %) and biotite (<5 %), with accessory minerals of zircon, apatite and magnetite. Sphene can be observed locally in some samples. A low degree of alteration is indicated by minor amounts of kaolinite and sericite observed in the granites. Potash feldspars are subhedral to anhedral in shape, with grain sizes ranging from ˜0.3 to 2.0 mm. Most of the potash feldspars are microcline with grid twinning and perthite with linear exsolutions. Quartz grains are anhedral in shape and vary in size from ˜0.2 to 0.5 mm. Plagioclase laths are subhedral and vary from ˜0.2 to 2.0 mm in diameter. Biotites are anhedral to subhedral and vary from ˜0.2 to 1.5 mm in diameter (Fig. 2d).
This large granitic pluton has long been regarded as having been emplaced during Palaeozoic time by previous 1:200 000 and 1:250 000 map scale geological surveys. However, a recent 1:50 000 map scale geological survey and zircon U–Pb dating suggest a middle Neoproterozoic age (Li, Reference Li2018), making it contemporaneous with the widespread Neoproterozoic magmatism in the South China block.
Twenty samples were collected along the road-cuts from five localities in the Chengwan pluton. None of them were collected in the deformed zones. All the samples are fresh and representative based on field and microscopic observations. Two representative samples were further processed for zircon U–Pb dating and Hf isotopic analyses.
4. Analytical methods
Zircon grains from samples 17LZY02 and 16LZY03 were separated by standard heavy liquid and magnetic separation techniques. Representative grains were selected under a binocular microscope and mounted in epoxy resin, and then polished and coated with carbon. Their internal structure was examined using cathodoluminescence (CL) imaging before in situ U–Pb dating and Hf isotopic analysis. The CL imaging was conducted at Beijing Gaonian GeoAnalysis Co. Ltd., using a JSM6510 environmental scanning electron microscope equipped with a Gatan detector.
U–Pb dating of the zircons was conducted by laser ablation inductively coupled plasma mass spectrometry (LA-ICP-MS) at the Laboratory of Isotope Geochemistry, Wuhan Centre of China Geological Survey. The RESOlution laser ablation system equipped with a 193-nm ArF-excimer laser was used coupled with an iCAP Q ICP-MS. Helium was used as the carrier gas to transport the ablated materials from the laser ablation cell into the ICP-MS. The laser spot diameter was 29 μm, while errors on pooled analyses are at the 2σ level. Zircon 91500 was used as a U–Pb isotope standard, and standard glass NIST610 was used to normalize U, Th and Pb contents. The detailed analytical procedures of Qiu et al. (Reference Qiu, Jiang, Wu, Zhao and Xu2020) were followed. Zircon trace-element analyses were simultaneously obtained during zircon U–Pb dating. NIST610 was used as an external standard to calculate the trace-element contents. 29Si was used as an internal standard to normalize each analysis. 49Ti was analysed for calculation of concentrations to avoid the isobaric interference of double-charged 96Zr with 48Ti. The average analytical error is ˜10 % for the trace elements. Raw data were processed using the ICPMSDataCal software (Liu, Y. S. et al. Reference Liu, Gao, Hu, Gao, Zong and Wang2010). The geochronological data were processed using the ISOPLOT program (Ludwig, Reference Ludwig2003).
Zircon Lu–Hf isotopic analyses were carried out on a Neptune Plus multi-collector ICP-MS equipped with a RESOlution laser ablation system at the Laboratory of Isotope Geochemistry, Wuhan Centre of China Geological Survey. Helium was used as the carrier gas and mixed with argon make-up gas via a T-connector before entering the ICP-MS. Lu–Hf isotopic analyses were obtained on the same zircon grains that were previously analysed for U–Pb isotopes, with ablation spots of 43 µm in diameter, an ablation time of 60 seconds, repetition rate of 6˜8 Hz and laser beam energy density of 5 J cm−2. The 179Hf/177Hf and 173Yb/171Yb ratios were used to calculate the mass bias of Hf (βHf) and Yb (βYb), which were normalized to 179Hf/177Hf = 0.7325 and 173Yb/171Yb = 1.12346 (Thirlwall & Anczkiewicz, Reference Thirlwall and Anczkiewicz2004) using an exponential correction for mass bias. Interference of 176Yb on 176Hf was corrected by measuring the interference-free 173Yb isotope and using 176Yb/173Yb = 0.78696 (Thirlwall & Anczkiewicz, Reference Thirlwall and Anczkiewicz2004) to calculate 176Yb/177Hf. Off-line selection and integration of analyte signals and mass bias calibrations were performed using the ICPMSDataCal software (Liu et al. Reference Liu, Zong, Kelemen and Gao2008). A decay constant value of 1.867 × 10−11 a−1 for 176Lu (Söderlund et al. Reference Söderlund, Patchett, Vervoort and Isachsen2004) and the present-day chondritic ratios of 176Hf/177Hf = 0.282772 and 176Lu/177Hf = 0.0332 (Blichert-Toft & Albarède, Reference Blichert-Toft and Albarède1997) were adopted to calculate the εHf(t) values. The depleted mantle model ages (TDM) were calculated using the measured Lu/Hf ratios and the present-day 176Hf/177Hf and 176Lu/177Hf ratios of 0.283250 and 0.0384, respectively, for the depleted mantle (Griffin et al. Reference Griffin, Pearson, Belousova, Jackson, Van Achterbergh, O’Reilly and Shee2000).
Whole-rock geochemical and Sm–Nd isotopic analyses were performed at the Zhongnan Mineral Resources Supervision and Test Centre for Geoanalysis, Wuhan Centre of China Geological Survey. Major elements were obtained on fused glass beads using a PANalytical AxiosmAX X-ray fluorescence spectrometer. Trace elements, including the rare earth elements (REEs), were determined using a Thermo Fisher X Series 2 ICP-MS. USGS standards AGV-1, GSR-3 and BCR-2 were used as reference materials. Based on the USGS standards and duplicate analyses, the analytical precision and accuracy of the XRF methods are estimated to be better than 5 % (RSD) for the oxides. The ICP-MS analyses yielded an accuracy and precision better than 5 % for most trace elements and about 10 % for some transition elements. Whole-rock Sm–Nd isotopic analyses were performed using a Triton Ti thermal ionization mass spectrometer (TIMS). The detailed analytical method has been described elsewhere (Qiu et al. Reference Qiu, Yang, Zhao, Lu, Wu, Zhang and Zhang2016). Mass fractional calibration for the 143Nd/144Nd ratios were based on the value of 146Nd/144Nd = 0.7219. The 143Nd/144Nd ratio of the ZkbzNd (JMC) standard measured during the run has an average of 0.511552 ± 0.000004 (2σ, n = 6). Analyses of the USGS standard BCR-2 gave 143Nd/144Nd = 0.512633 ± 0.000003 (2σ, n = 10), which agrees well with the recommended value within analytical errors (0.512630 ± 0.000007) (Raczek et al. Reference Raczek, Jochum and Hofmann2003).
5. Results
5.a. Zircon U–Pb ages
Sample 17LZY02 is a monzogranite from the Chengwan pluton near Liziyuan village (32° 24′ 56″ N; 113° 07′ 57″ E). Most zircons from this sample are euhedral to subhedral in form and ˜50 to 120 μm in length with length-to-width ratios of ˜1:1 to 2:1. Most zircons are transparent and show oscillatory zoning in CL images (Fig. 3). No inherited cores can be observed. Seventeen analyses of 17 zircons were determined (Table 1). The zircons U contents vary from 91.4 to 754 ppm, and Th contents from 129 to 1753 ppm. Their Th/U ratios are 0.85 to 3.35. All analyses have concordant (concordance ≥95 %) U–Pb ages within analytical errors, yielding a weighted mean 206Pb–238U age of 808 ± 9 Ma (MSWD = 0.50, n = 17) (Fig. 4a). This age is interpreted as the crystallization age of the Chengwan granite.
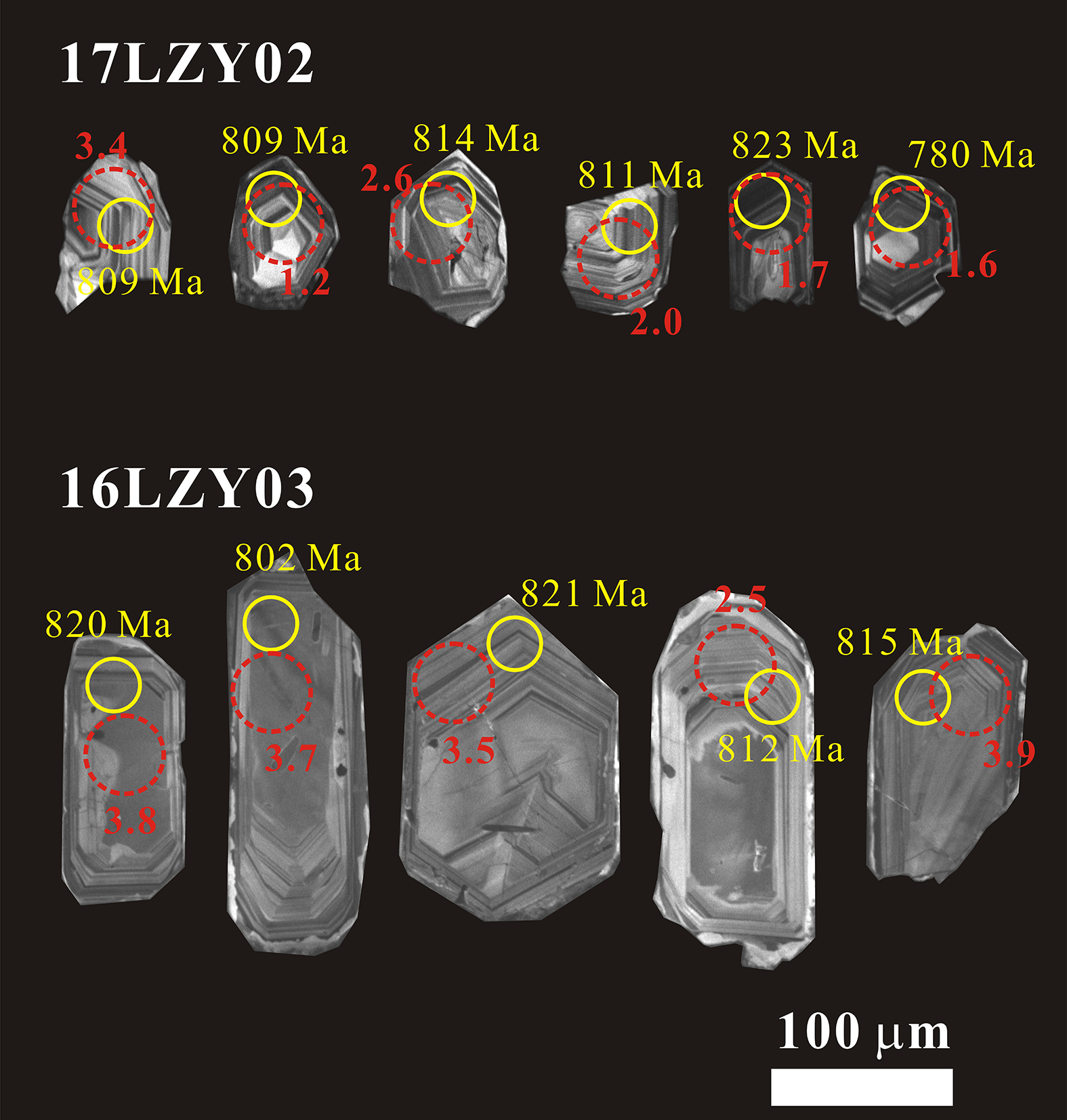
Fig. 3. Cathodoluminescence (CL) images of representative zircons for the Chengwan granite showing grain size. The smaller circles show LA-ICP-MS U–Pb isotope analytical spots with corresponding apparent 206Pb–238U ages, and the larger ones show locations of Lu–Hf isotope analyses with corresponding εHf(t) values. Scale bar is 100 µm.
Table 1. U–Pb isotopic ratios and apparent ages of zircons from the Chengwan granite of the South Qinling block

Conc. (%) is percentage concordance defined as ((206Pb–238U age)/(207Pb–206Pb age)) × 100.
Decay constants: 235U = 9.8485 × 10−10; 238U = 1.55125 × 10−10; 238U/235U = 137.88.
T was calculated by using the Ti-in-zircon thermometer of Ferry & Watson (Reference Ferry and Watson2007).

Fig. 4. U–Pb concordia diagrams for zircons from the Chengwan granite: (a) 17LZY02 and (b) 16LZY03. The error ellipses illustrated are 2σ.
Sample 16LZY03 is a syenogranite from the Chengwan pluton near Xiaowan village (32° 28′ 33″ N; 113° 06′ 59″ E). The zircon grains are subhedral in form and ˜80–200 μm in length. They have similar features to those from sample 17LZY02 in CL images (Fig. 3). Twenty-six spots on 26 grains were analysed, and most of them are concordant (Table 1). They have Th/U ratios of 0.41–0.67. Except for five discordant analysis, the remaining 21 analyses from the sample comprise a tight population giving a weighted mean 206Pb–238U age of 816 ± 4 Ma (MSWD = 0.61, n = 21) (Fig. 4b), nearly identical to the U–Pb age for sample 17LZY02.
5.b. Zircon Hf isotopes
A total of 25 Lu–Hf analyses, 9 for the sample 17LZY02 and 16 for the sample 16LZY03, were made on 25 zircon grains from the Chengwan granite. The results are listed in Table 2. εHf(t) values were calculated using the zircon age for the sample presented above (t = 810 Ma). Zircons from sample 17LZY02 have relatively narrow 176Hf/177Hf ratios of 0.282324 to 0.282380 and 176Lu/177Hf ratios of 0.001001 to 0.001951. εHf(t) values for these zircons are all positive, ranging from 1.2 to 3.4, corresponding to depleted mantle Hf isotopic model ages (TDM-Hf) of from 1327 to 1236 Ma with a weighted average of 1289 ± 25 Ma (Table 2). Zircons from sample 16LZY03 have 176Hf/177Hf ratios ranging from 0.282372 to 0.282421 and 176Lu/177Hf ratios from 0.000947 to 0.002647. The εHf(t) values are positive, ranging from 2.5 to 4.4, corresponding to a TDM-Hf range of from 1291 to 1201 Ma with a weighted mean of age 1233 ± 15 Ma (Table 2).
Table 2. Lu–Hf isotopic compositions of zircons for the Chengwan granite from the South Qinling block

εHf(t) values are calculated using their formation age (810 Ma).
5.c. Whole-rock major and trace elements
Elemental analyses for the Chengwan granite are listed in Table 3. The Chengwan granite has high SiO2 (72.09–77.42 wt %), alkali contents with Na2O + K2O of 6.89 to 8.49 wt %, and relatively low MgO (0.07–0.67 wt %), CaO (0.09–1.69 wt %) and P2O5 contents (0.01–0.09 wt %). They fall mainly in the field of monzogranite on the QAP plot (Fig. 5a). Most samples are peraluminous with A/CNK (molar Al2O3/(CaO + Na2O + K2O)) values ranging from 1.02 to 1.51, except for one sample (16LZY05, A/CNK = 0.91) that plots in the metaluminous field (Fig. 5b). In addition, the samples have high Fe index (Fe2O3 T/(Fe2O3 T + MgO)) values of 0.71 to 0.96, showing an affinity to those of ferroan granites (Frost et al. Reference Frost, Bell, Frost and Chamberlain2001).
Table 3. Major (wt %) and trace (ppm) elemental compositions for the Chengwan granite from the South Qinling block

ud – undetermined; * – data are from Li (Reference Li2018).

Fig. 5. (a) CIPW-normative QAP plot for classification of the Chengwan granite. (b) ANK versus A/CNK plot showing the metaluminous to peraluminous nature of the Chengwan granite; A – Al2O3; N – Na2O; K – K2O; C – CaO (all in molar proportion).
The Chengwan granite is characterized by low Cr (0.91–24.8 ppm) and Ni (0.73–7.68 ppm) contents. Except for one sample (19CW05) with the highest loss on ignition (LOI) values (3.03 wt %) and extremely high K2O contents (6.24 wt %), which may suggest an influence of later hydrothermal alteration, the remaining samples have Zr/Hf ratios (16.3–34.5) distinctly lower than the primitive mantle (Zr/Hf = 37.1; McDonough & Sun, Reference McDonough and Sun1995). All the samples show enriched light rare earth element (LREE) and flat heavy rare earth element (HREE) patterns, and have pronounced negative Eu anomalies (Eu/Eu* = 0.19–0.82) (Fig. 6a). It is notable that a clear tetrad effect can be identified in the REE patterns of the Chengwan granite. On the primitive mantle-normalized multi-element diagram, the Chengwan granite is characterized by depletion of Nb, Ta, Ti, P, Sr and Ba, and enrichment of Rb, K and Pb (Fig. 6b).

Fig. 6. (a) Chondrite-normalized REE and (b) primitive mantle-normalized multi-element diagrams for the Chengwan granite. Primitive mantle-normalized values are from McDonough & Sun (Reference McDonough and Sun1995). Chondrite-normalized values are from Sun & McDonough (Reference Sun, McDonough, Saunders and Norry1989). Grey area denotes the documented Neoproterozoic highly fractionated A-type granites in the western Yangtze block (Huang et al. Reference Huang, Xu, Li, Li, Lan, Zhang, Liu, Wang, Li, Luo and Yang2008).
5.d. Whole-rock Nd isotopes
Sm–Nd isotopic compositions for the Chengwan granite are listed in Table 4. εNd(t) values were calculated using their crystallization age (t = 810 Ma). The granites have 147Sm/144Nd ratios of between 0.1061 and 0.1399 and 143Nd/144Nd ratios of between 0.511768 and 0.512026, corresponding to negative εNd(t) values from −8.9 to −4.5 and depleted mantle Nd isotopic model ages (TDM-Nd) from 2.34 to 1.84 Ga.
Table 4. Sm–Nd isotopic compositions for the Chengwan granite from the South Qinling block

147Sm/144Nd is calculated by using Sm and Nd contents determined by ICP-MS and measured 143Nd/144Nd ratios by TIMS.
In TDM-Nd calculation, ratios of (143Nd/144Nd)DM = 0.51315 and (147Sm/144Nd)DM = 0.2137.
In εNd(t) calculation, ratios of (143Nd/144Nd)CHUR and (147Sm/144Nd)CHUR are 0.512638 and 0.1967, respectively.
I Nd denotes initial ratio of 143Nd/144Nd at t = 810 Ma.
6. Discussion
6.a. Petrogenetic type: highly fractionated A-type granite
Granites can be divided into I-, S-, M- and A-types according to the nature of their sources and petrogenesis (Pitcher, Reference Pitcher and Hsu1982, Reference Pitcher1993; Chappell & White, Reference Chappell and White1992). The Chengwan granite is composed mainly of potash feldspar, quartz and plagioclase with a minor amount of biotite, and no Al-rich minerals such as cordierite, garnet or muscovite have been observed, which is distinctly different from the composition of typical S-type granitoids (King et al. Reference King, White, Chappell and Allen1997). Some granitic samples are weakly peraluminous or even metaluminous (A/CNK <1.1), also indicating that the Chengwan granite does not belong to the S-type granitoids. In addition, amphibole is not found in the samples, and a marked decrease in P2O5 with increasing SiO2 has not been observed in the Chengwan granite, which discriminates it from the I-type granites when apatite reaches saturation in felsic magmas (Chappell, Reference Chappell1999). In contrast, the Chengwan granite exhibits petrological and geochemical features of typical A-type granites (e.g. Whalen et al. Reference Whalen, Currie and Chappell1987; Frost et al. Reference Frost, Bell, Frost and Chamberlain2001), including a high alkali content, Fe index, Ga/Al ratio, flat HREE pattern and pronounced negative Eu, Ba, Sr and Ti anomalies. In addition, it is generally accepted that A-type granites are derived from relative high-temperature magmas. The zircons in the two Chengwan samples have a wide range of Ti contents of between 3.57 and 36.2 ppm, corresponding to variable Ti-in-zircon temperatures of 657–883 °C with a median of 792 °C (Table 1). The calculated temperature should be regarded as the minimum estimate owing to the absence of rutile and the assumption of αTiO2 = 1 to calculate the zircon formation temperature using the Ti-in-zircon thermometer (Ferry & Watson, Reference Ferry and Watson2007). Nevertheless, this temperature is higher than that of typical I- and S-type granites (Miller et al. Reference Miller, McDowell and Mapes2003; Bonin, Reference Bonin2007). Differing from peralkaline A-type granites, some of the Chengwan granitic samples can be strongly peraluminous, indicating that they belong to aluminous A-type granites (Turner et al. Reference Turner, Foden and Morrison1992).
It is notable that the Chengwan granite has some highly fractionated geochemical features, such as evolved SiO2 contents (>72 wt %, up to 77.4 wt %), low Zr/Hf ratios and REE tetrad effects (the M-type with convex curves in the REE patterns). In addition, potash feldspars in the Chengwan granite are mainly composed of perthite and microcline. Field observations show that the granites are spatially associated with a couple of pegmatites. These lines of evidence indicate that the Chengwan granite may have experienced intensive fractionation. It is not easy to distinguish highly fractionated I-type granites from A-type granites, especially aluminous A-type granites. Whalen et al. (Reference Whalen, Currie and Chappell1987) found that A-type granites are low in Al but high in Ga contents, and thus proposed that A-type granites could have higher Ga/Al ratios than the other types of granites. However, high Ga/Al ratios can also be achieved in highly fractionated I-type or S-type granites (Breiter et al. Reference Breiter, Gardenová, Kanický and Vaculovič2013; Wu et al. Reference Wu, Liu, Ji, Wang and Yang2017). On the other hand, if the A-type granites experienced extensive fractionation, then the felsic end-member of the A-type suite will geochemically overlap with the highly fractionated I- and S-type granites (King et al. Reference King, Chappell, Allen and White2001). Wu et al. (Reference Wu, Liu, Ji, Wang and Yang2017) proposed a classification method for further verification, on the basis that the fractional crystallization of the A-type granites and I- or S-type granites would have two different kinds of evolutionary trends on the plot of Zr versus 10 000 × Ga/Al. In this study, the Chengwan granite exhibits a clear trend showing the crystal fractionation process decreases the Ga/Al ratios, making it move from A-type to highly fractionated granite on the Zr versus 10 000 × Ga/Al diagram (Fig. 7). Therefore, petrological and geochemical features all suggest that the middle Neoproterozoic Chengwan granite is a highly fractionated A-type granite.
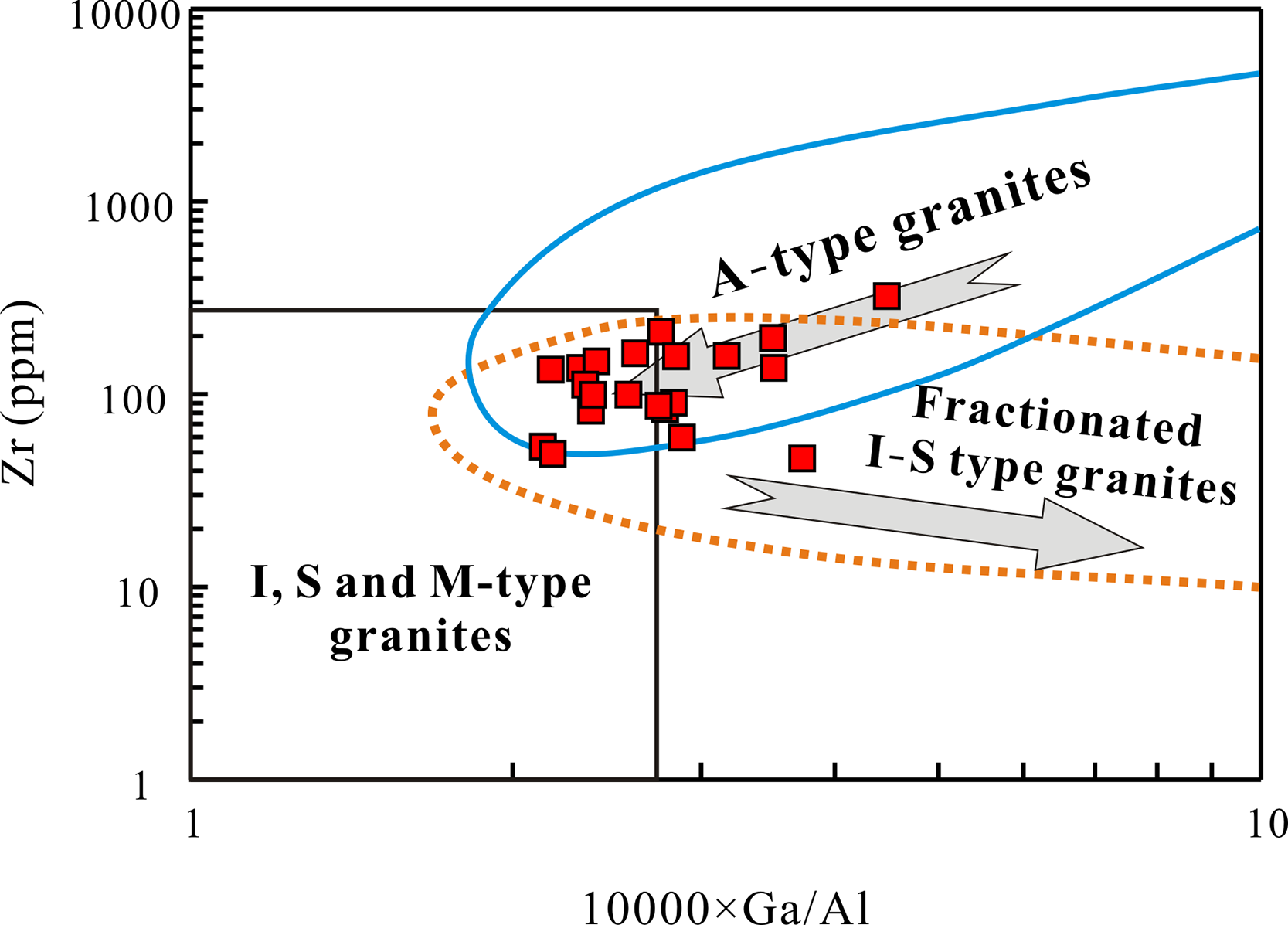
Fig. 7. Discrimination diagram for A-type and fractionated I- and S-type granites (modified after Whalen et al. Reference Whalen, Currie and Chappell1987 and Wu et al. Reference Wu, Liu, Ji, Wang and Yang2017). Note the Chengwan granite has a geochemical trend resembling A-type granites.
6.b. Fractional crystallization of the Chengwan granite
The geochemical compositions of the Chengwan granite suggest that it experienced a relatively high degree of fractional crystallization. Except for sample 19CW05, the granitic samples exhibit decreases in Al2O3, CaO and Na2O and increases in K2O with increasing SiO2, suggesting crystal fractionation of plagioclase rather than potash feldspar (Fig. 8). The presence of negative Eu, Sr and Ba anomalies in the Chengwan granite is also indicative of fractional crystallization of plagioclase. By comparing the compositions of the Chengwan granite with the vectors of fractionation of different phases, it can be inferred that fractionation of plagioclase (probably albite) is important in the evolution of the Chengwan granite (Fig. 9a). Phosphate minerals such as apatite and monazite are not the main fractionated phases, which is supported by the insignificant correlation between the SiO2 and P2O5 concentrations (Fig. 8).

Fig. 8. Harker diagrams of selected major elements against SiO2 for the Chengwan granite. Note that the granite might experience fractional crystallization of plagioclase and Fe–Ti oxides.

Fig. 9. Diagrams of (a) Ba versus Sr and (b) Ti/Yb versus Nb/La for the Chengwan granite. The former reveals that fractionation of plagioclase plays an important role in the evolution of the Chengwan A-type granite, whereas the latter shows that the Ti/Yb values of the Chengwan granite are not positively correlated to Nb/La ratios, suggesting the Nb and Ta negative anomalies in the granite are not caused by fractionation of the Fe–Ti oxides. Partition coefficients of Sr and Ba are from Arth (Reference Arth1976).
All the samples in this study show negative correlations between TiO2, Fe2O3 T and SiO2 (Fig. 8), indicating that Fe–Ti oxides (e.g. ilmenite) are fractionated from the granitic magma. Ti-bearing amphibole could not be the major fractionation phase for the Chengwan granite, because the removal of amphibole would decrease the Dy/Yb and Nb/Ta ratios but increase Zr/Sm ratios, as well as increase Sr/Y ratios (Foley et al. Reference Foley, Tiepolo and Vannucci2002), which is not observed in the Chengwan samples. Experimental studies revealed that Fe–Ti oxides dominate the Nb–Ta budget during the magma evolution processes (Schmidt et al. Reference Schmidt, Dardon, Chazot and Vannucci2004; Xiong et al. Reference Xiong, Adam and Green2005). Therefore, the negative Nb and Ta anomalies observed in the Chengwan granite might be caused by the removal of these Fe–Ti oxides. However, Fe–Ti oxide fractionation cannot be the main reason for the negative Nb and Ta anomalies in the Chengwan granite, as a positive correlation between Nb/La and TiO2/Yb ratios has not been observed (Fig. 9b). Therefore, the negative Nb and Ta anomalies in the Chengwan granite are likely to be inherited from its source rocks.
Taken together, fractional crystallization plays an important role in the generation of the Neoproterozoic Chengwan granite in the SQB. During the formation of the granite, plagioclase and Fe–Ti oxides are the main fractionated minerals from the primary magma.
6.c. Petrogenesis of the Chengwan highly fractionated A-type granite
Diverse mineralogical and geochemical compositions of A-type granites worldwide attest to large variations in the magma source and evolution processes, thus requiring multiple petrogenetic processes. So far, several models have been proposed for the origin of A-type granites, including (1) direct fractionation products of mantle-derived alkaline basalts (Turner et al. Reference Turner, Foden and Morrison1992); (2) hybridization between anatectic granitic and mantle-derived fluids and magmas (Yang et al. Reference Yang, Wu, Chung, Wilde and Chu2006); (3) a low degree of partial melting of F- and Cl-enriched granulitic residue depleted by prior extraction of granitic melt (Collins et al. Reference Collins, Beams, White and Chappell1982; King et al. Reference King, White, Chappell and Allen1997); (4) partial melting of calc-alkaline granitoids (tonalite or granodiorite) at low pressure or high temperatures (Skjerlie et al. Reference Skjerlie, Patiño Douce and Johnston1993; Patiño Douce, Reference Patiño Douce1997); and (5) high-temperature melting of granulite-facies metasedimentary rocks (Collins et al. Reference Collins, Beams, White and Chappell1982; Li et al. Reference Li, Lin, Xing, Jiang and Xia2018).
The crux of the matter is whether mantle materials have been involved in the generation of the granites. Given the occurrence of contemporaneous mantle-derived mafic igneous rocks in the study area (e.g. Deng et al. Reference Deng, Wang, Wang, Wang, Qiu, Yang, Du, Cui and Zhou2013; Chen et al. Reference Chen, Mao, Hu, Yang, Yang, Kong and Meng2017; Liu & Zhao, Reference Liu and Zhao2019), it is appealing to envision that a mafic magma progenitor produced the Chengwan granite. However, the petrogenesis of the granite in this study may not be correlated to mantle-derived magmas. First of all, compared to the Chengwan granite with an outcrop area of over 80 km2, only a small amount of contemporary mafic rocks occur in the area. To produce the Chengwan granite by fractionation, a huge volume of mafic magma is required. Secondly, the Chengwan granite has relatively uniform whole-rock εNd(t) and zircon εHf(t) values, and does not contain mafic microgranular enclaves in the field investigation, which precludes a magma mixing origin for the granite. Thirdly, the granite has high SiO2 and low Cr, Ni and MgO contents, suggesting that the mantle contribution in the generation of the Chengwan granite is insignificant. Therefore, the Chengwan granite cannot be derived from mantle-derived magmas, although crystal fractionation was responsible for its compositional variation.
A diversity of crustal protoliths has been envisaged in various crustal melting models, including dry residual granulitic lower crust, granulite-facies metasedimentary rocks or calc-alkaline felsic igneous rocks. Experimental studies reveal that partial melting of crustal rocks can produce refractory granulitic residues, which have low (Na2O + K2O)/Al2O3 and TiO2/MgO ratios (Patiño Douce & Beard, Reference Patiño Douce and Beard1995). Remelting of these residues is capable of generating A-type granites with low (Na2O + K2O)/Al2O3 and TiO2/MgO ratios (Creaser et al. Reference Creaser, Price and Wormald1991; Patiño Douce, Reference Patiño Douce1997). In contrast, Patiño Douce (Reference Patiño Douce1997) proposed that high-temperature dehydration melting of granitoids with a plagioclase-rich residual at 0.4–0.8 GPa can produce SiO2-enriched A-type magmas, which are depleted in Al2O3 relative to alkalis and in MgO relative to TiO2. Therefore, the crustal source region of the A-type granites can be effectively distinguished by their (Na2O + K2O)/Al2O3 and TiO2/MgO ratios. The Chengwan granite has relatively high (Na2O + K2O)/Al2O3 and TiO2/MgO ratios, ranging from 0.52 to 0.69 and from 0.34 to 2.14, respectively. On the TiO2/MgO versus (Na2O + K2O)/Al2O3 diagram for the less-evolved (SiO2 <74 wt %) samples, the Chengwan granite is distinct from the granites derived from partial melting of refractory granulitic residues, but similar to those A-type granites derived from partial melting of tonalites, suggesting that it might be generated from tonalitic partial melting (Fig. 10a).

Fig. 10. (a) TiO2/MgO versus (Na2O + K2O)/Al2O3. (b) Molecular normative An–Ab–Or plots for the less-evolved (SiO2 <74 wt %) Chengwan granite, showing that the granite might be derived from partial melting of tonalites under fluid-absent conditions. Fields of tonalite- and granulite residue-derived A-type granitoids are from Peng, M. et al. (Reference Peng, Wu, Gao, Zhang, Wang, Liu, Gong, Zhou, Hu and Liu2012) and Qiu et al. (Reference Qiu, Zhao, Yang, Lu, Jiang and Wu2018), respectively. Experimental greywacke- and granitoid-derived melts are compiled from Yang, H. et al. (Reference Yang, Zhang, Luo, Gao, Guo and Xu2016).
It is suggested that melting of previously dehydrated metasedimentary rocks can generate A-type granite (Anderson & Thomas, Reference Anderson and Thomas1985), which might also explain the peraluminous affinity of the Chengwan granitic rocks. However, the likelihood of having granulite-facies metasedimentary rocks as the protolith is overshadowed by complications arising from the fact that dehydration melting of such rocks yields dominant strongly peraluminous (A/CNK >1.1) products (Anderson & Thomas, Reference Anderson and Thomas1985), which contradicts with the fact that half of the Chengwan samples are weakly peraluminous or even metaluminous. In addition, the positive εHf(t) values for the zircons indicate that the source region of the granitic rocks is mainly composed of juvenile crustal materials, which precludes highly evolved sedimentary rocks from being the source.
Actually, partial melting of granitoids under fluid-absent or water-present conditions can also produce peraluminous A-type granites (e.g. Conrad et al. Reference Conrad, Nicholls and Wall1988; Watkins et al. Reference Watkins, Clemens and Treloar2007). Compared to the granitic melts generated under fluid-absent conditions, the melts generated under water-present conditions commonly have higher Na2O and lower SiO2, and thus are more tonalitic to trondhjemitic in composition. In contrast, the tonalite-produced melts under fluid-absent conditions would display elevated potassium (and SiO2) contents relative to their parental rocks and usually fall into the ‘granite’ field on the normative An–Ab–Or plot (Watkins et al. Reference Watkins, Clemens and Treloar2007) (Fig. 10b). In addition, the melts derived from water-present melting are characterized by relatively high Eu/Eu* ratios because of the destabilization of plagioclase caused by the addition of H2O and the absence of potash feldspar in the source (Weinberg & Hasalova, Reference Weinberg and Hasalova2015). The Chengwan granite in this study has relatively high K2O/Na2O ratios (mostly >1.0) and pronounced negative Eu anomalies (Fig. 6a), and they all fall into the granite field on the normative An–Ab–Or plot (Fig. 10b), which is not consistent with water-present melting. Therefore, the Chengwan granite might have been produced under fluid-absent conditions. The high K2O contents and K2O/Na2O ratios of the Chengwan granite indicate that the breakdown of biotite or muscovite was probably involved during fluid-absent melting (Patiño Douce & Beard, Reference Patiño Douce and Beard1995; Weinberg & Hasalova, Reference Weinberg and Hasalova2015). It is indicated that biotite dehydration melting reactions occur at higher temperatures (˜750–850 °C) than muscovite (˜650–750 °C) (Weinberg & Hasalova, Reference Weinberg and Hasalova2015). The Chengwan granite has relatively high average TTi-in-zircon values of 788 °C, implying that it is probably derived from fluid-absent biotite melting processes. Moreover, the melts generated by muscovite dehydration melting are commonly more enriched in Pb relative to Ba. The Chengwan granite is characterized by low Pb (9.16–19.2 ppm) and high Ba (221–1461 ppm) contents, which is also consistent with the high-temperature granites produced by crustal melting associated with biotite breakdown. In summary, we propose that the Chengwan granite was formed dominantly from partial melting of tonalitic rocks by biotite breakdown under fluid-absent conditions.
It is noteworthy that the Chengwan granite has negative whole-rock εNd(t) (−8.9 ˜ −4.5) but positive zircon εHf(t) (2.5˜4.4) values, illustrating a Hf–Nd isotopic decoupling (Fig. 11). This decoupling might be caused by later hydrothermal or high-grade (granulite-facies) metamorphic events, as the whole-rock Sm–Nd isotopic system could be disturbed by fluid–rock interaction or metamorphism (Pronost et al. Reference Pronost, Harris and Pin2008). However, the samples in this study did not experience high-grade metamorphism and no obvious fluid–rock interactions were shown based on both field and microscopic observations, precluding their Nd isotopic compositions from being affected by disturbances. In addition, the decoupling could also be related to assimilation of wall rock after zircon crystallization. In this scenario, zircon is an early-crystallized mineral in the felsic magma and preserves its primary Hf isotope composition during magma crystallization, whereas the whole-rock Sm–Nd system was readily affected by the wall rock and hence gave lower εNd(t) values. However, this process will result in a relatively large variation in Nd isotopic compositions, which is not observed in the Chengwan granite. In addition, correlations of εNd(t) values with SiO2 are also not recognized, inconsistent with the assimilation process. Alternatively, the Hf–Nd isotopic decoupling was genetically related to the existence of garnet as a residual phase in its magmatic source. Melt extraction in the presence of garnet will produce higher 176Lu/177Hf ratios than 147Sm/144Nd ratios in the restite, because the HREEs are highly compatible in garnet (e.g. van Westrenen et al. Reference van Westrenen, Blundy and Wood1999). This process can result in the preferential retention of Lu over Hf in the restite and thus HREE depletion in the melts. However, this is not consistent with the A-type affinity of the granites. In addition, the REE patterns of the Chengwan granite also do not support residual garnet in the source, because a progressive decrease in HREE is expected.

Fig. 11. Diagram of εHf(t) versus εNd(t) showing Hf–Nd isotopic decoupling for the Chengwan granite. The field of the Hf–Nd crust–mantle array is from Vervoort et al. (Reference Vervoort, Patchett, Blichert-Toft and Albarède1999).
Abnormal enrichment of radiogenic Hf isotopes in crustal rocks is principally related to anomalously high Lu/Hf ratios in the magmatic source. Disequilibrium melting of zircon during crustal anatexis could result in Hf isotope heterogeneity (Tang et al. Reference Tang, Wang, Shu, Wang, Yang and Gopon2014). Zircon is refractory during both melting and dissolution processes while mafic minerals that contain Lu and Hf (e.g. biotite) tend to break down (melt) prior to zircon. As mentioned above, the Chengwan granite was derived from partial melting of tonalitic rocks; it is thus likely that disequilibrium processes during melting are the cause of Hf–Nd isotopic decoupling (Huang et al. Reference Huang, Niu and Mo2017; Wang et al. Reference Wang, Wang, Cai, Goldstein and Yang2018). During partial melting of these crustal rocks, the zircon in the tonalites would be resistant to melting and the Lu–Hf system might be dominated by those non-zircon components. In contrast, the Sm–Nd system would be dominated by breakdown of biotite in the tonalitic source. Therefore, we hypothesize that the Hf–Nd isotopic decoupling observed in the Chengwan granite might be due to disequilibrium partial melting of tonalitic rocks. The positive εHf(t) values for the zircons indicate the source region of the Chengwan granite is mainly composed of juvenile crustal material, while the TDM-Hf ages (1327˜1201 Ma) further indicate that the extraction of juvenile crust from the depleted mantle was during Mesoproterozoic time. As discussed above, the Nb and Ta depletion in the Chengwan granite did not result from crystal fractionation, but was mostly inherited from the source. Taken together, we thus speculate that the source of the Chengwan granite was juvenile crustal rocks.
6.d. Tectonic implications
Neoproterozoic magmatic and sedimentary rocks, in the time interval of ˜950–630 Ma, are extensively distributed in the SQB (e.g. Deng et al. Reference Deng, Wang, Wang, Wang, Qiu, Yang, Du, Cui and Zhou2013; Xu et al. Reference Xu, Yang, Polat and Yang2016; Yang, Y. N. et al. Reference Yang, Wang, Li and Li2016; Liu & Zhao et al. Reference Liu and Zhao2019; Qiu et al. Reference Qiu, Jiang, Lu and Khattak2021). These rocks have been highlighted in the last few decades for their possible connection with the evolution of the Rodinia supercontinent. In a recent study, magmatic events in the SQB were divided into three cycles: ˜920–820 Ma, ˜800–710 Ma and ˜700–620 Ma (Zhu et al. Reference Zhu, Chen, Nie, Siebel, Yang, Xue and Zhai2014). Although there is a general agreement that the late Neoproterozoic magmatic rocks were formed in a rifting-related environment, the origin and tectonic settings of the earlier two cycles of magmatism have been the subject of a long-lasting dispute (Deng et al. Reference Deng, Wang, Wang, Wang, Qiu, Yang, Du, Cui and Zhou2013; Hu et al. Reference Hu, Mao, Tian and Li2015; Xie et al. Reference Xie, Hu, Mao, Kong, Yang, Yang and Guo2019). Recently, with new geological surveys and increasing high-precision geochronological and geochemical studies of the early Neoproterozoic magmatic rocks in the SQB, more and more evidence suggests that plate subduction might have existed in the SQB during late Mesoproterozoic to early Neoproterozoic times (Dong et al. Reference Dong, Liu, Santosh, Zhang, Chen, Chen and Zhao2011; Hu et al. Reference Hu, Mao, Tian and Li2015, Reference Hu, Liu, Qu and Chen2019; Xu et al. Reference Xu, Yang, Polat and Yang2016). For example, Xu et al. (Reference Xu, Yang, Polat and Yang2016) reported ˜860 Ma high-Mg adakitic rocks and contemporaneous arc-related calc-alkaline mafic intrusions in the Suizao terrane, which may represent typical arc-related magmatic rocks in the SQB. However, the bone of contention focuses on the tectonic setting of those middle Neoproterozoic magmatic rocks with ages between ˜830 Ma and 750 Ma. One opinion is that these magmatic rocks were generated in an anorogenic setting, probably related to a long-lasting continental rifting event corresponding to the break-up of the Rodinia supercontinent. The proponents of this model suggest that the orogenic event in the SQB and the northern margin of the Yangtze block was terminated at ˜840–830 Ma, and that the region was under extension and became a long-lasting continental rift during 820–620 Ma (Yang, Y. N. et al. Reference Yang, Wang, Li and Li2016; Zhang et al. Reference Zhang, Sun, Zhang, Ao and Santosh2016; Qiu et al. Reference Qiu, Jiang, Lu and Khattak2021). Therefore, they indicate that the magmatic rocks in the region may represent melting products associated with the development of the rift. However, a competing model suggests that the orogenic events in the SQB may last until ˜750 Ma or even later (Liu et al. Reference Liu, Zhao, Cawood and Wang2018; Zhao & Asimow, Reference Zhao and Asimow2018) and that the middle Neoproterozoic magmatic rocks in the region were formed in a continental arc (Dong et al. Reference Dong, Liu, Santosh, Zhang, Chen, Chen and Zhao2011; Zhao et al. Reference Zhao, Li, Liu and Wang2018) or intra-oceanic arc setting (Hu et al. Reference Hu, Mao, Tian and Li2015; Xie et al. Reference Xie, Hu, Mao, Kong, Yang, Yang and Guo2019), mainly on the basis of the arc-related affinity of these magmatic rocks.
The discovery of ˜816–808 Ma highly fractionated A-type granites in this study may shed some new light on the tectonic evolution of the SQB and the northern margin of the Yangtze block. A-type granites are rather enigmatic not only regarding their petrogenesis but also in terms of their tectonic setting (Dall’Agnol et al. Reference Dall’Agnol, Frost and Rämö2012). It is widely accepted that the occurrence of A-type granites is commonly associated with an extensional environment, either in a post-collisional or anorogenic setting (Collins et al. Reference Collins, Beams, White and Chappell1982; Eby, Reference Eby1992; Turner et al. Reference Turner, Foden and Morrison1992; Teixeira et al. Reference Teixeira, Dall’Agnol, Santos, Kemp and Evans2019). According to the geochemical subdivision by Eby (Reference Eby1992), A-type granites can be further divided into A1 and A2 subgroups. A1-subgroup granites have geochemical characteristics similar to those magmas derived from oceanic island basalt (OIB)-like sources and emplaced in an anorogenic setting, whereas A2-subgroup granites are derived from melting of continental crust and emplaced in a post-collisional environment. Generally, A1-subgroup granites have relatively higher Nb/Y ratios than the A2-subgroup. The Chengwan granite belongs to the A2-subgroup on the Rb/Nb versus Y/Nb diagram (Fig. 12a). In addition, it plots within the post-collisional granite field on the tectonic discrimination diagram (Fig. 12b), also supporting that its generation may have occurred shortly after the collision. The post-collisional extensional setting is further supported by the occurrence of ˜820–810 Ma OIB and mid-ocean ridge (MORB)-like mafic rocks in the Suizao terrane of the SQB (Chen et al. Reference Chen, Mao, Hu, Yang, Yang, Kong and Meng2017; Liu & Zhao, Reference Liu and Zhao2019; Xie et al. Reference Xie, Hu, Mao, Kong, Yang, Yang and Guo2019).

Fig. 12. (a) Rb/Nb versus Y/Nb (Eby, Reference Eby1992) and (b) Rb versus Y + Nb (Pearce et al. Reference Pearce, Harris and Tindle1984) diagrams for the Chengwan granite. WPG – within-plate granite; VAG – volcanic arc granite; ORG – ocean ridge granite; syn-COLG – syncollisional granite; post-COLG – post-collisional granite. Note the Chengwan granite represents post-collisional A2-subgroup granites.
In view of the above argument, we suggest that a tectonic switch in the geodynamic regime from convergence to extension might have occurred at ˜820–810 Ma in the SQB (Fig. 13). This interpretation is also consistent with general geological observations in the SQB, including (1) ˜950–840 Ma arc-related high-Mg adakitic granitoids and arc-related volcanic suites in the SQB, relating to oceanic plate subduction (Shi et al. Reference Shi, Liu, Zhang, Miao, Zhang and Xue2007; Xu et al. Reference Xu, Yang, Polat and Yang2016); (2) ˜837–818 Ma high-grade metamorphism involving a clockwise P–T path in the Douling complex of the SQB, suggesting the region experienced crustal thickening followed by extensional collapse (Shen et al. Reference Shen, Zhang and Liu1997; Hu et al. Reference Hu, Liu, Qu and Chen2019); (3) ˜834 Ma syncollisional diorite in the SQB (Wang et al. Reference Wang, Xu, Santosh and Zeng2021); (4) extension-related Chengwan A-type granite emplaced at ˜816–808 Ma (Li, Reference Li2018; this study), along with ˜817 Ma OIB and ˜815 Ma MORB-like mafic rocks in the region (Chen et al. Reference Chen, Mao, Hu, Yang, Yang, Kong and Meng2017; Xie et al. Reference Xie, Hu, Mao, Kong, Yang, Yang and Guo2019); (5) a rifting-related basin initiated at ˜780 Ma as suggested by the Wudang Group (Ling et al. Reference Ling, Ren, Duan, Liu, Mao, Peng, Liu, Cheng and Yang2008; Xue et al. Reference Xue, Ma and Song2011; Yang, Y. N. et al. Reference Yang, Wang, Li and Li2016); and (6) detrital zircons from the Neoproterozoic sedimentary rocks of the Suizao terrane revealing that low-δ18O zircon grains appeared sporadically in the northern Yangtze block at as early as ˜840 Ma, relating to the onset of post-orogenic extension (Yang, Y. N. et al. Reference Yang, Wang, Li and Li2016).

Fig. 13. Cartoon model for the Neoproterozoic tectonic evolution of the Suizao terrane. It hypothesizes that (a) oceanic subduction occurred at ˜950–840 Ma, followed by (b) an arc–continental collision taking place at ˜837–818 Ma, resulting in metamorphism and reworking of pre-existing crustal rocks, forming syncollisional granite. (c) Local lithospheric extension is assumed to trigger extension-related magmatism at ~817–800 Ma.
These records constitute a reasonable successive relationship in timing from the ~950–840 Ma subduction-related, through the ~840–820 Ma collision-related, to the ~820–630 Ma rift-related geological events in the region. Therefore, the Chengwan granite in the Suizao terrane of the SQB may represent extensional collapse following collisional events. The geochemical and Hf–Nd isotopic compositions suggest that the source materials of the Chengwan highly fractionated A-type granite are juvenile arc-related crustal rocks extracted from the depleted mantle during late Mesoproterozoic time. Melting of these crustal rocks was then induced by heat from mantle-derived hot mafic magmas during crustal extension. Extensive fractionation of plagioclase and Fe–Ti oxides at shallow crustal levels finally resulted in the formation of the Chengwan granite. This interpretation is broadly in agreement with the rifting-related tectonic evolution model, but at odds with the arc models. It is also supported by the recent study suggesting that the geothermal gradient in the SQB during ˜820–780 Ma is ~18–25 °C km−1, which is significantly lower than that of typical magmatic arcs (˜40 °C km−1) (Hu et al. Reference Hu, Liu, Qu and Chen2019). The absence of coeval arc-related magmatism, such as high-Mg andesite, adakitic rocks and island arc basalt (IAB)-like mafic rocks in the SQB is further evidence against a magmatic arc setting during middle Neoproterozoic time.
7. Conclusions
From our study we can draw the following conclusions:
-
(1) The Chengwan granite was emplaced at ~816–808 Ma, incompatible with previous deductions that it was formed during Palaeozoic time.
-
(2) The Chengwan granite is highly fractionated A2-type granite, and derived from partial melting of juvenile tonalitic rocks under fluid-absent conditions in a post-orogenic setting.
-
(3) The occurrence of highly fractionated A-type granite in the SQB indicates that a tectonic regime switch from convergence to extension might have occurred at ˜820–810 Ma, in agreement with the rifting-related tectonic model.
Acknowledgements
The authors are grateful to Drs Xi-Run Tong and Wen-Wu Yang for their considerable help during the LA-(MC)-ICP-MS analyses at the Wuhan Centre of China Geological Survey. Editor Prof. Goodenough and two anonymous reviewers are kindly thanked for their thorough reviews and constructive suggestions. This study was supported by the National Science Foundation of China (Grant 41303026), the Project of China Geological Survey (Nos. DD20190050), and the Open Fund of the Research Centre for Petrogenesis and Mineralization of Granitoid Rocks, China Geological Survey (No. PMGR202003).