Introduction
The secretory epithelia of the stria vascularis, the vestibular dark cells, the planum semilunatum and the endolymphatic sac are involved in inner-ear fluid production. In addition, Reissner's membrane, the endolymphatic duct and sac, sulcus cells, spiral limbus cells, and supporting cells such as Deiter's cells, Boettcher cells and Hensen cells, are responsible for fine regulation of inner-ear fluids, including maintenance of ion and osmolarity gradients.
Morphological similarities between vestibular dark cells and strial marginal cells have long been recognised.Reference Kimura1 However, functional similarity was previously deemed unlikely, as the marginal cells were thought to be the direct source of the large positive endocochlear potential. Indeed, the endocochlear potential, of about 80 mV, has no equivalent in the vestibular labyrinth; the endovestibular potential in the semicircular canals is ± 1 mV.Reference Marcus, Liu and Wangemann2
Both vestibular dark cells and strial marginal cells have similar ion transport mechanisms under similar regulatory control, and generate a similar transepithelial voltage and transepithelial resistance; however, their canal characteristics and absolute levels of conductance are different.Reference Wangemann3 Both cell types have a distinct ultrastructural appearance, with increased surface area and microvilli located at the apical and basal sides, indicating involvement in fluid transport. Their extensively infolded basolateral membranes enclose numerous large mitochondria with a large energy output, their extensive, interdigitating processes form a complex network with neighbouring cells (e.g. melanocytes), and their numerous cytoplasmic processes leave little cytoplasm proper around the nuclear compartment.
An increase in knowledge of the inner ear over recent years has enabled the development of promising approaches to therapy, e.g. in cases of Ménière's disease. This review describes the current state of such knowledge. It will become obvious that further research is needed in order to understand the role of regulatory mechanisms and transporter systems in inner-ear pathophysiology and disease (Table 2)
Histological characteristics
Dark cells
Three types of cells are localised in the epithelium of vestibular organs: sensory cells, secretory dark cells and non-secretory cells (transitional and undifferentiated cells). This epithelium is characterised by cytokeratin, ZO-1 protein, occludin, tight junctions and kinocilia.Reference Milhaud, Nicolas and Bartolami4
The dark cells are located in the utricle covering almost the entire posterior wall and the periphery of the anterior wall. In the ampullae, the dark cells are localised at the base of the cristae on the canal side. Contrastingly, they are not found in the wall of the saccule.Reference Kimura1 The dark cells are structurally similar to ion-transporting epithelia in the renal tubules, ciliary plexus, choroid plexus and the like. Microvilli located at the apical and basal sides of the dark cells indicate involvement in fluid transport.Reference Nakai and Hilding5 The vestibular dark cells and the strial marginal cells possess basolateral infoldings with mitochondria providing the energy for active transport mechanisms. These cells contain condensed mitochondria which show a high level of bioenergetic or biosynthetic processes. In contrast, the light cells of the vestibular system possess intermediate or orthodoxic mitochondria.Reference Beketova and Sekenova6 On the luminal surface are unique meshwork structures composed of cytoplasmic processes in a reticular arrangement. Based on their findings in guinea pigs, Kawamata et al. proposed involvement in the metabolism of otoconia and fluid transport.Reference Kawamata, Harada and Tagashira7 Interestingly, these basolateral infoldings are closely interwoven with melanocyte processes. The melanocytes have calcium-binding capacity and probably participate in calcium homeostasis.Reference Meyer zum Gottesberge8 Pinocytotic vesicles are frequently found within these infoldings and the melanocyte processes, indicating extensive transport mechanisms.
Stria vascularis
The stria vascularis represents one of the few epithelial types that contains capillaries. The capillaries form an extensive, branching network within the stria vascularis and are drained at the scala tympani side by collecting venules. A thickening of the strial capillary basement membrane has been suggested as the primary site of cochlear pathogenesis in Alport syndrome, which results from mutations in genes encoding the collagen chains α3 (IV), α4 (IV) and α5 (IV), preventing proper production or assembly of the type IV collagen network. The syndrome is characterised by progressive glomerular disease associated with high-frequency sensorineural hearing loss.Reference Gratton, Rao, Meehan, Askew and Cosgrove9
The stria vascularis has a higher oxygen consumption than brain tissue, and the strial capillaries are larger in diameter, with a higher haematocrit and a slower flow, than the capillaries of any other tissue type.Reference Hawkins10 Regulation is mainly performed by locally produced substances such as prostaglandin I2 and endothelial nitric synthase (eNOS), with some differences between the strial and spiral ligament vessels.Reference Konishi, Yamane, Iguchi, Takayama, Nakagawa and Sunami11 Schuknecht defined the strial type of sensorineural hearing loss that is characterised by a flat stria vascularis,Reference Castaldo and Linthicum12, Reference Schuknecht13 and reduced stria vascularis function has been implicated in the pathogenesis of presbyacusis.Reference Johnsson and Hawkins14, Reference Nadol15 It has been shown in animal models that age-related atrophy of the stria vascularis, first characterised by degeneration of marginal cells, is associated with thickening of the basement membrane in strial capillaries. Consequently, degeneration has been attributed to decreased permeability imposed by the thickened basement membrane.Reference Thomopoulos, Spicer, Gratton and Schulte16
In human inner-ear epithelia, there is a wide distribution of different cytokeratin subunits, compared with animals, providing mechanical stability and allowing characterisation of the epithelium as complex or mixed.Reference Anniko, Thornell and Ramaekers17 In contrast with cytokeratin, vimentin is located epithelially as well as subepithelially. Cytokeratin encloses the organ of Corti like a shell and is expressed more strongly at the apex than at the base, correlating with the tonotopy of the cochlea. In the stria vascularis, cytokeratin is located in the marginal cells.Reference Anniko, Arnold, Thornell, Virtanen, Ramaekers and Pfaltz18–Reference Usami, Hozawa and Shinkawa20
The complex structure of the stria vascularis and the fine differentiation of its compartments reflect its function, and these two features characterise the stria vascularis as the key structure for inner-ear fluid regulation, guaranteeing an adequate environment for stimulation (Figure 1). The stria vascularis has three cell layers: marginal cells, intermediate cells and basal cells. The stria vascularis is separated from the spiral prominence and Reissner's membrane by spindle-shaped border cells, and it possesses no basal lamina at the connection to the spiral ligament. It is possible to identify the basal cells by their brain-type glucose transporter (Glut1),Reference Ito, Spicer and Schulte21 and to distinguish between basal intermediate cells (responsible for potassium intake from the basal cells) and upper intermediate cells. The marginal cells reach both other cell types with their processes.Reference Spicer, Smythe and Schulte22 The intermediate cells of the stria vascularis are partly melanocytes, and melanin-laden endosomes exist in the basal cells as well.Reference Fukazawa, Sakagami, Umemoto and Semda23 The melanocytes are connected by connexins 26 and 32.Reference Masuda, Usami, Yamazaki, Takumi, Shinkawa and Kurashima24 It has been shown that melanocyte-deficient rats (probably due to defects in neural crest cell migration) possess a flat stria vascularis with absent intermediate cells and poor interdigitation of marginal cells. Consequently, a reduced or absent endolymphatic potential and an elevated auditory brainstem response threshold are recorded.Reference Kitamura, Sakagami and Umemoto25
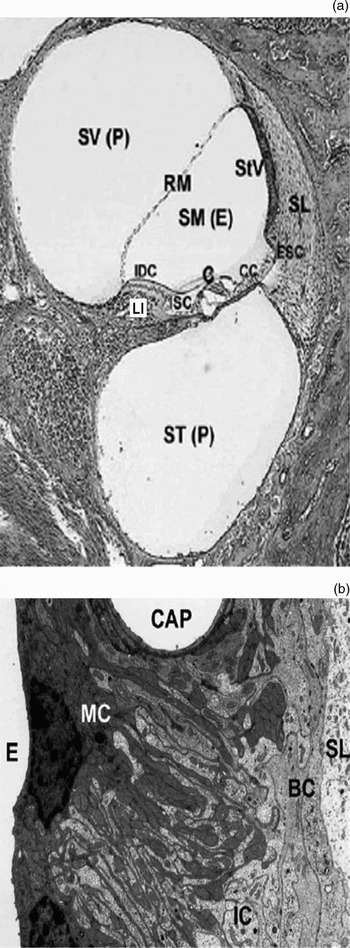
Fig. 1 a) Cross-section of one turn of the cochlea depicting the organ of Corti (C) composed of sensory hair cells and supporting cells, Claudius cells (CC), external sulcus cells (ESC), internal sulcus cells (ISC), limbus spiralis (Li), interdental cells (IDC), Reissner's membrane (RM), and stria vascularis (StV) adjacent to the fibrous spiral ligament (SL) (E endolymph, P perilymph). b) High magnification of the stria vascularis composed of marginal cells (MC) facing the endolymph (E), intermediate cells (IC), and basal cells (BC) adjacent to the spiral ligament (SL) (CAP capillary) Reproduced with permissionReference Peters, Monnens-Cor, Cremers-Jo and Curfs36.
The intrastrial space, containing fluid similar to perilymph, is embedded between two histological barriers that guarantee the specific composition of this space: on the apical side, marginal cells form a barrier against endolymph, and, on the basal side, basal cells form a barrier against the spiral ligament.Reference Ikeda and Morizono26, Reference Salt, Melchiar and Thalmann27 The barrier between intrastrial fluid and blood plasma is composed of endothelial cells. These vessels do not possess fenestrae and are connected by tight junctions.Reference Jahnke28 Tight junctions also exist between marginal cells and basal cells, but not between intermediate cells.Reference Bagger-Sjoback, Engstrom, Steinholtz and Hillerdal29
The inner ear shows a complex expression of the barrier protein claudin, underlining the complexity and importance of barrier function. In the stria vascularis, claudins one, three, four, eight, nine, 10, 12, 14 and 18 are expressed in the marginal cells, whereas the basal cells are only positive for claudin 11. In the dark cell area, claudins one, three, eight, nine, 12, 14 and 18 have been detected.Reference Kitajiri, Furuse, Morita, Saishin-Kiuchi, Kido and Ito30 Claudin four (which restricts cation passage) as well as claudins one and three and occludin are responsible for the cationic-tight permeability in marginal cells.Reference Florian, Amsheh, Lessidrensky, Todt, Bloedow and Ernst31 It has been shown in mice that absence of claudin 11 leads to impairment of the basal cell barrier, with a normal endolymph potassium content, endolymphatic potential reduction to 30 mV, and hearing loss of approximately 50 dB.Reference Gow, Davies, Southwood, Frolenkow, Chrustowski and Ng32, Reference Kitajiri, Miyamoto, Mineharu, Sonoda, Furuse and Hata33 Mutations of claudin 14 may result in autosomal recessive hearing loss (DFNB29) type autosomal recessive hearing loss.Reference Wilcox, Burton, Naz, Riazuddin, Smith and Ploplis34
The basal cells are connected by gap junctions with the intermediate cells, and are also connected with the fibrocytes of the spiral ligament. However, the marginal cells are excluded from the gap junctional network.Reference Kikuchi, Adams, Paul and Kimura35 Connexins are gap junction proteins which constitute a major system of intercellular communication, which is important in the exchange of electrolytes, second messengers and metabolites. Connexin 26 accounts for about 50 per cent of non-syndromic autosomal recessive hearing loss in European and American populations. Recently, families have been identified with a combination of heterozygous mutations of connexin 26 and 30 and hearing impairment.Reference Birkenhager, Zimmer, Maier and Schipper37 Consequently, a co-localisation could represent a distinct feature, and has been shown for the basal cell region of the stria vascularis of rats.Reference Forge, Becker, Casalotti, Edwards, Marziano and Nevill38 In addition, connexin 29 and 43 have also been found in rats,Reference Suzuki, Takamatsu and Oyamada39, Reference Yang, Liao, Su and Li40 and mutations of these proteins may also be responsible for non-syndromic hearing loss.Reference Sunose, Liu and Marcus41
Inner-ear fibrocytes
There is growing evidence that the fibrocytes of the inner ear play an important role in ion homeostasis and ion cycling and are also essential for stria vascularis and vestibular dark cell survival.Reference Doherty and Linthicum42
The functional closeness of the spiral ligament and the stria vascularis is underlined by the fact that both structures can be damaged by noise exposure and ageing, which appears to precede damage to the sensory cells in gerbils.Reference Gratton, Rao, Meehan, Askew and Cosgrove9, Reference Hirose and Liberman43–Reference Gratton, Schmiedt and Schulte45 In addition, it has been shown (in a mouse model with targeted deletion of the BRN-4 gene, causing abnormalities in type I, II and III fibrocytes) that selective damage to the lateral wall in the absence of organ of Corti damage can have important effects on hearing levels.Reference Neyroud, Tesson, Denjoy, Leibovici, Donger and Barhanin46
The spiral ligament is embedded between the multilayered stria vascularis and the otic capsule. The fibrocytes of the spiral ligament are divided into four cell types based on histological characteristics, immunostaining patterns and general location.Reference Spicer and Schulte47–Reference Takahashi and Kimura49 Type II fibrocytes are equipped with transporters that are suitable for the uptake of potassium (Na/K–adenosine triphosphatases (ATPases) and Na-K-Cl cotransporters) and are in gap junction continuity with type I and basal cells, suggesting that the spiral ligament plays a key role in the maintenance of the ionic environment and also the endocochlear potential.Reference Crouch, Sakaguchi, Lytle and Schulte50, Reference Schulte and Adams51 It is thought that potassium is taken up by type II fibrocytes and then transported to basal and intermediate stria vascularis cells via type I fibrocytes, characterising the so-called fibrocyte gap junction system. Similarly, fibrocytes in the vestibular labyrinth seem to be involved in the delivery of potassium to dark cells.Reference Kikuchi, Adams, Paul and Kimura35
Molecular aspects of secretion
Regulation of endolymph secretion
Many different hormones and messengers are responsible for stimulation or inhibition of the vestibular dark cells and the strial vascularis. This reflects, on the one hand, the necessity for fine regulation and, on the other, the need for a wide range of responses to stimulation. Vestibular dark cells and strial marginal cells are regulated by purinergic, adrenergic and muscarinic receptors, steroids, vasopressin and atrial natriuretic peptide (Table I).
Table I Regulation of endolymph composition

* As Agent Vasopressin = INN, Antidiuretic hormone = ADH, AVP = arginine vasopressin, DDAVP = (one trade name of desmopressin)
† Na+ absorption, K+ secretion. AQP = aquaporin; V2 = antidiuretic hormone receptor 2; c-AMP = cyclic adenosine monophosphate; ES = endolymphatic sac; Isc = short circuit current; ATPase = adenosine triphosphatase; Isk = short circuit current channel; ATP = adenosine triphosphate; UTP = uridine triphosphate
Table II Defective proteins in stria vascularis and vestibular dark cells, and related diseases
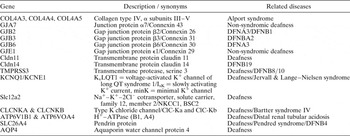
ATPase = adenosine triphosphatase
There is evidence that the stress hormones noradrenaline and adrenaline possess a key role in inner-ear homeostasis and sensory transduction. Noradrenaline seems to accelerate potassium cycling in the inner ear and potassium secretion in marginal cells.Reference Wangemann, Liu, Shimozono, Schimanski and Scofield52 β1-Adrenergic receptors, located in the basolateral plasma membrane, are the dominant subtype in the stria vascularis and the vestibular dark cells. Their activation leads to potassium secretion,Reference Wangemann, Liu, Shimozono, Schimanski and Scofield53 metabolic controlReference Ishii, Zhai and Akita54 and increased Na/K–ATPase activity.Reference Kanoh55 Non-strial tissues of the lateral wall express β2-receptors but not β1-receptors.Reference Schimanski, Scofield and Wangemann56 In contrast, the semicircular canal duct secretes chloride under the control of β2-adrenergic receptors.Reference Milhaud, Pondugula, Lee, Herzog, Lehouelleur and Wangemann57 The potassium secretion is under the inhibitory control of M3 and M4 muscarinic receptors that are located in the basolateral plasma membrane, as well as β2-adrenergic receptors.Reference Wangemann, Liu, Scherer, Herzog, Shimozono and Scofield58, Reference Wangemann59 Transepithelial potassium and sodium transport is mediated by cyclic adenosine 3′,5′ monophosphate (c-AMP) as second messenger.Reference Sunose, Liu, Shen and Marcus60, Reference Tu, Chiu, Shu and Lien61 The stria vascularis shows the highest adenylate cyclase activity of the entire inner ear.Reference Koch and Zenner62, Reference Kumagami, Beitz, Wild, Zenner, Ruppersberg and Schultz63
Potassium secretion in the marginal cells of the stria vascularis is stimulated by the vasopressin derivates ADH (antidiuretic hormone), AVP (arginine vasopressin) or DDAVP (desmopressin trade name), involving V2-receptors and also c-AMP.Reference Sunose, Liu, Shen and Marcus60, Reference Lee, Kim and Kim64 Corticosteroids increase potassium secretion dose-dependently in the range of therapeutic plasma concentrations, and mineralocorticosteroids such as aldosterone show an opposite effect.Reference Lee and Marcus65 All serum- and glucocorticoid-inducible kinases one to three, and the protein B kinase, stimulate an voltage gated potassium channel KCNE1/KCNQ1,Reference Embark, Bohmer, Vallon, Luft and Lang66 and it has been shown that sodium absorption and osmotically coupled water flux is under the control of short-circuit current (Isc) stimulation via steroids regulating the Na+/K+–ATPase and potassium channels.Reference Pondugula, Sanneman, Wangemann, Milhaud and Marcus67
In addition, potassium secretion in the stria vascularis and the dark cells can be mediated via ATP and uridine triphosphate.Reference Liu, Kozakura and Marcus68 Sound exposure leads to ATP secretion into the endolymph from a vesicular store inside the stria vascularis, and this initiates protective mechanisms.Reference Munoz, Kendrick, Rassam and Thorne69 Adenosine triphosphate and uridine triphosphate inhibit potassium secretion via the apically located P2Y4 and the basolaterally expressed P2Y2 purinergic receptors,Reference Marcus, Sunose, Liu, Shen and Scofield70–Reference Marcus, Liu, Lee, Scherer, Scofield and Wangemann72 and activate reabsorptive pathways via P2X receptors.Reference Konishi, Yamane, Iguchi, Takayama, Nakagawa and Sunami11 The exclusively in the apical plasma membrane located P2Y4 receptor is similarly expressed to the staining of KCNE1 in the apical plasma membrane.Reference Sage and Marcus73 Adenosine triphosphate regulates potassium transduction, via the P2X receptors (ion channel) and the slower P2Y receptors (G-protein), and simultaneously reduces the efflux from the stria vascularis.Reference Housley, Jagger, Greenwood, Raybould, Salih and Jarlebark74
There is a strongly expressed and largely non-overlapping distribution pattern for different aquaporin subtypes in the inner ear, suggesting the existence of regional, subtype-specific water transport pathways.Reference Beitz, Kumagami, Krippeit-Drews, Ruppersberg and Schultz75–Reference Lowenheim and Hirt77 Global regulation of water transport in the inner ear may require the concerted actions of many different types of aquaporins.Reference Huang, Chen, Chen, Nagura, Lim and Lin78 Aquaporin one has been found to be expressed in the intermediate cells which abundantly express ion transporters, suggesting a role in water distribution associated with vigorous ion transport in the stria vascularis.Reference Sawada, Takeda, Kitano, Takeuchi, Kakigi and Azuma79 Aquaporin two is highly expressed in the endolymph surrounding tissues,Reference Mhatre, Jero, Chiappine, Bolasco, Barbara and Lalwani80 and, additionally, aquaporins five and seven have been found in the lateral wall and vestibular epithelia.Reference Sawada, Takeda, Kitano, Takeuchi, Kakigi and Azuma79, Reference Mhatre, Steinbach, Hribar, Hogue and Lalwani81 It has been shown that vasopressin application increases aquaporin two and V2-receptors along the cochlear duct,Reference Sawada, Takeda, Kitano, Takeuchi, Kakigi and Azuma79, Reference Kitano, Suzuki, Kitanishi, Yazawa, Kitajima and Isono82 and corticosteroids have been demonstrated to increase aquaporins one and three.Reference Fukushima, Kitahara, Fuse, Uno, Doi and Kubo83–Reference Kitahara, Fukushima, Uno, Mishiro and Kubo85
Atrial natriuretic peptide play a role in the central and peripheral control of body water and electrolytes, and are highly expressed in dark cells, intermediate cells and marginal cells, but less so in basal cells.Reference Chen, Wang, Liu and Qiu86, Reference Suzuki, Kitanishi, Kitano, Yazawa, Kitajima and Takeda87 It has been shown in rats that atrial natriuretic peptide reduces the endolymph volume of the inner ear.Reference Kumagami, Beitz, Wild, Zenner, Ruppersberg and Schultz63
Transport characteristics
There are five different compartments in the lateral wall of the inner ear, each with a distinct ionic composition. The ionic composition of the spiral ligament, which is similar to that of perilymph, represents the first compartment. The second compartment contains the basal cells and demonstrates an abrupt change in ion concentration. In contrast, the third compartment, which includes the extracellular space, possesses a low potassium concentration (in comparison with the second compartment). The fourth compartment, which includes the marginal cells, is similar to the fifth compartment and the scala media in terms of potassium and sodium concentration, but contains lower chloride levels.Reference Ikeda and Morizono26 Briefly, the lack of Na/K–ATPase in the basal cells suggests that their main role is to establish a barrier between the stria vascularis and the spiral ligament. The intermediate cells, with their extensive, active transport mechanisms, are responsible for generating the endolymphatic potential;Reference Prazma88 consequently, the marginal cells possess a positive intracellular potential similar to that of the scala media.
Potassium
Potassium constitutes the major charge carrier for sensory transduction. It is ideal for this role, since it is by far the most abundant ion in the cytosol. The importance of adequate potassium regulation is underlined by the fact that ampullar nerve discharge activity (excitatory post synaptic potential (EPSP) and propagated spikes) may be decreased or increased by elevated or reduced potassium levels, respectively,Reference Botta, Valli, Zucca and Casella89 and an increase in potassium over a longer time could damage the motility of the outer hair cells.Reference Zenner, Reuter, Zimmermann, Gitter, Fermin and LePage90 The main pathway for potassium in the apical plasma membrane is the IsK (short circuit channel), which is composed of the KCNE1/KCNQ1 (beta-subunit of the voltage dependent and outwardly conducting channel = IsK/minK/alpha-subunit of the slowly activating channel = KvLQT1.Reference Nicolas, Dememes, Martin, Kupershmidt and Barhanin91 Potassium secretion is correlated with the potassium content of the perilymph.Reference Wangemann, Shen and Liu92 The Isk channel is activated by acidification and inhibited by alkalinisation of the cytosol.Reference Wangemann, Liu, Shen, Shipley and Marcus93 There is evidence that its KCNQ1 subunit is tightly regulated by small changes in cell volume when coexpressed with aquaporin one. Activation by cell swelling and inhibition by cell shrinkage probably involves interaction between the cytoskeleton and the N-terminus of the channel proteins.Reference Grunnet, Jespersen, Macaulay, Jorgensen, Schmitt and Pongs94 Defects of either the KCNQ1 or KCNE1 subunit result in impairment of endolymph production and collapse of the endolymph spaces.Reference Nicolas, Dememes, Martin, Kupershmidt and Barhanin91, Reference Bleich and Warth95–Reference Vetter, Mann, Wangemann, Liu, McLaughlin and Lesage97 Mutations of these subunits are associated with the autosomal recessive, cardioauditory Jervell and Lange–Nielsen syndrome, characterised by profound sensorineural deafness and prolonged Q–T intervals of cardiac action potentials.Reference Neyroud, Tesson, Denjoy, Leibovici, Donger and Barhanin46, Reference Monnig, Schulze-Bahr, Wedekind, Eckardt, Kirchhof and Funke98, Reference Tyson, Tranebjaerg, McEntagart, Larsen, Christiansen and Whiteford99
Further potassium channels have been found in the secretory epithelia of the inner ear. KCNK6 (inward rectifying potassium channel) encodes a tandem pore domain potassium channel (TWIK2). This channel is located predominantly in the stria vascularis, and has been hypothesised to complement the effects of KCNQ1 and KCNE1 by fine-tuning the endolymph potassium concentration (the net effect being potassium efflux).Reference Mhatre, Li, Chen, Yost, Smith and Kindler100 Nonselective, calcium-activated cation channels and maxi K+ channels have also been found but are expressed at too low a density to maintain transepithelial transport.Reference Marcus and Shen101 Under stimulated conditions, these channels may make a limited contribution to potassium secretion and sodium absorption.Reference Marcus, Takeuchi and Wangemann102
Sodium and chloride
The largest conductance in the basolateral membrane of vestibular dark cells and marginal cells is chloride conduction responsible for chloride recirculation.Reference Wangemann3, Reference Wangemann and Marcus103 This is primarily represented by the type K chloride channel CLCKNA (ClC-K1),Reference Ando and Takeuchi104 and probably to a lesser extent by the chloride channels CLCN2 and CLCN3.Reference Oshima, Ikeda, Furukawa and Takasaka105 Impairment of both subunits of the ClC-K chloride channel (ClC-Ka and ClC-Kb; rodent orthologues are type K chloride channel CLCKNA and ClC-K2, respectively) in marginal and dark cells leads to decreased endolymph volume, labyrinth collapse and deafness. Mutations in the gene encoding the Barttin subunit affect both type K chloride channels, causing the sensorineural hearing loss observed in Bartter syndrome type IV.Reference Flagella, Clarke, Miller, Erway, Giannella and Andringa106–Reference Sage and Marcus108 Recently, mutations in the genes encoding ClC-Ka and ClC-Kb, detected in one patient, were observed to result in similar clinical symptoms to those seen in Bartter syndrome type IV.Reference Schlingmann, Konrad and Jeck109
The Na+–K+–Cl− cotransporter NKCC1 (SLC12A2) has been found in the basolateral membrane of marginal cells and vestibular dark cells, in spiral ligament fibrocytes, in the spiral limbus and in tissues underlying the neurosensory epithelium.Reference Crouch, Sakaguchi, Lytle and Schulte50 Marginal cells extrude sodium basolaterally and provide the driving force for the Na–K–2Cl cotransporter, resulting in additional flow of potassium into the cell. Co-localisation of Na+/K+–ATPase and Na–K–2Cl cotransporter has been found in cochlear and vestibular fibrocytes, underlining their role in potassium circulation and endolymph production.Reference Ikeda and Morizono26, Reference Crouch, Sakaguchi, Lytle and Schulte50 In contrast to the renal absorptive form, this isoform is secretory, and dysfunction leads to deafness, imbalance, reduced endolymph production and cellular collapse of the membranous labyrinth due to impaired potassium cycling.Reference Flagella, Clarke, Miller, Erway, Giannella and Andringa106, Reference Delpire, Lu, England, Dull and Thorne110 In dark cells, the Na+–K+–2Cl cotransporter has been shown to represent a solute uptake and efflux mechanism, and is thus responsible for volume control,Reference Wangemann and Marcus111 together with K+–Cl− transporter and Na+/K+–ATPase.Reference Wangemann and Shiga112 In the apical plasma membrane, the Na+–K+–2Cl– cotransporter is expressed together with amiloride-sensitive sodium channels, guaranteeing intake. In the basolateral membrane, these mechanisms probably contribute to the specific resting potential.Reference Ferrary, Bernard, Oudar, Sterkers and Amiel113–Reference Komune, Nakagawa, Hisashi, Kimituki and Uemura115 The alpha, beta and gamma subunits of the epithelial amiloride-sensitive sodium channel are extensively expressed in the inner ear.Reference Zhong and Liu116 Mutations in transmembrane serine protease (TMPRSS3), an activator of the epithelial sodium channel, have been observed in both familial and sporadic cases of autosomal recessive sensorineural deafness (i.e. DFNB8/10). Mutations in the subunits of epithelial amiloride-sensitive sodium channels have been found in patients with autosomal recessive pseudo-hypoaldosteronism.Reference Guipponi, Vuagniaux and Wattenhofer117
Na+-K+-adenosin triphosphatase
Adequate Na+-K+–ATPase is the basis for the high potassium concentration of endolymph and for the endocochlear potential, which are essential for sensory function of the inner ear. Expression of this enzyme in the stria vascularis (together with the morphology of the supporting cells) correlates with the endolymphatic potential decline observed towards the apex, reflecting the tonotopy of the cochlea.Reference Spicer, Smythe and Schulte22, Reference Kuijpers and Bonting118 In addition, expression of Na+/K+–ATPase is greater in the ampullae than in the utricle, and correlates with the extent of membrane infolding.Reference Pitovski and Kerr119 The Na+/K+–ATPase in the dark and marginal cells, consisting of ATPA1 and ATPB2 (and to a lesser extent ATPB1) subunits, confers a very low affinity for sodium and potassium compared with other subunit combinations. This perfectly assists the strial marginal cells in their maintenance of a very low potassium concentration in the intrastrial spaces of the marginal cells.Reference Blanco and Mercer120–Reference TenCate, Curtis and Rarey123 Consequently, it is efficient that ATP is stored in the stria vascularis and is secreted in response to metabolic stresses such as noise and hypoxia.Reference Munoz, Kendrick, Rassam and Thorne69 It has been found that the binding sites of aldosterone are similar to the localisation of Na+/K+–ATPase, with highest expression in the stria vascularis and the epithelial cells of the spiral prominence.Reference Shibata, Hibino, Doi, Suzuki, Hisa and Kurachi124 Mineralocorticoid receptor one has been found in the stria vascularis;Reference Furuta, Mori, Sato, Hoshikawa, Sakai and Iwakura125 however, it has been shown that Na+/K+–ATPase activation cannot be due to mineralocorticoid receptor one alone.Reference Erichsen, Berger, Schmid, Stierna and Hultcrantz126 In addition, it has been shown in animal models that the ATPA1 and ATPB1 isoforms of Na+/K+–ATPase in the stria vascularis, the spiral ganglion neuron, the cochlear nerve and the limbus spiralis are responsive to thyroid hormone.Reference Zuo and Rarey127 It has also been shown that the potassium-dependent phosphatase activity of the Na+/K+–ATPase complex is upregulated in the stria vascularis by adrenaline, noradrenaline and serotonin, whereas dopamine and reserpine decrease its activity.Reference Ikeda, Kusakari, Takasaka and Saito128
Other transport mechanisms
The main buffer in the endolymph appears to be HCO3–/CO2 and generated metabolically in stria vascularis, in particular in strial marginal cells. Proteins play only a minor role, due to their low concentration. The significance of pH homeostasis in cochlear fluids is linked to the general pH sensitivity of ion channels, transporters and metabolic enzymes. In animal models, it has been shown that acidification of cochlear fluids reduces the endocochlear potential and enhances free radical stress and hearing loss, whereas alkalinisation has a protective effect.Reference Sterkers, Saumon, TranBaHuy, Ferrary and Amiel129–Reference Misrahy, Hildreth, Clark and Shinabarger131
The pH of endolymph is not substantially different from that of bloodReference Misrahy, Hildreth, Clark and Shinabarger131 or perilymph,Reference Sterkers, Saumon, TranBaHuy, Ferrary and Amiel129 suggesting that H+ is being secreted into endolymph against an electrochemical gradient. Alpha and beta H+–K+–ATPase has been found in the intermediate cells of the stria vascularis, the spiral ligament, the organ of Corti and the spiral ganglion, and extrudes H+ in exchange for K+. A role in cochlear potassium circulation and thus in generation of the endolymphatic potential has been suggested, as inhibition leads to a prominent reduction in endolymphatic potential.Reference Sinha and Pitovski132, Reference TranBaHuy and Lecain133 In addition, the pH-regulating protein vH+–ATPase is expressed most strongly in the apical plasma membrane of marginal cells.Reference Stankovic, Brown, Alper and Adams134 Mutations of the H+–ATPase cause distal renal tubular acidosis and have been found in patients with mild and early or later onset hearing loss.Reference Stover, Borthwick and Bavalia135 The vestibular dark cells and the strial marginal cells contain the Na+–H+ exchanger NHE1 in the basolateral membrane, which probably functions to maintain intracellular pH levels; it has been shown that blockage of this exchanger leads to cell acidification and transient stimulation of potassium secretion.Reference Bond, Ng and Schulte136–Reference Wangemann, Liu and Shiga138
The Glut1-facilitated glucose transporter is ubiquitously expressed. It is localised in the basal cells and basolateral infoldings of the marginal cells and also in the capillary walls of both the stria vascularis and the dark cells.Reference Yoshihara, Satoh, Yamamura, Itoh and Ishii139 The importance of this transporter is underlined by the fact that the most metabolically active cells lack glycogen storage.Reference Ito, Spicer and Schulte140 The H+–monocarboxylate cotransporter facilitates cellular uptake of lactate, pyruvate and other monocarboxylates. Coexpression with Na+–K+–ATPase in marginal and dark cells has been found in gerbils, suggesting an important source of energy to drive inner-ear Na+/K+–ATPase activity.Reference Okamura, Spicer and Schulte141
Endolymphatic potential generation
The main driving force for sensory transduction is the endocochlear potential. Its maturation is correlated with the development of tight junctions in basal cells and the development of gap junctions in basal and intermediate cells of the stria vascularis.Reference Souter and Forge142 The endolymphatic potential is essentially a potassium equilibrium potential generated by the low potassium content of the intrastrial space and the high potassium content of the intermediate cells. In the lateral cochlear wall, the inwardly rectifying potassium Kir 4.1 channel (KCNJ10) is expressed in intermediate cells and the Kir 5.1 channel is expressed in types II, IV and V fibrocytes of the spiral ligament.Reference Hibino, Higashi-Shingai, Fujita, Iwai, Ishii and Kurachi143 The latter participates in potassium circulation, whereas intermediate cells create the endolymphatic potential with a contribution from the Kir 4.1 channel.Reference Takeuchi and Ando144 Strial marginal cells contribute to endocochlear potential generation, in that they maintain the potassium concentration of the intrastrial fluid spaces at an extremely low level.Reference Marcus, Rokugo and Thalmann145, Reference Takeuchi, Ando and Kakigi146 Similarly, spiral ligament fibrocytes assist the generation of the endolymphatic potential by maintaining a high cytosolic potassium concentration in the intermediate cells.Reference Crouch, Sakaguchi, Lytle and Schulte50, Reference Schulte and Adams51 Mice lacking the Kir 4.1 channel do not generate an endolymphatic potential, and endolymph volume and potassium concentration are reduced.Reference Marcus, Wu, Wangemann and Kofuji147 Endothelins one and three and the ETA (endothelin receptor A) receptor in intermediate cells and in marginal cells opposed to the intermediate cells may participate in endolymphatic potential regulation by mediating Na+–K+–ATPase.Reference Fujimara, Furukawa, Doi and Fujimoto148
The Pendred syndrome is (at 5 per cent) the most common non-syndromal hearing loss syndrome and is characterised by congenital deafness, an enlarged vestibular aqueduct and goitre. It is caused by mutations of SLC26A4, which codes for pendrin, an anion exchanger that appears to secrete HCO3– into the endolymph.Reference Everett, Glaser, Beck, Idol, Buchs and Heyman149 Pendrin is expressed in the apical plasma membrane of the stria vascularis, in the spiral prominence, in the outer sulcus cells, in the transitional cells of the vestibular labyrinth and in the apical membrane of endolymphatic sac cells.Reference Everett, Morsli, Wu and Green150–Reference Wangemann, Itza, Albrecht, Wu, Jabba and Maganti152 Pendrin-negative mice show a normal potassium concentration in the endolymph and the marginal cells but also an endolymphatic potential close to zero, a high endolymphatic calcium concentration and a thin stria vascularis. These alterations are probably caused by reduced expression of KCNJ10, which is involved in endolymphatic potential generation in the basal layer of the intermediate cells. Loss of pendrin increases the concentration of HCO3– and CO2 in the stria vascularis, which leads to compensatory upregulation of proteins involved in HCO3– generation and transport. The carbonic anhydrases CAR2, CAR3 and CAR14, the Na+ independent anion exchanger SLC4A3, the Na+-dependent HCO3– transporter SLC4A7, and the Ca2+-activated Cl– channel CLCA1 are upregulated in the stria vascularis in pendrin-negative mice. Elevated CO2 concentrations facilitate an accelerated rate of nitrate radical generation, leading to free radical stress, which may cause the loss of the KCNJ10 K+ channel and an increase in melanin production and hyperpigmentation. Melanin may serve as a scavenger for nitrate radicals. Loss of the KCNJ10 K+ channel leads to loss of endolymphatic potential, which in turn causes an increase in endolymphatic Ca2+ concentration, resulting in the death of sensory hair cells.Reference Wangemann, Jabba, Singh and David153 In addition to a decreased endolymphatic potential, endolymph volume and potassium content are decreased in null-mutants for the KCNJ10 channel, as this channel also provides a major pathway for potassium cycling.Reference Marcus, Wu, Wangemann and Kofuji147
Potassium circulation
According to the tonotopy of the cochlea, potassium concentration and circulation are generally stronger at the cochlear base, and they differ in the three scalae. In the auditory system, potassium circulation begins with entrance of potassium into the sensory cells via the apical transduction channel (Figure 2). The inner and outer hair cells possess different mechanisms of potassium recirculation. The outer hair cells deliver potassium via the basolateral channels into the perilymph, including the KCNQ4 channel. Potassium is absorbed by spiral ligament fibrocytes and enters the intermediate cells via connexins 26, 31 and 30 (Gene GJB 2, 3 and 6). Subsequently, potassium is secreted into the intrastrial space via KCNJ10, which generates the endolymphatic potential, and then enters the marginal cells via the Na+–K+–2Cl– cotransporter NKCC1 and the Na+–K+–ATPases α1 and β2. Potassium is secreted into the endolymph by KCNQ1/KCNE1 (Isk/KvLQT1). There is evidence for a medial potassium recycling pathway from the inner hair cells and also the inner radial nerves. The border cells, inner sulcus cells, limbal fibrocytes and interdental cells all participate in this pathway and return potassium to the endolymph.Reference Spicer and Schulte154, Reference Wangemann155 Along the proposed pathways, multiple types of aquaporins (one, three, four, five and seven) are expressed but do not generally overlap. These water pathways may provide a necessary water regulation mechanism in order to compensate the osmotic changes which result from potassium ion flow.Reference Huang, Chen, Chen, Nagura, Lim and Lin78

Fig. 2 Schematic representation of a cochlear turn with the most significant recycling pathways of K+ ions illustrated by arrows. Furthermore it depicits the organ of Corti composed of sensory inner (IHC) and outer (OHC) hair cells and supporting cells (ESC), internal sulcus cells (ISC), spiral limbus (Li), interdental cells (IDC), Reissner's membrane (RM), and stria vascularis (StV) adjacent to tue fibrous spiral ligament (SL) Reproduced with permissionReference Peters, Monnens-Cor, Cremers-Jo and Curfs36
Potassium circulation in the vestibular system and dark cells involves the same transporters and mechanisms as in the cochlea; however, there is no equivalent for the spiral ligament and intermediate cells.Reference Wangemann156 In the semicircular canal ampullae, potassium ions are initially released into the extracellular space during stimulation, and are subsequently absorbed by supporting and light cells. Finally, they are transported transcellularly over numerous very long gap junctions into the the dark cell area. From here, they move to an extracellular compartment, which is tightly sealed off basally by the basal plates of the light cells. Apically, the intercellular space between the light and dark cells is sealed by junctional complexes. This storage space corresponds to the extracellular compartment between the marginal and intermediate cells in the stria vascularis.Reference Helling and Merker157
The cycling of potassium in the cochlea is not limited to the pathway through the hair cells. Parts of the current generated by the stria vascularis appear to be carried through Reissner's membrane and the outer sulcus cells.Reference Chiba and Marcus158–Reference Zidanic and Brownell160 Similarly, some of the current generated by the vestibular dark cells appears to be carried through the vestibular transitional cells, which are similar to the outer sulcus cells.Reference Lee, Chiba and Marcus161
Calcium
Calcium plays a role in metabolism, cytoskeletal integrity, cell shape and cell excitability, amongst other functions. Calcium enters the hair bundle together with potassium. Endolymphatic calcium is present only in the nanomolar range but is essential for adequate hair cell relaxation, mechano-electrical transduction, current generation and transduction mechanism adaptation.Reference Holt and Corey162, Reference Ricci and Fettiplace163 Hearing loss in cases of vitamin D deficiency and hypoparathyroidism has been attributed to reduced endolymphatic calcium concentration.Reference Brookes164, Reference Ikeda, Kobayashi, Kusakari, Takasaka, Yumita and Furukawa165 Increased expression of the calcium-binding protein calmodulin can be found in the stria vascularisReference Yamashita and Bagger-Sjoback166 but not in the supporting and dark cells,Reference Ogata and Slepecky167 representing an important calcium reservoir. In contrast to endolymph, in which calcium is mostly undissociated and mainly bound to bicarbonate or phosphate, calcium in the perilymph is dissociated as it is ultrafiltrated from plasma. This, together with the electrochemical gradient over the endolymph–perilymph barrier, explains the necessity of active calcium transport. Calcium secretion into the endolymph is conducted by Ca2+–ATPase located in the apical and basolateral plasma membrane of basal and marginal cells and also in the basolateral plasma membrane of dark cells.Reference Ikeda and Morizono168, Reference Yoshihara, Igarashi, Usami and Kanda169 In mice, mutation of plasma membrane calcium–ATPase leads to ataxia and decreased endolymph calcium levels.Reference Wood, Muchinsky, Filoteo, Penniston and Tempel170 Calcium absorption may occur through paracellular and transcellular pathways, and is driven at least in part by the endolymphatic potential.Reference Housley, Jagger, Greenwood, Raybould, Salih and Jarlebark74 Transcellular pathways may include uptake from endolymph by Ca2+-permeable TRP (transient receptor potential) channels (ECaC) and export into the perilymph via Ca2+–ATPases and Na+–Ca2+ exchangers.Reference Wood, Muchinsky, Filoteo, Penniston and Tempel170 In addition, there is some evidence that voltage-dependent Ca2+ channels in the vestibular supporting and dark cells play a role in regulating calcium in the endolymph; intake is stimulated by a high K+ concentration.Reference Imon, Amano, Ishihara, Sasa and Yajin172, Reference Mori, Amano, Sasa and Yajin173 It has also been suggested that the calcium content of the endolymph could be related to the demineralisation of otoconia and the subsequent intake of mucopolysaccharides and mucoproteins in the otoconial matrix into the dark cells by active pinocytosis.Reference Harada and Takumida174
Conclusion
The morphology and function of the stria vascularis and the vestibular dark cells are the bases for inner-ear fluid homeostasis and adequate response to stimulation, respectively. Defects lead to altered volume, ion concentrations, osmolarity and pressure in the inner ear and are associated with numerous diseases. Further research into the distinctive functions and regulation of these structures is necessary in order to develop future clinical interventions.
Acknowledgements
I am indebted to Dr Theo Peters and Prof G van Camp, who contributed their excellent figures to this work.