Introduction
Uranium is commonly deposited in geochemical environments where a solution containing U6+ is met by reducing conditions created by organic matter, ferrous-iron minerals or sulfides, leading to precipitation of uraninite (Langmuir, Reference Langmuir1978). Oxidizing groundwater can re-solubilize uranium and alter sulfides, leading to the formation of sulfate (Garrels and Christ, Reference Garrels, Christ, Garrels and Larsen1959; Finch and Murakami, Reference Finch, Murakami, Burns and Ewing1999; Plášil, Reference Plášil2014). Subsequent evaporation in the presence of cations (e.g. Na+, K+ or Mg2+) can lead to crystallization of uranyl sulfate minerals, most commonly those of the zippeite group (Brugger et al., Reference Brugger, Meisser and Burns2003). The majority of natural and synthetic analogues of zippeite-group minerals have refined structures and crystal-chemical variations observed in the group are well-established (Burns et al., Reference Burns, Deely and Hayden2003; Brugger et al., Reference Brugger, Wallwork, Meisser, Pring, Ondruš and Čejka2006; Peeters et al., Reference Peeters, Vochten and Blaton2008; Plášil et al., Reference Plášil, Dušek, Novák, Čejka, Císařová and Škoda2011a,Reference Plášil, Mills, Fejfarová, Dušek, Novák, Škoda, Čejka and Sejkorab, Reference Plášil, Fejfarová, Škoda, Dušek, Čejka and Marty2013, Reference Plášil, Dušek, Čejka and Sejkora2014; Plášil, Reference Plášil2015; Plášil and Škoda, Reference Plášil and Škoda2015; Plášil et al., Reference Plášil, Škácha, Sejkora, Kampf, Škoda, Čejka, Hloušek, Kasatkin, Pavlíček and Babka2017, Reference Plášil, Petříček, Mills, Favreau and Galea-Clolus2018). The interlayer composition and configuration of group members is diverse, due to the ability of the zippeite sheet to shift its charge and accommodate variable amounts of monovalent, divalent, and even trivalent cations. Redcanyonite is a new NH4 and Mn-bearing member of the zippeite group, which now includes 12 unique minerals.
Redcanyonite is named after Red Canyon in southeast Utah, and alludes to the red and orange hues of iron-stained sandstones within the canyon, which are also adopted in the striking colour of the new mineral. Red Canyon is the type locality for 22 recently-described uranium minerals, with several more currently under study that we are aware of. The region has produced a remarkable number of unique uranyl minerals that are only found there; in particular, several uranyl sulfates. Extensive oxidation of ore minerals (uraninite and sulfides) coupled with inflow of dry desert air has favoured crystallization of uranyl minerals. Episodic dissolution and re-precipitation occurring between wet and dry periods augments the complexity and diversity of uranyl sulfates found there.
The mineral and its name were approved by the Commission on New Minerals, Nomenclature and Classification of the International Mineralogical Association (IMA2016-082). Six co-type specimens are deposited in the collections of the Natural History Museum of Los Angeles County, 900 Exposition Boulevard, Los Angeles, CA 90007, USA, under the catalogue numbers 66293, 66294, 66295, 66296, 66297 and 66298.
Occurrence
Redcanyonite occurs underground in the Blue Lizard mine, Red Canyon, White Canyon mining district, San Juan County, Utah (37°33'26”N, 110°17'44”W). Red Canyon is located in west-central San Juan County, in southeastern Utah (Fig. 1). The Blue Lizard mine is situated on the northern ridge of Red Canyon, ~1 km to the northeast of the Markey mine and ~22 km southeast of Good Hope Bay on Lake Powell. Detailed historical and geological information on the Blue Lizard mine is described elsewhere (Kampf et al., Reference Kampf, Plášil, Kasatkin and Marty2015), and is derived primarily from a report by Chenoweth (Reference Chenoweth1993).

Fig. 1. Topographical map (Garmin MapSource®) highlighting important mines within Red Canyon, San Juan County, Utah, USA.
Redcanyonite is amongst the rarest of the uranyl sulfates found in the Blue Lizard mine and has so far been found only in a small (~0.5 m2) area of the mine. Its rarity is probably due to a dependence on the availability of Mn2+ and co-existence with NH4+. Secondary uranium mineralization in Red Canyon is often localized and most prevalent within organic-rich beds that are laced with uraninite and sulfides. We infer the source of NH4+ is from decomposition of organic material, but were unable to identify the source of Mn2+. The new mineral occurs intimately with ammoniozippeite in several specimens, which was previously only known as a synthetic phase. Other associated secondary minerals include bobcookite, brochantite, devilline, gypsum, johannite, posnjakite, natrozippeite, pentahydrite and pickeringite.
Physical and optical properties
Redcanyonite occurs as radial aggregates of needles and blades individually measuring up to 0.2 mm long; the aggregates are up to 1 mm in diameter (Figs 2 and 3). Crystals are flattened on {010} and elongated on [100], and exhibit the forms {001}, {010}, {101} and {10$\bar{1}$} (Fig. 4). Many crystals are twinned by 180° rotation on [100]. Crystals are translucent with a vitreous lustre, pale orange streak, and are non-fluorescent under both longwave and shortwave ultraviolet illumination. The mineral is easily soluble in room-temperature dilute HCl. The Mohs hardness is ~2, estimated by the behaviour of crystals when broken. Crystals of redcanyonite are brittle with perfect {010} cleavage and uneven fracture. The density could not be measured due to the limited availability of material, and because it exceeds that of known heavy liquids. The calculated density is 4.633 g cm–3 based on the empirical formula, and 4.688 g cm–3 for the ideal formula.

Fig. 2. Spray of redcanyonite needles on brochantite and devilline. Horizontal field of view is ~0.6 mm.

Fig. 3. Sprays of bladed redcanyonite with bluish-green devilline, and white pentahydrite. Horizontal field of view is ~1.2 mm.

Fig. 4. Crystal drawing of redcanyonite; clinographic projection in non-standard orientation (a vertical).
Optically, redcanyonite is biaxial (+), with α = 1.725(3), β = 1.755(3) and γ = 1.850(5) (measured in white light). The measured 2V is 60(2)°, based on extinction data collected on a spindle stage and analysed using EXCALIBRW (Gunter et al., Reference Gunter, Weaver, Bandli, Bloss, Evans and Su2004); the calculated 2V is 61.3°. Dispersion is very strong, r < v. The mineral is pleochroic with X = orange, Y = yellow and Z = orange; Y << X < Z. The optical orientation is X = b, Y ≈ c*, Z ≈ a.
The Gladstone-Dale compatibility, 1 – (K p/K c), is 0.001 (superior) for the ideal formula, and 0.065 (fair) for the empirical formula (Mandarino, Reference Mandarino2007) using the value for k(UO3) = 0.134, given by Larsen (Reference Larsen1921).
Infrared spectroscopy
Attenuated total reflectance (ATR) Fourier-transform infrared (FTIR) spectra were obtained using a liquid N2 cooled SENSIR Technologies IlluminatIR mounted to an Olympus BX51 microscope. An ATR objective was pressed into crystals of redcanyonite and measured from 4000 to 650 cm–1. The infrared spectrum of redcanyonite is shown in Fig. 5, and assignments are based on those of Čejka (Reference Čejka, Burns and Ewing1999). A series of broad bands observed between ~3500 cm–1 and ~2800 cm–1 are attributed to the ν O–H stretching vibrations of hydrogen-bonded water molecules, overlapped with N–H stretching vibrations from interlayer NH4+ molecules. Due to overlap, attributing specific assignments to these bands is difficult. Approximate O–H⋯O hydrogen donor–acceptor distances calculated from the observed stretching frequencies are in the range ~2.9 to 2.6 Å using the correlation function given by Libowitzky (Reference Libowitzky1999) and correspond well to those measured by single-crystal X-ray diffraction. A weak band found at 1615 cm–1 is assigned as the ν2 (δ)-bending vibration of hydrogen-bonded crystalline water. A medium strong band at 1408 cm–1 is assigned to the N–H bending vibration of NH4+ molecules (Pekov et al., Reference Pekov, Krivovichev, Yapaskurt, Chukanov and Belakovskiy2014).

Fig. 5. Infrared spectrum (ATR) of redcanyonite in the region from 4000 to 600 cm–1.
Absorption bands at 1155, 1140 and 1084 cm–1 are assigned to the split triply degenerate ν3 (SO4)2– antisymmetric stretching vibration. The ν1 (SO4)2– symmetric stretch, which usually occurs between ~1100–1000 cm−1 is enveloped by the ν3 (UO2)2+ antisymmetric stretch. The very strong antisymmetric ν3 (UO2)2+ stretch occurs as a relatively broad band at 940 cm–1. A weak band at 836 cm–1 is attributed to the ν1 (UO2)2+ symmetric stretch, which although forbidden by selection rules, may be observed due to slight distortions. The uranyl U–O bond lengths inferred from the IR spectrum of redcanyonite using the empirical relation given by Bartlett and Cooney (Reference Bartlett and Cooney1989) are 1.77 Å (ν3) and 1.78 Å (ν1).
Raman spectroscopy
The Raman spectrum of redcanyonite was taken using a Bruker Instruments Sentinel-785 laser head mounted on a Nikon Optiphot-2 microscope with Peltier-cooled integrated 785 nm diode laser, operated at 200 mW, 100 µm spot size and ~5 cm–1 resolution. The spectrometer was calibrated using software-controlled procedures (Opus software) from neon emission lines (wavelength calibration), and Tylenol® Raman bands (frequency calibration). A background correction was applied using the Opus software.
The Raman spectrum of redcanyonite is given in Fig. 6. The split triply degenerate ν3 (SO4)2– antisymmetric stretching vibrations occur as weak bands at 1263, 1158 and 1097 cm–1, and a medium intensity band at 1013 cm–1 is assigned to the ν1 (SO4)2– symmetric stretching vibration. The ν1 (UO2)2+ symmetric stretching vibration is present as a very strong band at 819, with a shoulder at 809 cm–1. Bartlett and Cooney (Reference Bartlett and Cooney1989) provide an empirical relation to derive the approximate U–Oyl bond lengths from the band positions assigned to the (UO2)2+ stretching vibrations, which gives 1.79 Å (819 cm–1) and 1.80 Å (809 cm–1), in agreement with U–Oyl bond lengths from the X-ray data (see below).

Fig. 6. Raman spectrum of redcanyonite, taken with a 785 nm laser.
Weak bands at 666 and 601 cm–1 are attributed to the split, triply degenerate ν4(δ)(SO4)2– bending vibrations, and those at 506, 464 and 418 cm–1 to the split doubly degenerate ν2(δ)(SO4)2– bending vibrations. Bands at 354 and 329 cm–1 are attributed to ν (U–Oequatorial) stretching vibrations, and bands found at 284 and 261 arise from ν2(δ)U–O–U bending modes. The remaining bands at 206, 176, 150 and 127 cm–1 are assigned to external lattice vibration modes and UO22+ translations and rotations (Plášil et al., Reference Plášil, Buixaderas, Čejka, Sejkora, Jelička and Novák2010).
Chemical composition
Chemical analyses (five) were performed using a Cameca SX-50 electron microprobe (University of Utah), operating at an accelerating voltage of 15 kV, with a beam current of 10 nA and 5 µm spot diameter. Redcanyonite contains appreciable U, N, Mn and S, with some Zn and Cu. No other elements were detected. Matrix effects were accounted for using the PAP correction routine (Pouchou and Pichoir, Reference Pouchou, Pichoir and Armstrong1985), and the analytical data are given in Table 1. The small and frail crystals were difficult to polish and exhibited moderate beam damage, resulting in low totals (96.22 wt.%). Due to the limited amount of material available the H2O content was not measured and is instead calculated based on the structure. The presence of H2O and NH4 were confirmed by infrared spectroscopy. The empirical formula, calculated on the basis of 4 U and 24 O atoms per formula unit (apfu) is (NH4)2.02(Mn0.49Cu0.09Zn0.06)Σ0.64H+0.72[(UO2)4O4(S0.99P0.01O4)2](H2O)4. The ideal formula is (NH4)2Mn[(UO2)4O4(SO4)2](H2O)4 which requires (NH4)2O 3.48, SO3 10.68, MnO 4.73, UO3 76.30, H2O 4.81, for a total 100 wt.%.
Table 1. Data from electron microprobe analysis for redcanyonite, average of five analyses.

*Calculated based on the structure
S.D. – standard deviation
Powder X-ray diffraction
Room-temperature powder diffraction data (Table 2) were recorded using a Rigaku R-Axis Rapid II curved imaging plate microdiffractometer with monochromated MoKα radiation. A Gandolfi-like motion on the φ and ω axes was used to randomize diffraction from the sample. Observed d-values and intensities were derived by profile fitting using JADE 2010 software (Materials Data, Inc.). Unit-cell parameters refined from the powder data using whole pattern fitting in JADE 2010 are as follows: a = 8.665(2), b = 14.359(2), c = 8.834(2) Å, β = 104.190(5)° and V = 1065.5(4) Å3.
Table 2. Powder X-ray data (d in Å) for redcanyonite.

Single-crystal X-ray diffraction
Single crystal X-ray diffraction data were collected using an Apex II CCD-based detector and MoKα X-rays from a microfocus source mounted to a Bruker Quazar three-circle diffractometer. Corrections for Lorentz, polarization and background effects were made using the Bruker program SAINT. A multi-scan semi-empirical absorption correction was applied using equivalent reflections in SADABS-2012. An initial structure model was obtained by the charge-flipping method using SHELXT (Sheldrick, Reference Sheldrick2015) in space group C2/m. The JANA2006 software package (Petříček et al., Reference Petříček, Dušek and Palatinus2014) was used to refine the structure of redcanyonite on the basis of F 2 for unique reflections, to a final R 1 of 3.75% for 1079 reflections with I obs > 3σI. Details regarding the data collection and refinement results are given in Table 3, and atomic coordinates and displacement parameters in Table 4. The crystallographic information files have been deposited with the Principal Editor of Mineralogical Magazine and are available as Supplementary material (see below). Hydrogen atoms could not be located due to weak X-ray scattering, however hydrogen-bonding information is proposed on the basis of selected interatomic distances given in Table 5, and a bond-valence analysis in Table 6. Uranium, S, Mn and O atoms of the sheet were refined using harmonic atomic displacement parameters, and the remaining atoms (interlayer N and Ow) were refined isotropically. Due to the small size of the crystals, weak and diffuse reflections requiring doubling of the c parameter are present in the diffraction pattern, but attempts to integrate and refine these reflections were difficult, and refinement in a cell with c = ~17 Å led to overall unsatisfactory results. The crystal of redcanyonite chosen for diffraction was twinned, and applying the twin law ($\bar{1}$,0,½/0,
$\bar{1}$,0/0,0,1) led to an improvement in the R factor by ~5%.
Table 3. Data collection and structure-refinement details for redcanyonite.
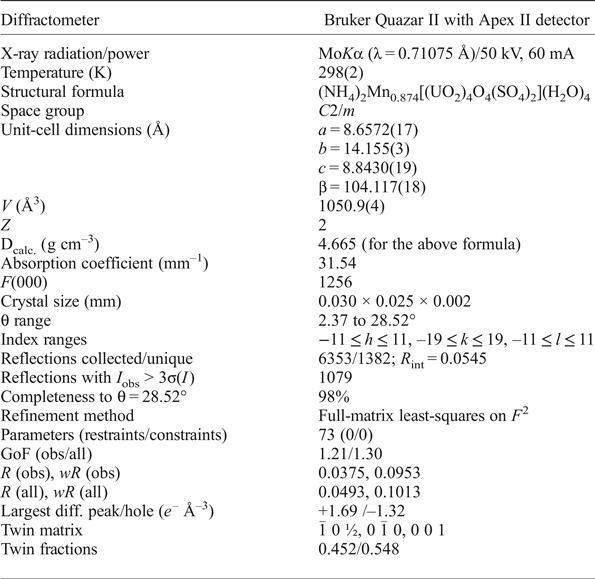
Table 4. Atomic coordinates and displacement parameters (U eq, U iso, in Å2) for redcanyonite.

*Refined occupancy of Mn1 is 0.218
Table 5. Selected interatomic distances (Å) in the structure of redcanyonite.

Table 6. Bond-valence analysis (valence units, vu) for redcanyonite.

Bond-valence parameters for U6+–O, Mn2+–O, S6+–O from Gagné and Hawthorne (Reference Gagné and Hawthorne2015); and NH4+···O from García-Rodríguez et al. (Reference García-Rodríguez, Rute-Pérez, Piñero and González-Silgo2000). Expected hydrogen bond-valence contributions are included, with values for donor (D—H, ~0.8 vu) and acceptor (H—A, ~0.2 vu) bond strengths taken from Hawthorne and Schindler (Reference Hawthorne and Schindler2008).
Features of the crystal structure
The structure of redcanyonite contains one symmetrically distinct U site, with one S site, one Mn site and seven O sites. Two multiply-bonded oxygen atoms are arranged in a linear fashion about each uranium atom, forming the uranyl unit (UO2)2+. Five O anions sit at the equatorial vertices of a uranyl pentagonal bipyramid, capped on top and bottom by uranyl oxygen atoms Oyl. Each sulfur cation (S6+) is tetrahedrally coordinated, with < S–O > bond lengths ranging from 1.44 to 1.49 Å.
The uranyl pentagonal bipydramids and sulfate tetrahedra are linked to form the well-known zippeite type (Burns, Reference Burns2005). It consists of zigzag chains of uranyl pentagonal bipyramids two-polyhedra wide that extend along [100], where individual chains link to form a sheet by sharing equatorial vertices with sulfate tetrahedra. Each sulfate tetrahedron links four unique bipyramids (two bipyramids of two separate chains) and propagates the zippeite-type sheet along [001]. Individual sheets stack parallel to (010), which corresponds to the excellent cleavage. Sheets are linked through a network of H bonds that emanate from interstitial water and ammonium groups (Fig. 7). Each ammonium group bonds to two water molecules within the interlayer (Ow1), four Oyl atoms (two from each sheet, O4 and O5), and two equatorial oxygen atoms (one from each sheet, O2) which are part of the SO4 tetrahedra. Additional linkages between sheets are made through Mn(H2O)2O4 octahedra, where the apex of each octahedron correspond to two water molecules of the interlayer (Ow2), and four O atoms of uranyl ions (O4).

Fig. 7. Polyhedral representation of the structure of redcanyonite. Uranium (yellow), sulfur (blue), manganese (orange), nitrogen (light blue), oxygen (red).
According to the chemical analyses, redcanyonite contains variable Mn2+, Cu2+ and Zn2+ in the interlayer and site-scattering refinement performed during the final cycles of refinement gives 0.874 Mn apfu. Chemical and configurational variability is a recurring feature in natural zippeite-group phases (e.g. Brugger et al., Reference Brugger, Meisser and Burns2003; Plášil et al., Reference Plášil, Dušek, Novák, Čejka, Císařová and Škoda2011a,Reference Plášil, Mills, Fejfarová, Dušek, Novák, Škoda, Čejka and Sejkorab, Reference Plášil, Fejfarová, Škoda, Dušek, Čejka and Marty2013, Reference Plášil, Dušek, Čejka and Sejkora2014; Plášil and Škoda, Reference Plášil and Škoda2015) and synthetic phases (Burns et al., Reference Burns, Deely and Hayden2003; Peeters et al., Reference Peeters, Vochten and Blaton2008). Similarly, Frondel et al. (Reference Frondel, Ito, Honea and Weeks1976) observed considerable solid solution between end-member compositions of synthetic NH4, Ni, Co and Mg-containing zippeites. It is also common within this group for phases with similar compositions to have slightly different interlayer arrangements (Plášil et al., Reference Plášil, Fejfarová, Škoda, Dušek, Čejka and Marty2013). The structure of redcanyonite is most closely related to plavnoite (Plášil et al., Reference Plášil, Škácha, Sejkora, Kampf, Škoda, Čejka, Hloušek, Kasatkin, Pavlíček and Babka2017), ammoniozippeite (Kampf et al., Reference Kampf, Plášil, Olds, Nash and Marty2018), and other monoclinic members of the zippeite group containing M 2+. It is the first naturally occurring zippeite-group mineral that contains NH4+ groups in the interlayer, and differs from others by the unique arrangement of Mn2+ and NH4+ (Fig. 8).

Fig. 8. A comparison of interlayer arrangement in redcanyonite, ammoniozippeite and plavnoite as viewed down b. Manganese (orange), nitrogen (light blue), potassium (purple), oxygen (red), uranium (yellow) and sulfur (blue).
Refinement indicates that structural units in redcanyonite have the composition [(UO)4O4(SO4)2]4–, although some sheets in zippeite minerals contain hydroxyl. The calculated bond-valence sum of the μ3-bridging oxygen atom O3 is 1.87 valence units (vu), inconsistent with OH–. Where this site contains OH– in other zippeite-group phases, sums range between 1.27–1.4 vu (e.g. Burns et al., Reference Burns, Deely and Hayden2003; Brugger et al., Reference Brugger, Meisser and Burns2003; Plášil et al., Reference Plášil, Dušek, Novák, Čejka, Císařová and Škoda2011a,Reference Plášil, Mills, Fejfarová, Dušek, Novák, Škoda, Čejka and Sejkorab; Plášil and Škoda, Reference Plášil and Škoda2015). The lack of evidence for sheet hydroxyl in redcanyonite in addition to partially occupied interlayer constituents may suggest that minor H+ (as H3O+) is associated with interlayer water molecules to provide the charge balance. Such is the case for natural zippeite (Plášil et al., Reference Plášil, Mills, Fejfarová, Dušek, Novák, Škoda, Čejka and Sejkora2011b), with an interlayer composition of K1.85H+0.15. Similarly, the interlayer composition of the related zippeite-group phase sejkoraite-(Y) (Plášil et al., Reference Plášil, Dušek, Novák, Čejka, Císařová and Škoda2011a) is (Y1.98Dy0.24)Σ1.82H+0.34, where excess H+ accounts for charge-balance deficiencies from partially occupied M 3+. Some complications with this assignment arise due to artefacts from site-scattering refinement and inadequacies of microprobe analyses, although, that zippeite-group members are formed under acidic conditions supports the existence of H3O+. We cannot rule out partial sheet-protonation in redcanyonite, however, as in other zippeite-group minerals the charge balancing mechanism for partial Mn occupancy in redcanyonite may involve excess H+ (as H3O+) dispersed in the interlayer, or excess NH4+.
Yellow crystals of ammoniozippeite are found to occur intimately with redcanyonite in some of the samples under study, and structure refinement suggests ammoniozippeite crystallizes in a cell related to redcanyonite, but with vacancy at the corresponding Mn site. Its structural sheet is not protonated; consistent with the observations by Burns et al. (Reference Burns, Deely and Hayden2003) for synthetic NH4-zippeite designated ‘SZIPPNH4I’. Thus, interlayer compositions between redcanyonite and ammoniozippeite may form a solid-solution series, which can be written as (NH4)2+2xMn1–x, assuming that no sheet protonation or interlayer H3O+ is present. The formula of redcanyonite from the structure refinement is (NH4)2Mn0.874[(UO2)4O4(SO4)2](H2O)4 with Z = 2 and D calc = 4.665 g cm–3, which has a net –0.25 charge. With this in mind, the structural formula can be balanced with respect to solid solution towards ammoniozippeite as (NH4)2.26Mn0.874[(UO2)4O4(SO4)2](H2O)4. However, charge balance may also be met by calling upon excess interlayer H+ (NH4)2Mn0.874H+0.26[(UO2)4O4(SO4)2](H2O)4. Either assignment is plausible in the absence of H-atom positions, and we can neither confirm nor deny the presence of H3O+ or excess NH4+ in the interlayer from the X-ray data. Future work requires application of neutron diffraction or spectroscopic techniques sensitive to H atoms to resolve the role of H3O+ in redcanyonite and other zippeite-group minerals.
Conclusions
Humans can have a significant influence on Earth's mineral diversity (Hazen et al., Reference Hazen, Grew, Origlieri and Downs2017). We owe ourselves partial credit for the occurrence of chemically and structurally complex uranyl sulfate minerals found in Red Canyon. Human activity (mining and removal of rock) has accelerated secondary mineralization of uranium there, and has allowed us to study the formation of uranyl sulfate structures. Redcanyonite has formed because mining facilitated interactions between Mn2+, possibly derived from primary oxide or sulfide sources, and NH4+ formed by decomposition of organic material. Such “human mediated mineralogy” (Hazen et al., Reference Hazen, Grew, Origlieri and Downs2017) has produced unique geochemical conditions in Red Canyon, and recognizing them is useful from an environmental standpoint. For example, successful remediation of sulfide-rich uranium mine tailings, or storage of actinide-bearing wastes in a geological repository are both dependent on preventing interactions between actinides, anthropogenical materials and acidic sulfate groundwater. The Red Canyon region has, and will probably continue, to provide significant advances to our understanding of uranyl minerals and uranyl sulfates formed by alteration of underground mine workings.
Table 7. A comparison of related phases with the zippeite sheet topology.

Acknowledgements
We thank two anonymous reviewers for valuable comments that improved the quality of this manuscript. Support for this work is provided by the Chemical Sciences, Geosciences and Biosciences Division, Office of Basic Energy Sciences, Office of Science, U.S. Department of Energy, Grant No. DE-FG02-07ER15880. We thank the ND Energy Materials Characterization Facility for use of the single-crystal X-ray diffraction and Raman spectroscopy instruments. A portion of this study was funded by the John Jago Trelawney Endowment to the Mineral Sciences Department of the Natural History Museum of Los Angeles County. Jakub Plášil thanks support from GACR, grant no. 17-09161S.
Supplementary material
To view supplementary material for this article, please visit https://doi.org/10.1180/minmag.2017.081.094