1. Introduction
The Lower Rhine Basin is part of the European Cenozoic Basin (Fig. 1) which extends c.1100km from the Mediterranean to the North Sea (e.g. Zagwijn, Reference Zagwijn1989; Ziegler, Reference Ziegler1992; Sissingh, Reference Sissingh2003; Dèzes, Schmid & Ziegler, Reference Dèzes, Schmid and Ziegler2004). The European Cenozoic Basin formed as a result of passive rifting in late Eocene times, related to the reactivation of late Variscan, Permo-Carboniferous and Mesozoic fracture systems (Dèzes, Schmid & Ziegler, Reference Dèzes, Schmid and Ziegler2004). The 100km long and 50km wide (Klett, Eichhorst & Schäfer, Reference Klett, Eichhorst, Schäfer, Schäfer and Siehl2002), triangular-shaped Lower Rhine Basin extends from the Netherlands in the NW to Bonn in the SE and cuts into the NW margin of the Rhenish Massif (Fig. 1).

Figure 1. A structural map of the European Cenozoic Rift System. At its northern end the Upper Rhine Graben bifurcates into the Hessen and Leine grabens to the NE, and the Lower Rhine Basin in the NW (Rhenish Triple Junction in the area of Frankfurt; after Schumacher, Reference Schumacher2002; Sissingh, Reference Sissingh2003; Rasser et al. Reference Rasser, Harzhauser, Anistratenko, Anistratenko, Bassi, Belak, Berger, Bianchini, Čičić, Ćosović, Doláková, Drobne, Filipescu, Gürs, Hladilová, Hrvatovć, Jelen, Kasiński, Kováč, Kralj, Marjanac, Márton, Mietto, Moro, Nagymarosy, Nebelsick, Nehyba, Ogorelec, Oszczypko, Pavelić, Piwocki, Poljak, Pugliese, Redžepović, Rifelj, Roetzel, Skaberne, Sliva, Standke, Tunis, Vass, Wagreich, Wesselingh and McCann2008).
The German part of the Lower Rhine Basin contains c. 1300m of Oligocene- to Pleistocene-age siliciclastic sediments with associated lignite seams (Schäfer et al. Reference Schäfer, Hilger, Gross and Von der Hocht1996; Klett, Eichhorst & Schäfer, Reference Klett, Eichhorst, Schäfer, Schäfer and Siehl2002). The depositional succession records a transgressive–regressive trend beginning with marine deposits overlying Palaeozoic- and Mesozoic-age basement (Klett, Eichhorst & Schäfer, Reference Klett, Eichhorst, Schäfer, Schäfer and Siehl2002) in Rupelian times, through to a continental–marine environment from Chattian to Messinian times (late Oligocene to late Miocene) and a final phase of continental sedimentation from Zanclean times (early Pliocene) onward (Sissingh, Reference Sissingh2003; Schäfer & Utescher, Reference Schäfer and Utescher2014).
During Miocene times regression of the North Sea and warm and temperate climatic conditions (Utescher, Mosbrugger & Ashraf, Reference Utescher, Mosbrugger and Ashraf2000; Zachos et al. Reference Zachos, Pagani, Sloan, Thomas and Billups2001; Utescher et al. Reference Utescher, Mosbrugger, Ivanov and Dilcher2009) in combination with ongoing subsidence (Hager, Reference Hager1993; Schäfer et al. Reference Schäfer, Hilger, Gross and Von der Hocht1996) favoured the accumulation of up to 270m of peat deposits in the centre of the basin (Hager, Kothen & Spann, Reference Hager, Kothen, Spann, Reiche and Hilden1981; Hager, Reference Hager1986; Schäfer et al. Reference Schäfer, Hilger, Gross and Von der Hocht1996). These thick peat accumulations formed the Main Seam of the Ville Formation (present-day thickness: up to 100m; Figs 2, 3). In the NW part of the Lower Rhine Basin the Main Seam is subdivided by two transgressive marine sand units (Frimmersdorf and Neurath sands) into the Morken, Frimmersdorf and Garzweiler seams (Figs 2, 3).

Figure 2. Stratigraphic log of the Lower Rhine Basin (after Klett, Eichhorst & Schäfer, Reference Klett, Eichhorst, Schäfer, Schäfer and Siehl2002; Schäfer, Utescher & Mörs, Reference Schäfer, Utescher and Mörs2004; Schäfer et al. Reference Schäfer, Utescher, Klett and Valdivia-Manchego2005; Schäfer & Utescher, Reference Schäfer and Utescher2014). The lithostratigraphy is a synthesis based on the SNQ 1 (Erft Block) and Efferen (Köln Block) wells (RWE Power AG), and taken to represent the stratigraphy at the centre of the Erft Block (lithostratigrapical code established by Schneider & Thiele, Reference Schneider and Thiele1965). Biostratigraphical ages (Ma) on the left after Berggren et al. (Reference Berggren, Kent, Swisher, Aubry, Berggren, Kent, Aubry and Handenbol1995), cycle ages (Ma) after Hardenbol et al. (Reference Hardenbol, Thierry, Farley, Jacquin, De Graciansky, Vail, de Graciansky, Hardenbol, Jacquin and Vail1998).
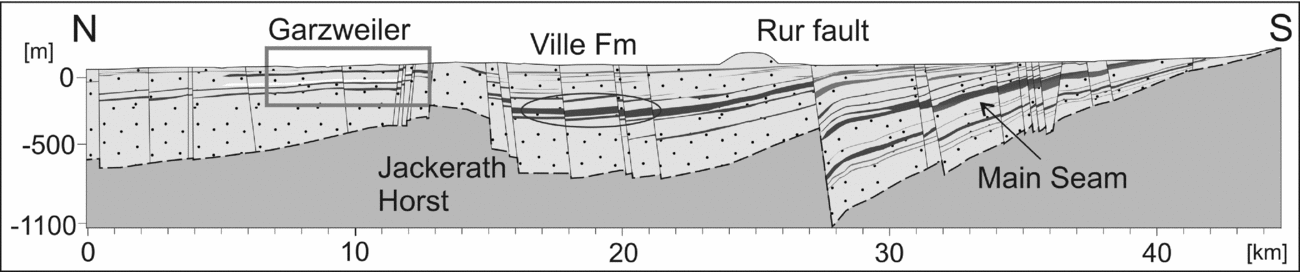
Figure 3. N–S-oriented cross-section of the Lower Rhine Basin (N–S line in structural map, Fig. 4a), cross-cutting the Jackerath Horst, the Garzweiler open-cast mine (rectangle) and a series of normal and reverse faults. The Main Seam is present in the SE, subdividing into the Morken, Frimmersdorf and Garzweiler seams in the NW (Ville Formation indicated by oval; stratigraphy based on RWE data).
The Lower Rhine Basin became the subject of increased scientific and economic interest in the 20th century, resulting in a wide range of stratigraphic, sedimentological, palaeoclimatic and palaeontological studies (M. Teichmüller, Reference Teichmüller and Ahrens1958a ; R. Teichmüller, Reference Teichmüller and Ahrens1958 b, Reference Teichmüller, Illies and Fuchs1974; Utescher, Ashraf & Mosbrugger Reference Utescher, Ashraf, Mosbrugger and Kovar-Eder1992; Hager, Reference Hager1993; Schäfer et al. Reference Schäfer, Hilger, Gross and Von der Hocht1996; Klett, Eichhorst & Schäfer, Reference Klett, Eichhorst, Schäfer, Schäfer and Siehl2002; Becker & Asmus Reference Becker and Asmus2005; Schäfer et al. Reference Schäfer, Utescher, Klett and Valdivia-Manchego2005; Schäfer & Utescher, Reference Schäfer and Utescher2014). The thick lignite accumulations in the Lower Rhine Basin have been exploited since the 19th century, when industrial open-cast mining commenced. Today, there are three active open-cast mines run by RWE Power AG in the region, namely Hambach, Inden and Garzweiler (Fig. 4). The Garzweiler open-cast mine (this study), NW of Cologne, was developed in 1983 as the NW extension of the older Frechen, Bergheim, Fortuna-Garsdorf and Frimmersdorf open-cast mines (RWE Power AG). In the Garzweiler II open-cast mine, the seams of the Ville Formation with an initial lignite amount of 1.2 billion tons are currently mined, mainly for electricity generation (RWE Power AG, internal reports).

Figure 4. Structural maps of the Lower Rhine Basin. (a) The NW part of the Lower Rhine Basin comprises the Peel, Venlo and Krefeld blocks in the N and the Rur, Erft and Köln blocks in the S (after Schäfer & Utescher, Reference Schäfer and Utescher2014; RWE Power AG); the blocks are each separated by major fault systems; section N–S: see Figure 3. (b) Distribution of lignite within the Lower Rhine Basin (white, dotted: Morken Seam; light grey, hatched: Frimmersdorf Seam; dark grey, hatched: Garzweiler Seam; based on RWE data). JH = Jackerath Horst; G = Garzweiler open-cast mine; H = Hambach open-cast mine; I = Inden open-cast mine.
The Langhian/Serravallian-age Frimmersdorf Seam in the Garzweiler open-cast mine contains sand bodies, which are broadly distributed throughout the Garzweiler mining area, and which have been recognized for over 30 years (RWE Power AG internal report). Their irregular distribution as well as their extremely variable nature has detrimentally affected both the mining and processing of the lignite. Indeed, the increase in occurrence of sand bodies within the seam, as well as their extent, has since 2008 complicated mining in the area, so that up to 1.5 million tons of lignite per year has had to be discarded during this period (RWE Power AG, internal report). Currently the economic loss is significantly reduced as a result of additional processing in coal preparation plants. The situation would be greatly eased by early recognition of the entire sand volume in any future mining areas. However, this is difficult, largely because most sand bodies are restricted in their thickness (centimetres to metres). The distances between the exploration wells and the resolution (sand bodies have to be at least 10cm thick) of geophysical logs (e.g. gamma ray, density, resistivity) impede the precise location of sand bodies and, therefore, the medium- to short-term disposal of lignite volumes which, as noted above, is an important aspect for future planning and mining.
The origin and emplacement of these sand bodies has, to date, not been examined. This study is the first to provide a detailed classification of the highly variable sand bodies, with the aim of reconstructing their origin and emplacement mechanisms as well as providing a temporal framework for these processes.
2. Geological setting
The Lower Rhine Basin is located at the NW end of the European Cenozoic Rift System, which extends c.1100km from the Mediterranean via the Upper Rhine Graben to the North Sea Basin (Ziegler, Reference Ziegler1992; Sissingh, Reference Sissingh2003) (Fig. 1). Initiation and Cenozoic-age evolution of this extensive rift system appear to be related to the Alpine Orogeny, i.e. the collision of the anticlockwise-rotating African with the European plates (Schumacher, Reference Schumacher2002; Sissingh, Reference Sissingh2003; Dèzes, Schmid & Ziegler, Reference Dèzes, Schmid and Ziegler2004; Ziegler & Dèzes, Reference Ziegler and Dèzes2007; Rasmussen, Dybkjaer & Piasecki, Reference Rasmussen, Dybkjaer and Piasecki2010).
Rifting within the European Cenozoic Rift System was initiated in middle to late Eocene times, and induced by intra-plate, compressive tectonics (Ziegler, Cloetingh & van Wees, Reference Ziegler, Cloetingh and van Wees1995; Dèzes, Schmid & Ziegler, Reference Dèzes, Schmid and Ziegler2004; Ziegler & Dèzes, Reference Ziegler and Dèzes2007). The rift system consists of two main parts, the Massif Central – Rhône Valley rift systems in the S and the Rhine Rift System in the N, both of which comprise numerous, subordinate, graben systems (Schumacher, Reference Schumacher2002; Sissingh, Reference Sissingh2003; Dèzes, Schmid & Ziegler, Reference Dèzes, Schmid and Ziegler2004; Ziegler & Dèzes, Reference Ziegler and Dèzes2007). The initiation of the subsidiary rift systems within the European Cenozoic Rift System resulted from compressional and regional tensional stresses in the Alpine Foreland (Ziegler, Reference Ziegler1992). Rifting was associated with intra-plate magmatism and volcanism (Ziegler, Cloetingh & van Wees, Reference Ziegler, Cloetingh and van Wees1995; Dèzes, Schmid & Ziegler, Reference Dèzes, Schmid and Ziegler2004; Ziegler & Dèzes, Reference Ziegler and Dèzes2007).
Within the Rhine Rift System, the N–S-trending Upper Rhine Graben bifurcates into the Hessen–Leine Depression in the NE and the Lower Rhine Basin in the NW (Prodehl et al. Reference Prodehl, Mueller, Glahn, Gutscher and Haak1992; Ziegler, Reference Ziegler1992; Sissingh, Reference Sissingh2003). These three subordinate graben systems form the Rhenish Triple Junction (Sissingh, Reference Sissingh2003), located at the SE end of the Rhenish Massif (Fig. 1). The Rhenish Triple Junction was the central point of volcanic activity during Cenozoic times (Ziegler, Reference Ziegler1992).
Subsidence in the Lower Rhine Basin commenced in Oligocene times (Schäfer et al. Reference Schäfer, Hilger, Gross and Von der Hocht1996; Klett, Eichhorst & Schäfer, Reference Klett, Eichhorst, Schäfer, Schäfer and Siehl2002) at the NW margin of the Rhenish Massif, initiated at reactivated SW–NE-trending Variscan thrusts that influenced rifting from Rupelian times onwards (Nickel, Reference Nickel2003; Sissingh, Reference Sissingh2003). Subsidence continued along several NW–SE-trending fault systems (Schäfer et al. Reference Schäfer, Hilger, Gross and Von der Hocht1996), including the Rur, Erft, Peel and Viersen faults, together with their numerous subsidiary faults (Figs 3, 4). These fault systems subdivide the Lower Rhine Basin into a series of grabens and horsts, comprising six main blocks. These include the Rur, Erft and Köln blocks to the SE of the Lower Rhine Basin, and the Peel, Venlo and Krefeld blocks to the NW (Figs 3, 4). The six main blocks subsided at different rates, for example, the Erft Block to a maximum of 1300m, while the Peel Block subsided to a depth of 2000m (Klett, Eichhorst & Schäfer, Reference Klett, Eichhorst, Schäfer, Schäfer and Siehl2002).
The study area, within the Garzweiler open-cast mine, is surrounded by several tectonic features, some of which are still active (Ziegler, Reference Ziegler1992; Hinzen, Reference Hinzen2003; Sissingh, Reference Sissingh2003; Ewald et al. Reference Ewald, Igel, Hinzen and Scherbaum2006). Of these, the most important (in terms of this study) are the Erft Fault System (which separates the Erft and Köln blocks) and the Jackerath Horst at the southern margin of the Garzweiler open-cast mine (Figs 3, 4). The Erft Fault System comprises a series of broadly NW–SE-trending normal faults with an average dip of 70–80° to the SW (Schäfer, Utescher & Mörs, Reference Schäfer, Utescher and Mörs2004). However, in the area S of the Garzweiler open-cast mine the strike direction of the faults within the Erft Fault System changes from the usual NW–SE orientation to broadly WNW-ESE and W-E trends, with a subsidiary ENE-WSW orientation, as well as the formation of a small horst structure (Fig. 4a). The WNW–ESE-striking step faults of this horst, termed the Jackerath Horst, form the S boundary of the present-day open-cast mine and were initially active in early Rupelian times. Therefore, the Jackerath Horst can be considered to be one of the oldest Cenozoic-age tectonic elements in the Lower Rhine Basin (Nickel, Reference Nickel2003).
The Cenozoic evolution of the Lower Rhine Basin was influenced by major climatic changes (Utescher, Mosbrugger & Ashraf, Reference Utescher, Mosbrugger and Ashraf2000; Zachos et al. Reference Zachos, Pagani, Sloan, Thomas and Billups2001; Utescher et al. Reference Utescher, Mosbrugger, Ivanov and Dilcher2009), characterized by a cooling trend from mean annual temperatures of 23–25°C in Oligocene and Miocene times down to 14°C in late Pliocene times (Utescher, Mosbrugger & Ashraf, Reference Utescher, Mosbrugger and Ashraf2000; Mosbrugger, Utescher & Dilcher, Reference Mosbrugger, Utescher and Dilcher2005). The warmest Cenozoic-age climatic conditions in the Lower Rhine Basin have been observed in middle/late Eocene to early Oligocene times, with temperature peaks at the Late Oligocene Warming (late Chattian-age) and the Mid-Miocene Climatic Optimum (late Burdigalian-age; Zachos et al. Reference Zachos, Pagani, Sloan, Thomas and Billups2001; Utescher et al. Reference Utescher, Mosbrugger, Ivanov and Dilcher2009; Grein et al. Reference Grein, Oehm, Konrad, Utescher, Kunzmann and Roth-Nebelsick2013). These temperature peaks are associated with sea-level highstands in the Lower Rhine Basin (Schäfer & Utescher, Reference Schäfer and Utescher2014). Warm and temperate (at least subtropical) climatic conditions became increasingly cold and temperate in late Miocene times (Utescher, Mosbrugger & Ashraf, Reference Utescher, Mosbrugger and Ashraf2000; Zachos et al. Reference Zachos, Pagani, Sloan, Thomas and Billups2001; Utescher et al. Reference Utescher, Mosbrugger, Ivanov and Dilcher2009). In Pliocene times a further marked temperature decrease with mean annual temperatures of 14°C has been observed (Utescher, Mosbrugger & Ashraf, Reference Utescher, Mosbrugger and Ashraf2000; Mosbrugger, Utescher & Dilcher, Reference Mosbrugger, Utescher and Dilcher2005), heralding the Pleistocene glaciation (Mosbrugger, Utescher & Dilcher, Reference Mosbrugger, Utescher and Dilcher2005).
Sedimentation in the Lower Rhine Basin was influenced by constantly changing depositional environments which were controlled by subsidence, sea-level changes and river channel avulsion (Becker & Asmus, Reference Becker and Asmus2005). The ingression of the North Sea onto the Palaeozoic and Mesozoic basement in early Oligocene times marked the beginning of marine sedimentation in the North Sea region (Schäfer & Utescher, Reference Schäfer and Utescher2014). In the centre of the Lower Rhine Basin, sub- to supratidal sediments were deposited (Petzelberger, Reference Petzelberger1994; Schäfer et al. Reference Schäfer, Hilger, Gross and Von der Hocht1996) in a high-energy estuarine embayment with a meso-tidal range (Schäfer et al. Reference Schäfer, Hilger, Gross and Von der Hocht1996; Schäfer & Utescher, Reference Schäfer and Utescher2014). Sediment input at this time was mainly clastic, derived from fluvial systems located to the S (Boersma et al. Reference Boersma, van Gelder, de Groot, Puigdefabregas, Reiche and Hilden1981; Schäfer & Utescher Reference Schäfer and Utescher2014), draining the Rhenish Massif (Hager, Reference Hager1993). It has also been suggested that a secondary clastic component was derived from coastal currents transporting material from the NW (Hager, Reference Hager1993) as well as redistributing previously deposited fluvial sediment.
At the Oligocene–Miocene transition, a decrease in the rate of basin subsidence resulted in partial regression of the North Sea (Becker & Asmus, Reference Becker and Asmus2005), and a northwesterly shift in the shoreline. Subsequently, the fluvial system became more extensive, traversing the newly exposed coastal plain. Subsidence was also accompanied by the onset of peat formation (Schäfer, Utescher & Mörs, Reference Schäfer, Utescher and Mörs2004; Becker & Asmus, Reference Becker and Asmus2005). Repeated marine transgressions resulted in the deposition of basin succession comprising alternating beds of clays, silts, peat and marine sediments (Schäfer, Utescher & Mörs, Reference Schäfer, Utescher and Mörs2004; Becker & Asmus, Reference Becker and Asmus2005), the so-called Köln Formation (Lower Seam Group; Aquitanian – early Burdigalian).
From early Burdigalian to late Serravallian times, coastal swamps with associated peat bogs and marshes became widespread (Teichmüller, Reference Teichmüller and Ahrens1958a , Reference Teichmüller and Ahrens1958b ; Mosbrugger et al. Reference Mosbrugger, Gee, Belz and Ashraf1994; Schäfer et al. Reference Schäfer, Hilger, Gross and Von der Hocht1996; Klett, Eichhorst & Schäfer, Reference Klett, Eichhorst, Schäfer, Schäfer and Siehl2002). The thick peat accumulations from this period were the result of optimal climatic conditions, reduced subsidence and a decrease in sediment input from the Rhenish Massif. Up to 270m of peat were accumulated over a period of 9Ma (Hager, Kothen & Spann, Reference Hager, Kothen, Spann, Reiche and Hilden1981; Hager, Reference Hager1993), and subsequently compacted to form a lignite seam (up to 100m) in the centre of the Lower Rhine Basin (Hager, Kothen & Spann, Reference Hager, Kothen, Spann, Reiche and Hilden1981; Hager, Reference Hager1986; Mosbrugger et al. Reference Mosbrugger, Gee, Belz and Ashraf1994; Mosbrugger, Utescher & Dilcher, Reference Mosbrugger, Utescher and Dilcher2005). This Main Seam (Burdigalian–Serravallian) extends from the SE of the Lower Rhine Basin up to a NE–SW-trending boundary in the area of the Hambach and Inden open-cast mines (Fig. 4). Northwest of this line, two NW–SE-directed transgressions resulted in the deposition of thick marine sand intercalations within the Main Seam of the Ville Formation (Petzelberger, Reference Petzelberger1994), resulting in its subdivision into three lignite units (Figs 3, 4).
Stratigraphically the lowest lignite is the Morken Seam (c.10–12m; average seam thicknesses within the Garzweiler open-cast mine; derived from internal RWE Power AG reports) and this is overlain by the marine Frimmersdorf Sand (c.20m). Subsequent regression resulted in a period of peat growth, forming the present-day Frimmersdorf Seam (c.12m). The boundary of the Frimmersdorf Sand with the overlying Frimmersdorf Seam is commonly planar or slightly wavy and may be marked by the presence of well-developed root horizons. These roots are up to 0.5cm in diameter and extend down (up to 1m) into the Frimmersdorf Sand. The Frimmersdorf Seam is overlain by the marine Neurath Sand (15–40m), which accumulated during a period of transition from sub- to supratidal conditions. The uppermost Garzweiler Seam (Serravallian) is the youngest part of the Ville Formation. At the contact of the Garzweiler Seam and the underlying Neurath Sand, root horizons are rarely developed.
The Ville Formation was erosively capped by the sediments of the Inden Formation at the end of Serravallian times (Fig. 2). These were mainly deposited in meandering fluvial systems (Abraham, Reference Abraham1994; Klett, Eichhorst & Schäfer, Reference Klett, Eichhorst, Schäfer, Schäfer and Siehl2002; Schäfer, Utescher & Mörs, Reference Schäfer, Utescher and Mörs2004). The Inden Formation also contains thin lignite seams, the so-called Upper Seam Group (Figs 2, 4), which accumulated in locally restricted swamp environments (Schäfer, Utescher & Mörs, Reference Schäfer, Utescher and Mörs2004) over a period of 2Ma (Hager, Reference Hager1993). The alternation of peats and fluvial deposits in the Inden Formation marks the final retreat of the North Sea from the Lower Rhine Basin (Boersma et al. Reference Boersma, van Gelder, de Groot, Puigdefabregas, Reiche and Hilden1981; Abraham, Reference Abraham1994; Valdivia-Manchego, Reference Valdivia-Manchego1994; Schäfer & Utescher, Reference Schäfer and Utescher2014).
At the late Miocene/Pliocene transition, sand and gravel as well as thick clay beds were deposited in lacustrine and fluvial environments on top of the Inden Formation (Schäfer & Utescher Reference Schäfer and Utescher2014). Fluvial deposition changed from meandering to braided river systems during Pliocene times (Schäfer & Utescher, Reference Schäfer and Utescher2014). These clastic Messinian- to Piacenzian-age sediments are termed the Hauptkies, Rotton and Reuver formations (Fig. 2). In Pleistocene times, the drastic climatic changes were marked by the deposition of thick fluvial units (terrace sediments; Schäfer & Utescher Reference Schäfer and Utescher2014), accumulated in braided systems (precursors of the Rhine, Meuse, Mosel, Sieg and Wupper rivers) (Boenik, Reference Boenik, Reiche and Hilden1981; Klett, Eichhorst & Schäfer, Reference Klett, Eichhorst, Schäfer, Schäfer and Siehl2002).
3. Classification of sand bodies in the Frimmersdorf Seam
Sand bodies within the Frimmersdorf Seam exhibit strong variations related to their appearance, as well as their frequency or position within the seam. Sand bodies in fresh outcrops commonly vary from black to grey, or brown to reddish brown in colour. In older cut faces (days to one to two weeks old), the weathered sand bodies bleach to light grey, beige or white. Many sand bodies show evidence of vertical or horizontal bleaching. Colour changes and bleaching occur independently of any variations in grain size or internal sedimentary structures. Classification of the sand bodies is based on six criteria: (1) position, (2) morphology, (3) orientation, (4) composition, (5) grain size and (6) sedimentary structures.
3.a. Position
The frequency and position of the sand bodies within the Frimmersdorf Seam show strong lateral and vertical variations. Sand bodies may be either isolated or grouped (up to 10). They can be (1) concentrated at the seam base, (2) concentrated at the top of the seam, (3) distributed throughout the seam or, indeed, (4) absent. Sand bodies occurring at the seam base can be isolated or concentrated. These latter occurrences consist of a variety of sand bodies, both laterally and vertically extensive.
Isolated sand bodies in the lower 50cm of the seam are often connected to the underlying Frimmersdorf Sand. More extensive sand bodies are distributed in the lower 2m of the seam, and these may also be connected to the underlying sand. The boundary between the Frimmersdorf Seam and the Frimmersdorf Sand is commonly marked by well-established root horizons. However, roots are absent in areas with sand bodies at the base of the seam, connected to the Frimmersdorf Sand.
Sand bodies at the top of the seam are generally isolated and may be connected to the overlying Neurath Sand. These sand bodies may also contain rare chert nodules. Similar cherts are commonly found in the Garzweiler open-cast mine, occurring as an extended bed in the lower metres of the overlying Neurath Sand.
Most sand bodies are distributed throughout the Frimmersdorf Seam without any apparent concentration at a certain level and lacking visible connections to both the Frimmersdorf and Neurath sands. However, the vertical, as well as lateral, extent of the sand bodies can change over only a few metres, so that barren (≥ 17% sand, up to 30% in total) parts of the Frimmersdorf Seam and sand-free units may be juxtaposed over short distances.
3.b. Morphology
The sand bodies show a high degree of variation in terms of morphology (Fig. 5). This is further complicated by the fact that the various morphologies may be connected with one another, thus leading to composite forms. Four main morphologies have, to date, been recognized: (1) layers/beds, (2) channel-like bodies, (3) irregular bodies and (4) reticulate or net-like bodies formed from combinations of 1–3. These shapes do not take into account vertical/subvertical bodies with a dyke-like character (these will be examined below, in Section 3.c). All recognized shapes can occur within a single vertical profile.

Figure 5. Sand bodies within the Frimmersdorf Seam illustrating the different morphologies present: (a) concordant sand beds, with even upper and wavy lower boundaries; (b) thick (up to 1.8m) channel-like sand body (c) irregular-shaped sand body; and (d) reticulate sand bodies.
The horizontal layers/beds (1; Fig. 5a) are very variable in terms of thickness, ranging from 2mm up to 1.0m. Thicknesses are also laterally variable over distances of centimetres to tens of metres. The individual layers may thin and taper laterally, dying out over metres to tens of metres, or they may end abruptly, and for no apparent reason, with a sharp cut-off. Individual layers may show constant thicknesses laterally, or they may be lenticular, with lateral thinning and thickening. Bifurcation may also occur within individual layers, with one bed splitting into two or more separate beds. Indeed, these beds may laterally rejoin to form one continuous bed again, thus resulting in a series of lignite pods separated by horizontal layers of continuous sand. The boundaries of these sand layers vis-à-vis the lignite are usually sharp. However, the upper and lower boundaries of the sand layers are variable, the former being wavy while the latter show a more pronounced wavy or irregular character.
Lenticular or channel-like sand bodies (2) (Fig. 5b) are much thicker than the layers and beds, ranging from 50 to 200cm. They are never present as isolated sand bodies, but are always connected to horizontal (i.e. sills) or vertical (i.e. dykes) sand bodies. The boundaries with the surrounding lignite are commonly sharp, although they resemble the sand layers (1, above) in that the lower boundaries are often wavy while the upper boundaries are less wavy. The lignite in both the sand beds/layers and the channel-like bodies is generally more compact and undisturbed than in areas with sand bodies of other shapes (see 4, reticulate sand bodies).
Irregular sand bodies (3; Fig. 5c), where present, generally have angular to roughly circular to ovoid forms, and range in diameter from c.10 to c.60cm. The contacts with the lignite are sharp. Irregular sand bodies never occur in isolation but always in combination with one of the other four recognized shapes, as well as with sand sills and/or dykes (Fig. 5c).
Reticulate sand bodies (4; Fig. 5d) are widespread, often with a poorly-defined net-like shape. However, given that they are often combined with other morphologies (1–3 above), as well as sand sills and dykes, they can be highly irregular (Fig. 5c). These sand bodies can extend laterally over 3–4m, and have a general thickness of 50–150cm. The individual veins of the net structure range in thickness from millimetres to centimetres. Of particular note is that the lignite within these reticulate structures is less compacted than that adjacent to, or within, the other morphologies (1–3). Additionally, the isolated lignite fragments vary in shape from roughly rectangular to roughly ovoid.
3.c. Orientation
Although there is a degree of overlap between the morphological characteristics used above, the clear directional aspect of the sand bodies discussed within this category has led us to consider them as a descriptive feature in their own right. Three main bodies can be recognized: concordant sand bodies (beds/layers (1), morphology above), broadly horizontal, sill-like bodies and broadly vertical, dyke-like bodies (Fig. 6a). Detailed examination of the existing photographic material (RWE Power AG Archive) has revealed that sills are much more common than dykes (2:1 ratio). Both of these bodies will be discussed in more detail below.

Figure 6. Sand bodies illustrating the orientation: (a) combination of sills and dykes; (b) vertical dyke between sills; (c) sills with vertical offset of 1–2cm; and, (d) sills with vertical offsets of up to 10cm.
Sill-like sand bodies may be straight or slightly wavy and extend over distances of tens of metres. The sill thickness varies between 1 and 50cm (although the majority range between 1 and 20cm). Unlike the bedded/layered morphology (1) above, the sills are not concordant with bedding, but are instead slightly discordant (with angles of up to c.15°). Sills may be isolated or can occur in ‘swarms’ with a series of up to 10 parallel or subparallel sill bodies. Additionally, individual sills may form convex-upward bodies within the lignite. The various sills are often linked with dykes, particularly where there are a series of parallel or subparallel sills present (Fig. 6b).
Sills may show evidence of normal faulting (Fig 6c, d). Offsets of 1–20cm have been observed, and the faults may extend through a group of adjacent sills (over a distance of c.2m).
Sand dykes are vertical or subvertical (16–90°) bodies of sand which extend upwards within the lignite linking other sand bodies together, although isolated dykes (extremely rare) may also occur. The dykes vary in thickness from 5mm up to 20cm and can be traced over a scale of metres across the outcrop. As mentioned above, sills and dykes of varying inclinations may commonly occur in combination with one another.
Dykes may extend into the Frimmersdorf Seam from the underlying Frimmersdorf Sand (these also comprise the majority of the observed dykes which are connected to the surrounding strata), or downwards from the overlying Neurath Sand (these dykes are extremely rare). Dykes intruding from the Frimmersdorf Sand into the Frimmersdorf Seam often merge into sills, and these latter may bifurcate and/or thin laterally. One particular dyke extending c.2m downwards from the Neurath Sand had a listric/curved form and thinned laterally. (Interestingly, this dyke also contained pebbles of chert in its upper part, i.e. that part which was closest to the Neurath Sand.)
The orientations of both sills (n = 29) and dykes (n = 61) were measured within the Frimmersdorf Seam. The orientations of a range of concordant sand bodies (i.e. 1 above) were also measured (n = 16). Additionally, bedding orientations of both the lignite and the sand bodies bracketing it were measured, as well as a range of fractures within the lignite itself (Fig. 7). The predominant fracture orientations measured within the lignite were NW–SE, NE–SW and less commonly E–W. These three directions are also present in the sand bodies, although the NE–SW direction is more common (Fig. 8).

Figure 7. Stereographic projection of lignite surfaces, (a) symbol ’+’ illustrating fault planes, ‘x’ reflecting bedding surfaces; (b) density diagram.

Figure 8. Stereographic projection of sand surfaces, (a) symbol ‘+’ illustrating sills and dykes, ‘x’ reflecting bedding surfaces; (b) density diagram.
3.d. Composition
Petrographically, the sand bodies within the Frimmersdorf Seam predominantly consist of quartz (mainly monocrystalline) and K-feldspar, subordinate plagioclase and rarer amphibole, pyroxene, muscovite and zircon, in decreasing order of abundance. The white- or light grey-coloured sand grains are surrounded by dark-coloured humic substances which give the sand bodies a dark red, brown or even black colour.
Two clast types, namely lignite (as well as wood fragments) and mud, are common within the sand bodies (Fig. 9a, b, c). Such clasts are only present in sand bodies that are > 10cm thick. Lignite clasts (1; Fig. 9a, b) are the most frequent clasts present and range in shape from angular to well rounded, with diameters of 1–20cm, although the majority are sub-angular to sub-rounded and <20cm in diameter. Lignite clasts are generally isolated and irregularly distributed throughout the sand bodies, although they also tend to be concentrated in the central parts of the sand bodies, rather than along the boundary areas with the surrounding lignite.
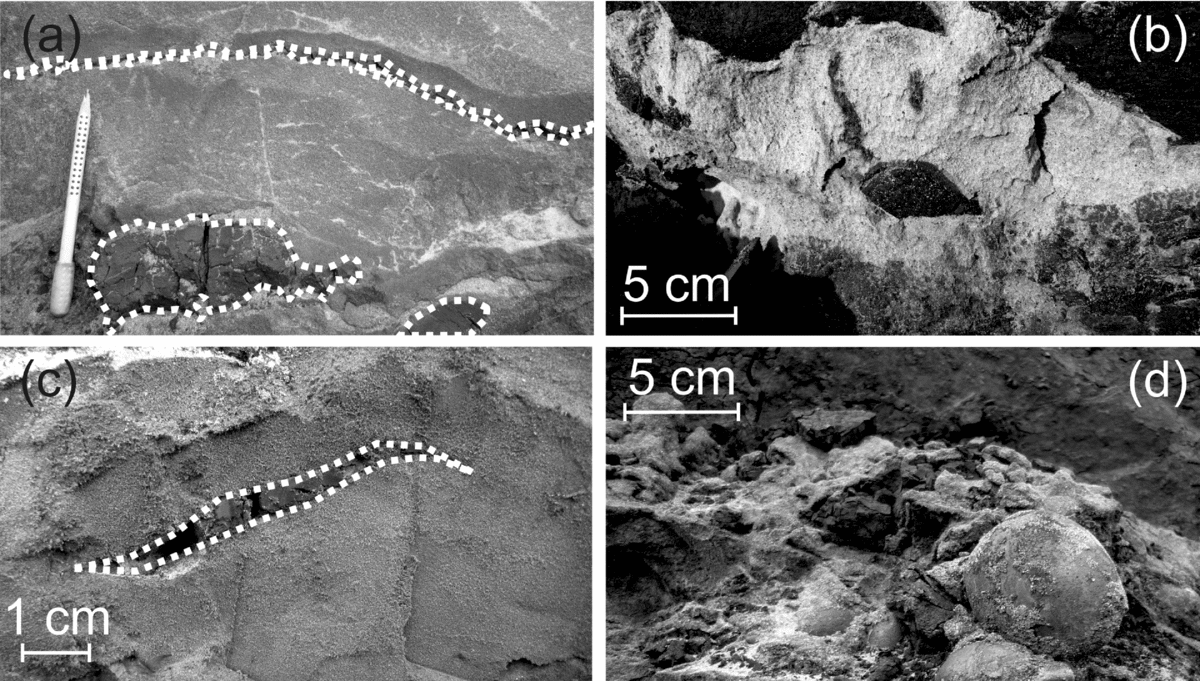
Figure 9. Composition of the sand bodies: (a) lignite lens at the top, lignite clasts at the bottom of a sand body; (b) sub-rounded lignite clasts; (c) mud lens; and (d) chert pebbles.
Thin sigmoidal-shaped lenses of lignite (Fig. 9a) were noted in three different sand bodies. These lenses are 1–3cm thick and extend over a distance of up to 20cm. The individual lenses are oriented broadly perpendicular to bedding. These occur in sand bodies which also contain sub-rounded lignite clasts (up to 10cm long; Fig. 9a).
Within one of the largest of the observed sand bodies (10m wide and up to 1m thick) there was a lens of concentrated lignite clasts close to the top of the sand, and parallel with the sand–lignite contact. Individual clasts were sub-angular to rounded. In addition, two large wood fragments (up to 20cm in diameter and 30cm long) were also present. The smaller of the wood fragments (c.10cm diameter) was located adjacent to the lignite-clast lens, whereas the larger fragment occurred at the upper boundary of the sand body and the lignite.
Mud in the form of lenses (2) and beds (3) is also present within the sand bodies. The lenses, dark grey or black, are generally 1–2cm thick and are often sigmoidal deformed (Fig. 9c). Thicker mud beds may also occur within individual sand bodies. In such cases, individual mud beds may be up to 10cm thick, with sharp boundaries with the surrounding sand. These mud beds may occur at the top or bottom of horizontal sand bodies.
At seven different locations within the Garzweiler open-cast mine, sand bodies containing chert pebbles were noted (Fig. 9d). As noted above, isolated chert pebbles occurred rarely in sand dykes; these dykes migrated downwards from the overlying Neurath Sand. In addition, lenses or clusters of chert pebbles (up to 50cm thick) may be concentrated either at the bottom, the centre or the top of concordant sand bodies and sills. Isolated chert pebbles may also occur within the lignite. However, thus far they have only been noted in lignites which are in close proximity to sand bodies also containing chert clasts.
3.e. Grain size and sorting
The sands within the Frimmersdorf Seam are commonly fine- to medium-grained, and medium to well sorted, as well as being remarkably uniform (silt+clay <10%, coarse-grained sand <5%). Individual grains are sub-angular to rounded. Both the underlying Frimmersdorf Sand and the overlying Neurath Sand were also examined, indicating that the grain-size distribution of the sampled sand bodies was most similar to that of the upper part of the Frimmersdorf Sand, although there was enough overlap between the sand bodies within the lignite and the two bounding sand units to suggest that the sand across the region was similar in terms of grain size. In contrast to the sands, the chert clasts range in size from pebble through to small cobbles and are generally sub-rounded to well rounded.
3.f. Sedimentary structures
Sedimentary structures were observed within some of the sand bodies, including concordant sand bodies, sills and channel-like sand units (up to 2m thick). The sedimentary structures can be classified as cross-lamination (1) and trough cross-lamination (2).
Cross-lamination (1) has been noted in concordant sand bodies and sills with the individual laminae highlighted by colour changes related to different proportions of humic substances within the sand. The sand units are up to 50cm thick and extend laterally for tens of metres.
Trough cross-lamination (2) is present in concordant sand bodies, sills and channel-like bodies, where the grain sizes of the individual laminae range from fine- to coarse-grained sand (although the sand bodies themselves are not graded). Lamination is not always visible throughout the entire sand body.
Thin-section analyses of samples from cross-laminated sills have revealed that lamination is related to grain-size variations (90–450µm) and grain packing (Fig. 10a). Humic substances fill the interstitial space between individual mineral grains (Fig. 10b), and these substances are enriched in the coarser-grained laminae (230–430µm). Additionally, the alignment of elongate particles of organic matter (up to 3mm in length) also accentuates the lamination (Fig. 10a).

Figure 10. Petrographic view of sand from a sill-like sand body. The sorting of the quartz grains results from a variation of grain sizes and grain packing (a and b); the pore space is filled by dark humic substances (a). Note the presence of elongated organic particles (a) oriented parallel to lamination (b).
In two locations, planar (cf. Section 3.g. below) and trough cross-lamination are present within thin, alternating sand–lignite beds. The trough cross-lamination was observed in the basal part of a thick (up to 3m thick) sand body.
3.g. Sand body complexity
The various features noted above may occur together, resulting in the generation of sand bodies of extraordinary complexity. One particular example comprises a sand body (20–40cm thick and laterally extensive over c.5m) underlain by laminated lignite (i.e. part of the Frimmersdorf Seam). The boundary of the sand and lignite is sharp, but when traced laterally in two dimensions, the sand and lignite interdigitate with one another, at least at the base of the contact between them (Fig. 11). In the upper third of the sand body, there is well-developed trough cross-lamination (thickness 2cm) which passes laterally into a cluster of chert clasts. Individual lamina surfaces may contain small, isolated pebbles of lignite. The chert clasts within the cluster range in size from 0.5 to 3.0cm, while the entire cluster is c.5cm thick and laterally extensive over at least 10cm. A summary of the various sand body types, classified on the basis of the above-noted criteria, is shown in Figure 12.
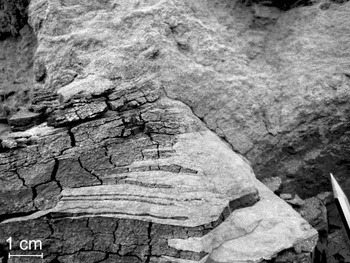
Figure 11. Thick sand body underlain by laminated lignite. The sand body also contains a variety of chert pebbles and lignite clasts.

Figure 12. Classification of sand bodies: (1) concordant layers/beds (tabular); (2) channel-like sand bodies; (3) sill-like sand bodies (tabular), (a) isolated or several parallel sills, (b) sill-like sand bodies with cross-lamination; (4) dyke-like sand bodies, (a) isolated dykes on top of the Frimmersdorf Seam with connection to the overlying Neurath Sand, (b) isolated or parallel dykes within the seam, without connection to the Neurath or Frimmersdorf sands; (5) combination of sills and dykes, (a) dykes intruding into the Frimmersdorf Seam from the underlying Frimmersdorf Sand, (b) symmetric sills and dykes within the seam, (c) dykes connected to tabular sand bodies; (6) sand bodies with irregular morphology; (7) reticulate sand bodies.
3.h. Frimmersdorf Seam and Frimmersdorf Sand Boundary
The Frimmersdorf Seam is bounded by two sand units. The lowermost boundary (i.e. with the Frimmersdorf Sand) is widely exposed within the Garzweiler open-cast mine, while the uppermost boundary with the Neurath Sand is rarely accessible (being covered by mine tailings or exposed at locations where it is difficult to access). This latter boundary, however, has been interpreted as broadly erosional. The nature of the lower boundary, in contrast, is unclear and of particular interest in terms of the development of the sand bodies within the lignite unit.
As noted above, the lower boundary of the Frimmersdorf Seam is commonly marked by extensive root horizons, extending down into the Frimmersdorf Sand. However, the morphology of this lower boundary would appear to be affected by the presence and quantity of sand bodies within the lower 6m of the seam. Well-defined root horizons are common in areas with no or few sand bodies in the overlying seam, whereas in areas with extensive sand bodies the boundary would appear to be more diffuse. Four criteria have been used in this study to describe the three boundary variations observed. These are (1) density of roots, (2) the presence of sedimentary structures, within the upper metre of the Frimmersdorf Sand, as well as (3) the quantity and (4) the types of sand bodies occurring in the overlying seam. The different boundary types are laterally restricted and may change over a few metres.
The first and most frequent boundary type between the lignite and sand is commonly sharp and slightly uneven. This boundary type appears to be restricted to areas of sand-free lignite or rare isolated, mainly concordant or sill-like sand bodies in the overlying seam. It is also marked by up to 1m long roots. The roots are embedded in white-coloured and planar-laminated fine-grained sands.
The second boundary type is characterized by increasing amounts of sand bodies in the lignite, and the boundary between the lignite and sand becomes increasingly undulatory. Additionally, the amount, length and diameter of the roots present in the upper part of the Frimmersdorf Sand decreases. In this boundary type, the sand immediately below the lignite often contains larger wood fragments (trunks or branches), up to 20cm in diameter. The sands are partly laminated and often dark-coloured.
The boundary type forming beneath barren (i.e. relatively sand-rich) lignite, containing sills and dykes, irregular and reticulate sand bodies, changes significantly. The boundary is extremely undulatory, with height differences of up to 1m between the wave peaks and troughs. Roots or wood fragments as well as sedimentary structures are all absent.
4. Discussion
A variety of different sand bodies occurs within the Frimmersdorf Seam in the Garzweiler open-cast mine. These sand bodies have been described according to a number of different criteria mainly based on their morphologies and compositions (cf. Table 1). This study provides a classification of the highly variable sand bodies within the Frimmersdorf Seam and suggests a variety of possible emplacement mechanisms, which may act in isolation or conjunction, resulting in the complexity of the present-day sand body pattern.
Table 1. List of the criteria used for the classification of the sand bodies, their description and interpretation

4.a. Syn-depositional features
A number of features within the sand bodies have been used to suggest a syn-depositional origin. In particular, we refer to the presence of sedimentary structures or depositional/erosional morphologies which are best interpreted in terms of depositional processes. Thus, evidence for a syn-depositional origin of the sand bodies is based on (a) the internal structure within some of the sand beds/units, indicative of the action of wave and/or current activity, and (b) the concordance of the sand beds/units within the lignite seam suggesting stratigraphic continuity and thus linking the processes governing deposition.
As noted above, channel-like sand bodies are present within the lignite. These channels (with depths of up to 200cm) are clearly erosional, cutting down into the lignite. As such they were formed subsequent to peat formation, albeit not necessarily of the entire seam. Indeed, the presence of these channels would suggest that peat formation occurred in stages, each stage marked by periods of increased sand deposition across the swamp area. Thus the lignite body (i.e. Frimmersdorf Seam) can be best categorized as an amalgamated unit, formed over a period of time, but with significant (days, months, years?) periods when fluvial systems prograded across the partially indurated organic layers.
The presence of trough cross-lamination within the channel-like sand bodies within the Frimmersdorf Seam would suggest deposition from unidirectional currents within an aqueous setting. Such lamination, mainly located at the bases of the channel-like units, forms due to the migration of fluvial ripples or dunes located at the channel bottom. The fact that these bodies contain evidence of unidirectional current activity would militate against their being small ponds within the swamp landscape, since such ponds would not have ripples within them (lacking currents, ripples could only be generated by wind energy, which in such cases is too weak to form ripples). However, the fact that the trough cross-bedding is partly formed by alternating lignite and sand laminae may be interpreted as resulting from variations in the current velocity within the system.
The channel-like bodies also contain clasts of lignite, wood and mud. Such intraclasts may be typical in fluvial systems (e.g. Collier, Reference Collier2002). Indeed, Feldman, McCrimmon & De Freitas (Reference Feldman, McCrimmon, De Freitas, Hampson, Steel, Burgess and Dalrymple2008) have reported common concentrations of mud clasts (i.e. intraclast conglomerates), suggesting that they result from bank collapse. The relatively isolated nature of the mud clasts in the channel-like sand bodies, however, would preclude such an origin. The isolated mud clasts would suggest that they may be derived from local mud lenses within the lignite, rather than bank collapse, or have been transported into the channels from elsewhere within the system. Indeed, such wholescale bank collapse would be less likely within an environment where the banks are formed from relatively compacted organic matter rather than less compacted sediment. In such situations, lignite intraclasts would be more prevalent, which is precisely the situation observed here, where both isolated mud clasts and lignite intraclast concentrations were noted.
Both lignite intraclasts as well as wood fragments (including branches and trunks, up to 30cm in diameter and up to 1-1.5m long, although they are frequently smaller) are often aligned concordant to bedding, suggesting that they were derived as a result of erosion of the swamp vegetation and rapidly buried. Some larger fragments, however, show an orientation parallel to the current direction suggesting a degree of transport prior to final burial.
Although channel-like sand bodies are a useful indicator for the reconstruction of deposition of the sand bodies in a fluvial setting, thinner sand beds with lateral extents of tens of metres are much more frequent within the Frimmersdorf Seam. Tabular sand bodies with planar lamination, cross- and trough cross-lamination indicate that their deposition was controlled by currents. Such planar bodies are common in floodplain environments, which to date have been the subject of relatively less detailed study (see Miall, Reference Miall1996). Both Ethridge, Jackson & Youngberg (Reference Ethridge, Jackson, Youngberg, Ethridge and Flores1981) and Gersib & McCabe (Reference Gersib, McCabe, Ethridge and Flores1981) have subdivided floodplain areas within coal-bearing swamps into a number of different sub-environments, including crevasse splays and levees. Meanwhile, Wing (Reference Wing1984) noted that the presence of tabular bodies, similar to those recorded here, was related to the gradual aggradation of swamps (presumably with the incorporation of lignite intraclasts, as noted here), with the channel-like sand bodies representing active channel fill. Indeed, the overall fine-grained nature of the tabular and channel-fill sand bodies would suggest that the fluvial system which developed within the swampland of the present-day Frimmersdorf Seam was a low-gradient, meandering channel and floodplain system (cf. Madon, Abu Bakar & Ismail, Reference Madon, Abu Bakar and Ismail2010). Flooding of the low-lying areas across the swamp was controlled either by rising levels within the channel systems (most probably seasonal), or as a result of tidal/marine incursions from the adjacent North Sea. Such latter incursions, however, would be marked by the presence of mud drapes (in the case of tidal incursions; cf. Hovikoski et al. Reference Hovikoski, Räsänen, Gingras, Roddaz, Brusset, Hermoza, Romero Pittman and Lertola2005), or fossils in the case of more extensive marine incursions. Mud drapes were, however, not noted within the tabular sand bodies, while any fossils which may have been present within the sediments have been long since dissolved by the humic acids derived from the lignite units. Thus, it cannot be determined with absolute certainty whether the tabular sand bodies are the result of purely fluvial activity or whether a degree of marine influence occurred.
As noted above, chert pebbles may be present within both the channel-like and tabular sand bodies. These pebbles are concordantly arranged, parallel to bedding, and form flat-pebble conglomerates ranging in thickness from one clast thick through to 20cm thick. The origin of these chert pebbles, however, is problematic. Deposits within the Lower Rhine Basin containing chert pebbles are, to date, only known from marine strata. Indeed, a significant and widespread chert-pebble-rich marker bed is well known from the marine Neurath Sand (stratigraphically overlying the Frimmersdorf Seam), and interpreted by Utescher et al. (Reference Utescher, Ashraf, Dreist, Dybkjaer, Mosbrugger, Pross and Wilde2012) as a transgressive surface, deposited within a possible shoreface environment (Petzelberger, Reference Petzelberger1994), although such beds could also be interpreted as basal channel lags (cf. Andsbjerg et al. Reference Andsbjerg, Nielsen, Johannessen, Dybkjaer, Martinsen and Dreyer2001). Therefore, the pebble origin is of significance in determining the precise depositional relevance of these deposits. Chert-pebble beds have also been identified in the Morken Sands, a marine sand unit between the Morken I and Morken II seams and which is stratigraphically lower than the Frimmersdorf and Neurath sands (Petzelberger, Reference Petzelberger1994). Therefore, it is possible that the chert pebbles may have been eroded from older beds and redeposited as channel lags in the Frimmersdorf swamp setting. Provenance studies, however, would suggest the chert pebbles were eroded from the Aachen–Limburg carbonate plateau and distributed within the Lower Rhine Basin by marine currents (Albers & Felder, Reference Albers, Felder, Reiche and Hilden1981). If this was the case, their presence within sand bodies in the Frimmersdorf Seam may be indicative of the extensive influence of tides and tidal currents within the swamp area, given that these pebbles would need to be transported parallel to the palaeocoastline and carried upstream within the estuarine setting. In such a situation, the tidal limit (i.e. the maximum upstream tidal influence; Dalrymple, Zaitlin & Boyd, Reference Dalrymple, Zaitlin and Boyd1992) could have been located up to c.25km landward from the coastline (i.e the coastline in Miocene times, based on estimates in Utescher, Ashraf & Mosbrugger, Reference Utescher, Ashraf, Mosbrugger and Kovar-Eder1992). Certainly, tidal influence may reach hundreds of kilometres upstream, depending on tidal range and coastal morphology (cf. Dalrymple & Choi, Reference Dalrymple and Choi2007; Gong & Shen, Reference Gong and Shen2009). If, however, these chert pebbles were indeed transported by tidal currents along the coastline and upstream within the estuarine setting, it must also be presumed that the transporting currents carried significant amounts of sand-sized sediment in a bedload/suspension mix. This would then suggest that the channel-fill and tabular sands could be tidal deposits. However, there is a lack of any features suggestive of tidal influence (e.g. herringbone cross-stratification, paired mud/silt drapes; for details see Shanley et al. Reference Shanley, McCabe and Hettinger1992; Hovikoski et al. Reference Hovikoski, Räsänen, Gingras, Roddaz, Brusset, Hermoza, Romero Pittman and Lertola2005). Another possible explanation is that these deposits may have been transported onshore by storm surges. These high-energy events would have transported coarse-grained material upstream. Storm abatement would have been followed by the re-establishment of the fluvial regime, and the subsequent seawards transport of sediment, with the conglomerates representing a form of storm residue.
Tidal range and the effect on the depositional setting within the swamp area can increase significantly during spring or storm tides. Major storm events, supported by high temperatures of a subtropical climate (Zagwijn & Hager, Reference Zagwijn and Hager1987; Utescher, Mosbrugger & Ashraf, Reference Utescher, Mosbrugger and Ashraf2000; Mosbrugger, Utescher & Dilcher, Reference Mosbrugger, Utescher and Dilcher2005), may, as noted above, have resulted in widespread flooding of the swamps. Further evidence for the occurrence of flooding caused by storm surges is the presence of so-called groden bedding (i.e. alternating beds of sand and concentrated organic debris; cf. Schäfer, Utescher & Mörs, Reference Schäfer, Utescher and Mörs2004; Bungenstock & Schäfer, Reference Bungenstock and Schäfer2009; Schäfer, Reference Schäfer2010, p. 248) at the top of the Frimmersdorf Sand (Fig. 13), and the alternating sand–lignite succession in the overlying seam. Groden bedding develops as a result of flooding of salt marshes during storm tides, and may be identified from the juxtaposition of sands above halophyte vegetation (cf. Schäfer, Utescher & Mörs, Reference Schäfer, Utescher and Mörs2004; Bungenstock & Schäfer, Reference Bungenstock and Schäfer2009; Schäfer, Reference Schäfer2010, p. 248). In the study area, the high content of organic material within the bedding indicates the initial settlement of beach plants on top of the marine Frimmersdorf Sand following regression of the North Sea. In the lignite overlying these groden beds, a unit of alternating thick, concordant sand beds and lignite was developed. These layers may be indicative of repeated local flooding of the swamps.

Figure 13. Groden bedding, formed as a result of storm-controlled flooding of the swamp area, in the upper metres of the Frimmersdorf Sand. Note the presence of the root horizon at the sand–lignite boundary.
Sandstone dykes, extending downwards from the Neurath Sand into the Frimmersdorf Seam or from sand bodies (e.g. tabular sands and channel fills), have also been noted. The position of these dykes would suggest that they formed either due to dessication or as a result of tectonic activity. The fact that in the region groundwater levels were constantly rising, and that the organic-rich swamp (which ultimately formed the lignite seam) was more or less constantly under water, would militate against a dessication crack origin. The measured orientation of some of these dykes would appear to broadly conform to the regional tectonic trend, but the measured sample is too small to be definitive. However, what is clear is that some of these cracks were passively filled from above, most probably during periods of overbank flow or storm surges (e.g. those dykes extending downwards beneath tabular sands). The dykes at the top of the seam, and extending downwards from the Neurath Sand, were filled during the period of marine transgression and erosion which marked the cessation of swamp conditions (cf. Jolly & Lonergan Reference Jolly and Lonergan2002). This supposition is supported by the presence of cherts within one of the observed dykes; cherts, as noted above, are common in shoreface deposits at the base of the overlying Neurath Sand (Petzelberger, Reference Petzelberger1994).
The concurrence of fluvial, tidal and marine processes that may have formed the sand bodies within the Frimmersdorf Seam indicates a complex depositional environment controlled by river systems and the North Sea. As noted above, the Lower Rhine Basin in Mid-Miocene times formed an estuarine embayment (Schäfer et al. Reference Schäfer, Hilger, Gross and Von der Hocht1996; Schäfer & Utescher, Reference Schäfer and Utescher2014). Estuarine depositional settings are characterized by the interaction of fluvial and marine processes (Dalrymple, Zaitlin & Boyd, Reference Dalrymple, Zaitlin and Boyd1992; Hori et al. Reference Hori, Saito, Zhao, Cheng, Wang, Sato and Li2001). Based on the dominant controlling processes, estuarine embayments comprise three facies zones: the inner reach, the mixed-energy central part and the outer reach (Dalrymple, Zaitlin & Boyd, Reference Dalrymple, Zaitlin and Boyd1992). The inner reach is dominated by fluvial processes and extends to the landward limit of tidal influence. In the central zone of an estuary, the interaction of tides and river currents results in a mixed-energy facies environment. The influence of rivers decreases seawards, so that the outer reach is dominated by marine processes (Dalrymple, Zaitlin & Boyd, Reference Dalrymple, Zaitlin and Boyd1992).
Based on the dominant marine processes, estuaries can be further distinguished. Wave-dominated estuaries (e.g. the Eastern Shore estuaries, Nova Scotia, Canada; Boyd & Honig, Reference Boyd and Honig1992) are bordered by barrier sands separating the mixed-energy central zone from the purely marine shoreface. In tide-dominated estuarine environments (e.g. the South Alligator River, Australia; Woodroffe, Reference Woodroffe2000) the marine-dominated reach is characterized by tidal sand bars, whereas the mixed-energy zone is marked by tidal–fluvial channels (Dalrymple, Zaitlin & Boyd, Reference Dalrymple, Zaitlin and Boyd1992). The tidal influence may reach hundreds of kilometres landwards, depending on the tidal range and the coastal gradient (Dalrymple & Choi, Reference Dalrymple and Choi2007). Additionally, the tidal energy increases landwards due to the funnel-shaped geometry of estuaries (Dalrymple, Zaitlin & Boyd, Reference Dalrymple, Zaitlin and Boyd1992). This may result in tidal bores moving upstream (e.g. the up to 2m high wave in the Severn Estuary, SW UK; Uncles, Reference Uncles2010).
Sedimentary processes in an estuarine environment are regulated by periodic cycles, for example, tides and seasonal variations on the one hand, and spontaneous and aperiodic events (river flooding and storm surges) on the other hand (Gong & Shen, Reference Gong and Shen2009). Within the Lower Rhine Basin, fluvial influence from at least one river in the S (Hager, Reference Hager1993) interacted with meso- to macrotidal tidal ranges and minor swells of the North Sea (Schäfer et al. Reference Schäfer, Hilger, Gross and Von der Hocht1996). In Miocene times, the precursor of the present-day Sieg River entered the Lower Rhine Basin from the Rhenish Massif and crossed the central part of the swamp environment (Hager, Reference Hager1993). Minor fluvial channels within the Main Seam have been interpreted as river networks dewatering the swamp area (Petzelberger, Reference Petzelberger1994). In the SE area of the Lower Rhine Basin, an extended fluvial unit within the Frimmersdorf Seam has been identified (Schäfer et al. Reference Schäfer, Hilger, Gross and Von der Hocht1996; Schäfer & Utescher, Reference Schäfer and Utescher2014). Sand and clay were deposited in a highly sinuous meandering river, which eroded parts of the peat (Schäfer et al. Reference Schäfer, Hilger, Gross and Von der Hocht1996). The sinuous course of the river may be an indicator that deposition was located in the meandering zone of a tide-dominated estuary (Dalrymple & Choi, Reference Dalrymple and Choi2007). This hypothesis is supported by the presence of a thick sand body (up to 10m) within the uppermost Garzweiler Seam (Garzweiler open-cast mine), that comprised tidal bundles (Prinz et al., in press).
According to Dalrymple, Zaitlin & Boyd (Reference Dalrymple, Zaitlin and Boyd1992) and Dalrymple & Choi (Reference Dalrymple and Choi2007), estuarine environments only form under transgressive conditions. The lignite deposits of the Ville Formation are interpreted as base level rise half-cycles (Schäfer & Utescher, Reference Schäfer and Utescher2014). Additionally, the fluvial unit within the Frimmersdorf Seam (noted above) was associated with the Mid-Miocene Unconformity (MMU; Schäfer & Utescher, Reference Schäfer and Utescher2014), a marked transgressive surface that extends across the Lower Rhine Basin and the adjacent North Sea Basin (cf. Huuse, Lykke-Andersen & Michelsen, Reference Huuse, Lykke-Andersen and Michelsen2001; Thöle et al. Reference Thöle, Gaedicke, Kuhlmann and Reinhardt2014). An eustatic origin for this basin-wide transgression has been suggested (Schäfer & Utescher, Reference Schäfer and Utescher2014), although Thöle et al. (Reference Thöle, Gaedicke, Kuhlmann and Reinhardt2014) suggest a combination of climatic change and increased basin subsidence. In the German North Sea Basin, the transgressive surface of the MMU is overlain by deltaic sediments of the Eridanos Delta (Thöle et al. Reference Thöle, Gaedicke, Kuhlmann and Reinhardt2014). The onset of delta progradation was due to a significant increase in sediment input (Michelsen et al. Reference Michelsen, Thomsen, Danielsen, Heilmann-Clausen, Jordt, Laursen, Graciansky, Hardenbol, Jacquin and Vail1998), and a change in the sediment input direction from NE to E (Thöle et al. Reference Thöle, Gaedicke, Kuhlmann and Reinhardt2014). This increase in sediment supply may also have been responsible for the relatively large amount of sand bodies within the Frimmersdorf Seam, since the Morken and the Garzweiler seams only contain sporadic sand bodies.
4.b. Post-depositional emplacement mechanisms
The presence of various features (e.g. the discordance or faulting of sand bodies) has led us to consider post-depositional causal mechanisms, which may have been responsible for the emplacement and deformation of the sand bodies. In a broad sense, differential compaction across the region most probably resulted in thickness (and other?) variations occurring within both the sand and lignite units. Indeed, studies on the Main Seam (Hager, Kothen & Spann, Reference Hager, Kothen, Spann, Reiche and Hilden1981) have shown, for example, that the gentle folding of the lignite may have resulted from the settlement characteristics of the peat. Hager, Kothen & Spann (Reference Hager, Kothen, Spann, Reiche and Hilden1981) have suggested that the original thickness of the peat in the basin centre was c.270m, and that this was reduced over time and as a result of compaction, to a thickness of 100m. In contrast, the sand units both above and below the Main Seam are considered to be close to their depositional thicknesses (with only minor changes in density and porosity). The broad but gentle folding of the sedimentary units, however, does not explain the origin of the smaller features within the lignite bed, which is the subject of the present study, although it most probably resulted in additional post-depositional deformation/movement of some of the features.
The Lower Rhine Basin region in Pleistocene times was influenced by both glacial and periglacial activity, with relics of moraines, ice wedges or thick loess deposits being recorded (e.g. Thome, Reference Thome, Teichmüller and van der Brelie1959). These are generally associated with the Drenthe stage of the Saale glaciation (e.g. Golte & Heine, Reference Golte, Heine and Aymans1980). Indeed, permafrost conditions (associated with this or other glacial episodes) may have been responsible for the formation of fractures within the peat/lignite, that later could have been filled by fluidized sands. In Late Pleistocene times, climatic conditions favoured the formation of continuous permafrost in the area of the Rhine catchment (van Balen, Busschers & Tucker, Reference van Balen, Busschers and Tucker2010). However, ice wedges resulting from the seasonal thawing and freeze of the periglacial soils are, as far as known, in the Lower Rhine Basin restricted to the Pleistocene-age fluvial deposits (Thome, Reference Thome, Teichmüller and van der Brelie1959). Moreover, any characteristics (e.g. wedge-parallel lamination) of pseudomorphic ice wedge replacements are absent within the sand bodies of the Frimmersdorf Seam.
The presence of large ice bodies across the region of the Lower Rhine Basin meant that it was subjected to glacio-isostatic rebound subsequent to the retreat of the Weichselian-age Scandinavian and British ice sheets (e.g. Steffen, Kaufmann & Wu, Reference Steffen, Kaufmann and Wu2006; van Balen, Busschers & Tucker, Reference van Balen, Busschers and Tucker2010). Therefore, an isostatic response (5–10m of relative uplift along the Rhine; van Balen, Busschers & Tucker, Reference van Balen, Busschers and Tucker2010) would most probably have influenced the depositional setting. On the other hand, the general subsidence of the basin reached a maximum in Quaternary times (e.g. Grützner, Fischer & Reicherter, Reference Grützner, Fischer and Reicherter2016), at a time when rebound was occurring. Given that the degree of uplift (c.5–10m) occurred over a time span of 115ka, the precise amount of uplift which occurred during the period of basin subsidence would have been of the order of submillimetres, and probably not enough to influence the development of fractures within the lignite (which, as noted above, tend to be of the order of centimetres).
The evidence for post-depositional mechanisms resulting in the formation of some of the sand bodies includes (a) the discordance of the sand bodies (dykes, some sill-like sand bodies, irregular and net-like sand bodies) with regard to bedding, reflecting stratigraphic discontinuity, (b) the preferred orientation of some sand bodies reflecting the regional stress field (Figs 7, 8) and (c) the connection of some sand bodies either to the overlying Neurath Sand (see above) or the underlying Frimmersdorf Sand.
Sand bodies directly linked to the underlying Frimmersdorf Sand may have formed as a result of upward-directed intrusion. In lignite seams within the Lower Rhine Basin, upward-directed transport of sands into overlying lignite seams has previously been described (Bajor, Reference Bajor and Ahrens1958; Berger, Reference Berger and Ahrens1958). Both of these authors noted the presence of redistributed sand deposits within lignite seams of the Ville Formation in former open-cast mines. Bajor (Reference Bajor and Ahrens1958) described sand intrusions (up to 15m thick), as well as fan-like arrangements of sand-filled cracks (where the sands contained lignite clasts) in a location (former Neurath open-cast mine) where the Morken and Frimmersdorf seams are joined to form one thick lignite seam. Emplacement of these intrusions was interpreted as being related to the presence of confined groundwater (Bajor, Reference Bajor and Ahrens1958). Similarly, in the Morken Seam of the Frimmersdorf-Süd open-cast mine a channel deposit with low-angle sand dykes radiating outwards from the channel top into the lignite was described (Berger, Reference Berger and Ahrens1958). The emplacement of these channel-related sand dykes was interpreted as resulting from sand movement related to hydraulic pressure (Berger Reference Berger and Ahrens1958). Hager, Kothen & Spann (Reference Hager, Kothen, Spann, Reiche and Hilden1981) concluded that these various intrusions formed as a result of disequilibrium between hydraulic pressure within the pore waters of the sediment below the lignite seam and settlement of the peat forming these seams. Pore fluids within the underlying sand beds were trapped and led to the generation of overpressure (Hager, Kothen & Spann, Reference Hager, Kothen, Spann, Reiche and Hilden1981).
The presence of some of the sand bodies within the lignite may possibly be related to similar emplacement processes related to overpressuring. Recent work on sand injectites has recognized a series of interrelated processes, namely, (1) liquefaction, (2) fluidization and (3) hydraulic fracturing (e.g. Mazzini et al. Reference Mazzini, Jonk, Duranti, Parnell, Cronin and Hurst2003; Duranti & Hurst, Reference Duranti and Hurst2004; Jonk et al. Reference Jonk, Hurst, Duranti, Parnell, Mazzini and Fallick2005; Braccini et al. Reference Braccini, de Boer, Hurst, Huuse, Vigorito and Templeton2008; Cartwright et al. Reference Cartwright, James, Huuse, Vetel and Hurst2008; Scott, Vigorito & Hurst, Reference Scott, Vigorito and Hurst2009; Hurst, Scott & Vigorito, Reference Hurst, Scott and Vigorito2011). These three processes, acting in conjunction, can lead to the formation of complex patterns of sand distribution within the lignite. The precondition for the formation of sand injectites is the deposition of low-permeability host strata above unconsolidated sands, causing the generation of overpressure within the parent sand body (Jolly & Lonergan, Reference Jolly and Lonergan2002; Jonk et al. Reference Jonk, Hurst, Duranti, Parnell, Mazzini and Fallick2005; Braccini et al. Reference Braccini, de Boer, Hurst, Huuse, Vigorito and Templeton2008).
The Frimmersdorf Sand (in this system representing the parent sand body) consists of well-sorted and fine- to medium-grained, unconsolidated sand beds (Petzelberger, Reference Petzelberger1994). Overpressure in these sands, as noted above, may have been generated by their rapid burial and sealing by low-permeability organic deposits (Jonk et al. Reference Jonk, Hurst, Duranti, Parnell, Mazzini and Fallick2005). Peat accumulation rates on top of the Frimmersdorf Sand have been estimated at 0.1–2mma−1 (Hager, Reference Hager1986). This is comparable to the sedimentation rates of sediments in the South Viking Graben of the North Sea which have also been subjected to sand intrusion (i.e. 50–100mMa−1; Jonk et al. Reference Jonk, Hurst, Duranti, Parnell, Mazzini and Fallick2005). Peat commonly has a high hydraulic permeability, depending on its composition and level of maturity. However, during subsidence the porosity of the peat decreases due to overburden pressure (cf. Taylor et al. Reference Taylor, Teichmüller, Davis, Diessel, Littke and Robert1998, p. 93). Additionally the permeability of lignite may be influenced by external stresses, for example, compressional forces (Somerton, Söylemezoglu & Dudley, Reference Somerton, Söylemezoglu and Dudley1975; Jasinge, Ranjith & Choi, Reference Jasinge, Ranjith and Choi2011). In the Lower Rhine Basin, external stresses may have been related to tectonic movement along the numerous fault systems.
Overpressure in unconsolidated sands can also be generated as a result of so-called disequilibrium compaction (Jolly & Lonergan, Reference Jolly and Lonergan2002; Duranti & Hurst, Reference Duranti and Hurst2004; Braccini et al. Reference Braccini, de Boer, Hurst, Huuse, Vigorito and Templeton2008; Hurst, Scott & Vigorito, Reference Hurst, Scott and Vigorito2011). In such a situation, when the fluid pressure in the pore spaces increases due to the sealing of the sediments, overpressuring may occur (Jolly & Lonergan, Reference Jolly and Lonergan2002; Braccini et al. Reference Braccini, de Boer, Hurst, Huuse, Vigorito and Templeton2008). During compaction, the pore water is expelled from the overlying (and sealing) host strata, and drained into the permeable parent sand body (Jolly & Lonergan, Reference Jolly and Lonergan2002), increasing the amount of pore fluid present. The trapping of these fluids within the pore space precludes an even compaction of the sand body (Braccini et al. Reference Braccini, de Boer, Hurst, Huuse, Vigorito and Templeton2008), and the degree of disequilibrium which ensues can lead to the formation of sand injectites resulting from the forced emplacement of remobilized sand into fractures in the overlying unit (cf. Duranti & Hurst, Reference Duranti and Hurst2004; Scott, Vigorito & Hurst, Reference Scott, Vigorito and Hurst2009).
Disequilibrium compaction may also be an explanation for the lack of any comparable sand bodies within the underlying Morken and overlying Garzweiler seams, when compared with the frequency and extensive distribution of sand bodies in the Frimmersdorf Seam. The Morken and Garzweiler seams are covered by permeable deposits, i.e. the marine Frimmersdorf Sand on top of the Morken Seam, and the coarse-grained sands and gravels of the Hauptkies Formation covering the Garzweiler Seam. These permeable deposits would have facilitated compactional pressure within the underlying lignite beds and the concomitant release of pore water from the peat deposits as a result of ongoing (and increasing) compaction (cf. Hager, Kothen & Spann, Reference Hager, Kothen, Spann, Reiche and Hilden1981). The Frimmersdorf Seam, on the other hand, was capped by the marine sediments of the Neurath Sand. The lowermost metres of the Neurath Sand contain high amounts of clays and silt, which make this unit a sealing rather than a permeable layer. This may have resulted in the development of a degree of disequilibrium compaction and resultant pressure in the underlying peat accumulations (e.g. Hager, Kothen & Spann, Reference Hager, Kothen, Spann, Reiche and Hilden1981).
As noted above, a series of processes are required for the formation of sand injectites commencing with the liquefaction and fluidization of the sediments within the parent unit (e.g. Lowe, Reference Lowe1975; Owen, Reference Owen1996; Hurst & Cronin, Reference Hurst and Cronin2001; Duranti & Hurst, Reference Duranti and Hurst2004; Braccini et al. Reference Braccini, de Boer, Hurst, Huuse, Vigorito and Templeton2008; Hurst, Scott & Vigorito, Reference Hurst, Scott and Vigorito2011). Liquefaction occurs when increased pore-fluid pressure transforms a saturated granular material from a solid into a liquefied state (Duranti & Hurst, Reference Duranti and Hurst2004). Liquefaction of sediments results in the loss of primary sedimentary structures within the parent sand unit (Braccini et al. Reference Braccini, de Boer, Hurst, Huuse, Vigorito and Templeton2008; Hoffmann & Reicherter, Reference Hoffmann and Reicherter2012). This homogenization of primary sedimentary structures can be observed within the uppermost metres of the Frimmersdorf Sand (cf. Section 3.h) and may also result in the disintegration or redistribution of wood fragments possibly belonging to former root horizons. The remobilization of this liquefied material (= fluidization) occurs via the upwards-moving pore fluids which follow the hydraulic gradient towards the sediment surface (Jolly & Lonergan, Reference Jolly and Lonergan2002; Cartwright et al. Reference Cartwright, James, Huuse, Vetel and Hurst2008).
The presence of cracks within the host sediment body is considered to be the result of hydraulic fracturing (e.g. Jolly & Lonergan, Reference Jolly and Lonergan2002; Mazzini et al. Reference Mazzini, Jonk, Duranti, Parnell, Cronin and Hurst2003; Duranti & Hurst, Reference Duranti and Hurst2004; Jonk et al. Reference Jonk, Hurst, Duranti, Parnell, Mazzini and Fallick2005; Cartwright et al. Reference Cartwright, James, Huuse, Vetel and Hurst2008), generated when the overpressure within the pore-water filled sediment is greater than the fracture strength of the host sediment. When passing a plane of minor competence contrast (Cartwright et al. Reference Cartwright, James, Huuse, Vetel and Hurst2008), such as bedding planes, the pressure may be directed parallel to the bedding, resulting in vertical dykes that merge into sills (Fig. 6b).
Commonly, sand injection occurs at pre-existing, dilated fractures (e.g. Jolly & Lonergan, Reference Jolly and Lonergan2002; Mazzini et al. Reference Mazzini, Jonk, Duranti, Parnell, Cronin and Hurst2003; Duranti & Hurst, Reference Duranti and Hurst2004; Jonk et al. Reference Jonk, Hurst, Duranti, Parnell, Mazzini and Fallick2005; Cartwright et al. Reference Cartwright, James, Huuse, Vetel and Hurst2008). The orientations of these pre-existing fractures may thus reflect the regional stress directions (Jolly & Lonergan, Reference Jolly and Lonergan2002; Figs 4a, 7, 8).
Fluidization of water-saturated sands and their transport within fracture systems may result in significant erosion of the host strata (Duranti & Hurst, Reference Duranti and Hurst2004; Hurst, Scott & Vigorito, Reference Hurst, Scott and Vigorito2011). The erosion of the bounding peat during transport of the fluidized sands may lead to the intermixture of fine particles of organic detritus with the sand grains. The enrichment of peat detritus in the pore spaces of the sand bodies has been observed in thin-section (cf. Fig. 10). Additionally peat and wood fragments that are present within the sand bodies may have been derived from wall erosion as the fluidized sand moved within the dyke.
The deposition of fluidized sands may form a type of lamination, which forms due to grain-size variations, as well as variations in grain packing, and is orientated parallel to the margins of the sand bodies (Scott, Vigorito & Hurst, Reference Scott, Vigorito and Hurst2009; Hurst, Scott & Vigorito, Reference Hurst, Scott and Vigorito2011). Within the sampled sill-like sand bodies, the presence of this lamination is emphasized by the elongate orientation of the sand grains and wood fragments parallel to the sill margins.
Though fluidization would commonly appear to be restricted to sands (generally fine- to medium grained sands; Lowe, Reference Lowe1975; Hurst, Scott & Vigorito, Reference Hurst, Scott and Vigorito2011), gravel-sized sediments can also become liquefied and remobilized (Fontana et al. Reference Fontana, Lugli, Marchetti Dori, Caputo and Stefani2015). Therefore, syn-sedimentary sand bodies which contain chert pebbles may also have been reworked as a result of liquefaction and fluidization processes.
Liquefaction and fluidization processes may also have influenced syn-depositional sand bodies in the Frimmersdorf Seam. The combination of apparently syn-depositional, cross-laminated sand bodies with upward or laterally spreading dykes can be explained by the remobilization of the unconsolidated sands. The reworking of the syn-sedimentary sand bodies may result in the homogenization of former sedimentary structures (Braccini et al. Reference Braccini, de Boer, Hurst, Huuse, Vigorito and Templeton2008; Hoffmann & Reicherter, Reference Hoffmann and Reicherter2012), which may explain why sedimentary structures are relatively rare within the majority of sand bodies in the Frimmersdorf Seam.
The generation of overpressure in water-saturated sands, and the final remobilization of the sediments, may also have been initiated by seismic activity (e.g. Obermeier, Reference Obermeier1996; Jolly & Lonergan, Reference Jolly and Lonergan2002; Duranti & Hurst, Reference Duranti and Hurst2004; Jonk et al. Reference Jonk, Hurst, Duranti, Parnell, Mazzini and Fallick2005; Braccini et al. Reference Braccini, de Boer, Hurst, Huuse, Vigorito and Templeton2008). Fault activity can lead to rapid and sudden increases in pore pressure in unconsolidated sediments, resulting in hydraulic fractioning and the remobilization of sands within the parent unit (Duranti & Hurst, Reference Duranti and Hurst2004). The possibility that earthquakes may have been, at least partly, responsible for the emplacement of sand bodies within the seam is supported by the fact that the region is one of ongoing active seismicity (Ziegler, Reference Ziegler1992; Klostermann, Kremers & Röder, Reference Klostermann, Kremers and Röder1998; Hinzen, Reference Hinzen2003; Sissingh, Reference Sissingh2003; Ewald et al. Reference Ewald, Igel, Hinzen and Scherbaum2006). Tectonic activity in the Lower Rhine Basin occurs along the numerous fault systems, generated from the extensional forces which formed the rift basin, as well as forming the main faults for the ongoing subsidence of the basin (Nickel, Reference Nickel2003; Schäfer et al. Reference Schäfer, Utescher, Klett and Valdivia-Manchego2005). In particular, the Erft Fault System is considered to have been a major centre for seismic activity, since it has controlled the uplift (hundreds of metres) of the western Köln Block. Small-scale evidence of tectonically induced deformation of sand bodies includes the presence of normal faults within associated concordant or sill-like sand bodies which have vertical offsets of several centimetres (Fig. 6c, d).
Sand injectite studies have, to date, mainly concentrated on consolidated sandstone injectites in mudstone host strata, and exposed in outcrops (Petersen, Reference Petersen1968; Ewald et al. Reference Ewald, Igel, Hinzen and Scherbaum2006; Braccini et al. Reference Braccini, de Boer, Hurst, Huuse, Vigorito and Templeton2008; Scott, Vigorito & Hurst, Reference Scott, Vigorito and Hurst2009; Hurst, Scott & Vigorito, Reference Hurst, Scott and Vigorito2011), although injectite complexes in subaqueous deposits of the North Sea are increasingly reported in the literature (Duranti & Hurst, Reference Duranti and Hurst2004; Jonk et al. Reference Jonk, Hurst, Duranti, Parnell, Mazzini and Fallick2005; Cartwright et al. Reference Cartwright, James, Huuse, Vetel and Hurst2008; Scott, Vigorito & Hurst, Reference Scott, Vigorito and Hurst2009). Studies of subaqueous sand injectites, however, are difficult since only small sections of drill core can be sampled and analysed. Therefore, it is difficult to distinguish between syn-sedimentary and injected sand bodies within the basins (Duranti & Hurst, Reference Duranti and Hurst2004). Since injection structures can affect oil and gas reservoirs, for example, in allowing leakage of hydrocarbons, these sand bodies are of great scientific and economic interest (Jolly & Lonergan, Reference Jolly and Lonergan2002; Braccini et al. Reference Braccini, de Boer, Hurst, Huuse, Vigorito and Templeton2008). Sand bodies in the Garzweiler open-cast mine, due to their accessibility, provide the possibility to study unconsolidated sand injectites in outcrops which are constantly being renewed, since it is an active mine, as well as allowing a three-dimensional view of these sand bodies. In addition, the relation of the parent unit, i.e. the Frimmersdorf Seam, with the sand bodies within the host strata can be analysed.
4.c. Time frame
The current results do not allow the formation of the sand bodies to be accurately located within the formational time frame, apart from a broad assignment as either syn- and post-depositional (cf. Table 2). However, there are certain aspects which do shed more light on the interaction of sand emplacement and lignite formation, for example (a) the chronology of tectonic activity within the Lower Rhine Basin, (b) the properties of peat and lignite during subsidence and settlement and (c) the ductile deformation of peat clasts within some of the post-depositional sand bodies.
Table 2. Broad time frame for the development of the various structures observed within the lignite of the Frimmersdorf Seam.

Tectonic activity (a), which is considered to represent one possible mechanism for the formation of sand injectites, was particularly high during Oligocene times, decreased in importance during Miocene times, and again increased from Messinian times onward (Schäfer et al. Reference Schäfer, Utescher, Klett and Valdivia-Manchego2005). The reduction in tectonic activity in Miocene times (Sissingh Reference Sissingh2003), resulting from the W-directed shift of the Alpine Orogeny (Klostermann, Kremers & Röder, Reference Klostermann, Kremers and Röder1998), may indicate that formation of sand injectites was coeval with the renewed seismic activity in the Messinian.
The settlement and compaction of peat (b), which influences its sealing properties, is primarily dependent on its initial thickness. Comparatively thick peat accumulations (e.g. the Main Seam, with a thickness of c.100m of lignite) commonly undergo increased compaction due to their own weight, whereas thinner peat accumulations (e.g. the Morken or the Frimmersdorf seams, c.15m lignite) only undergo significant compaction when overburden pressure increases as a result of the deposition of thick sediment units above (Hager, Kothen & Spann, Reference Hager, Kothen, Spann, Reiche and Hilden1981). This may suggest that the formation of the sand injectites in the Frimmersdorf Seam was related to the increase in overburden due to the deposition of the Neurath Sand and Garzweiler Seam (and possibly including the overlying upper Miocene, Pliocene and Pleistocene-age deposits).
The presence of rounded lignite clasts (c) and, in particular, ductilely deformed lignite lenses indicates, however, that the emplacement of the sand bodies containing these lenses occurred during the peat generation stage. If the organic material had attained the lignite stage any redistributed material would have shown brittle behaviour.
5. Conclusions
The highly variable sand bodies present within the Frimmersdorf Seam of the Garzweiler open-cast mine suggest that a variety of causal mechanisms may have been responsible for their emplacement. Both syn- and post-depositional processes, acting in isolation or in combination, can be used to provide a suitable model for the formation of all the described sand bodies.
Coeval with peat formation, the sand bodies were deposited in an estuarine environment. Within this Miocene-age estuary, sand bodies were formed by the interaction of river and tidal influences, supplemented by aperiodic events, e.g. fluvial flooding or storm surges.
The post-depositional sand bodies may have formed due to the injection of fluidized sand into the peat accumulations. The liquefaction and fluidization of the parent sand body, as well as the hydraulic fracturing of the sealing host strata (i.e. lignite), were related to the generation of overpressure within the sands, while fracturing was also possibly linked to seismic activity within the Lower Rhine Basin.
Acknowledgements
We acknowledge the funding for this study from the RWE Power AG. Thanks to the staff members of the department GOC-L, especially the field crew of the Garzweiler open-cast mine, as well as Dr Thomas Thielemann and Sonja Weiler for editing datasets. Special thanks to Dr Friederike Bungenstock (NIhK Wilhelmshaven) for providing insight into recent swamp environments around the Jadebusen (North Sea). The manuscript benefited greatly from the comments of Gösta Hoffmann and an anonymous reviewer.