1. Introduction
Mafic intrusive rocks originated from subduction-induced magmas record valuable information on mantle processes, as their geochemical characteristics provide keys to the origin of mantle melt sources, magma evolution (partial melting, melt migration, assimilation contamination, fractional crystallization) and partial melting conditions (temperature, pressure, oxygen fugacity, aqueous fluid occurrence and compositions) (Duncan, Reference Duncan1987). The petrogenesis of mafic rocks is therefore important in investigations of regional tectonic evolution, the nature of mantle source (i.e. compositional range, heterogeneity, fertile versus depleted), and crust–mantle interactions (Dilek & Altunkaynak, Reference Dilek, Altunkaynak, van Hinsbergen, Edwards and Grovers2009; Su et al. Reference Su, Qin, Santosh, Sun and Tang2013).
Previous studies of Early Jurassic (190–180 Ma) bimodal volcanic rocks, ultramafic–mafic intrusions and A-type granitoids in the Songnen–Zhangguangcai Block (NE China) suggest a continental back-arc extensional setting of their formation related to westward subduction of the palaeo-Pacific plate (Wu et al. Reference Wu, Sun, Li, Jahn and Wilde2002; Tang et al. Reference Tang, Xu, Wang, Gao and Cao2011; Yu et al. Reference Yu, Wang, Xu, Gao and Pei2012; Xu et al. Reference Xu, Pei, Wang, Meng, Ji, Yang and Wang2013; Guo et al. Reference Guo, Li, Fan, Li, Zhao, Huang and Xu2015). These mafic rocks are similar in their geochemical signatures to island-arc basalts, characterized by enrichments in large-ion lithophile (LILEs) and light rare-earth elements (LREEs), and depletions in high-field-strength elements (HFSEs) that were likely due to metasomatism of the mantle source by sediment-derived melts and fluids released by dehydration of the subducting slab (Yu et al. Reference Yu, Wang, Xu, Gao and Pei2012; Guo et al. Reference Guo, Li, Fan, Li, Zhao, Huang and Xu2015). Continental mafic rocks with ocean-island basalt (OIB) affinities, which are different from typical back-arc basalts, are also found in southern Central America (Gazel et al. Reference Gazel, Hoernle, Carr, Herzberg, Saginor, Bogaard, Hauff, Feigenson and Swisher2011) and the Quaternary Andean back-arc setting in western Argentina (Hernando et al. Reference Hernando, Aragón, Frei, González and Spakman2014). Moreover, OIB-like high-pressure metabasalts occur in the Yuejinshan Complex, located along the suture zone separating the Jiamusi Massif and the Nadanhada Terrane (Bi et al. Reference Bi, Ge, Yang, Wang, Tian, Liu, Xu and Xing2017), and in the Heilongjiang Complex located between the Jiamusi–Khanka(–Bureya) and Songnen–Zhangguangcai blocks, both in NE China (Zhou et al. Reference Zhou, Wilde, Zhang and Zhao2009). The Yuejinshan and Heilongjiang complexes have been assigned, respectively, to an intraplate–seamount setting during the Late Carboniferous to middle Permian and to a rift environment, indicating that the Jiamusi Block rifted from the Songliao segment of the Central Asian Orogenic Belt (CAOB) prior to ~260 Ma. However, no Early Mesozoic OIB-like rocks have been reported as products of an extensional tectonic setting, and study of such rocks is important in elucidating the nature of deep mantle sources and hence the Mesozoic tectonic evolution of this region of Asia.
The tectonic evolution of the Xing’an Mongolian Orogenic Belt (XMOB) was controlled mainly by subduction of the palaeo-Asian oceanic slab beneath NE Asia throughout the Palaeozoic, overprinted by subduction of the palaeo-Pacific plate during the Mesozoic–Cenozoic. It is critical to confirm the timing of transition between these two tectonic regimes and their roles in the crustal development and magmatism in East Asia. Zhou et al. (Reference Zhou, Wilde, Zhang and Zhao2009, Reference Zhou, Cao, Wilde, Zhao, Zhang and Wang2014) inferred that subduction of the palaeo-Pacific plate beneath the continent began between the Triassic and Early Jurassic, whereas Xu et al. (Reference Xu, Wang, Meng, Gao, Pei, Yu and Tang2012) suggested that it was initiated instead during the Early–Middle Jurassic. Further investigation of the Early Jurassic magmatism is a key to constraining the timing of the onset of palaeo-Pacific plate subduction. Previous studies of Lower Jurassic mafic rocks focused on the Lesser Xing’an Range (Yu et al. Reference Yu, Wang, Xu, Gao and Pei2012; Fig. 1a, b, c), located in the northern part of the Zhangguangcai Range, with little attention being paid to mafic intrusions in the latter.
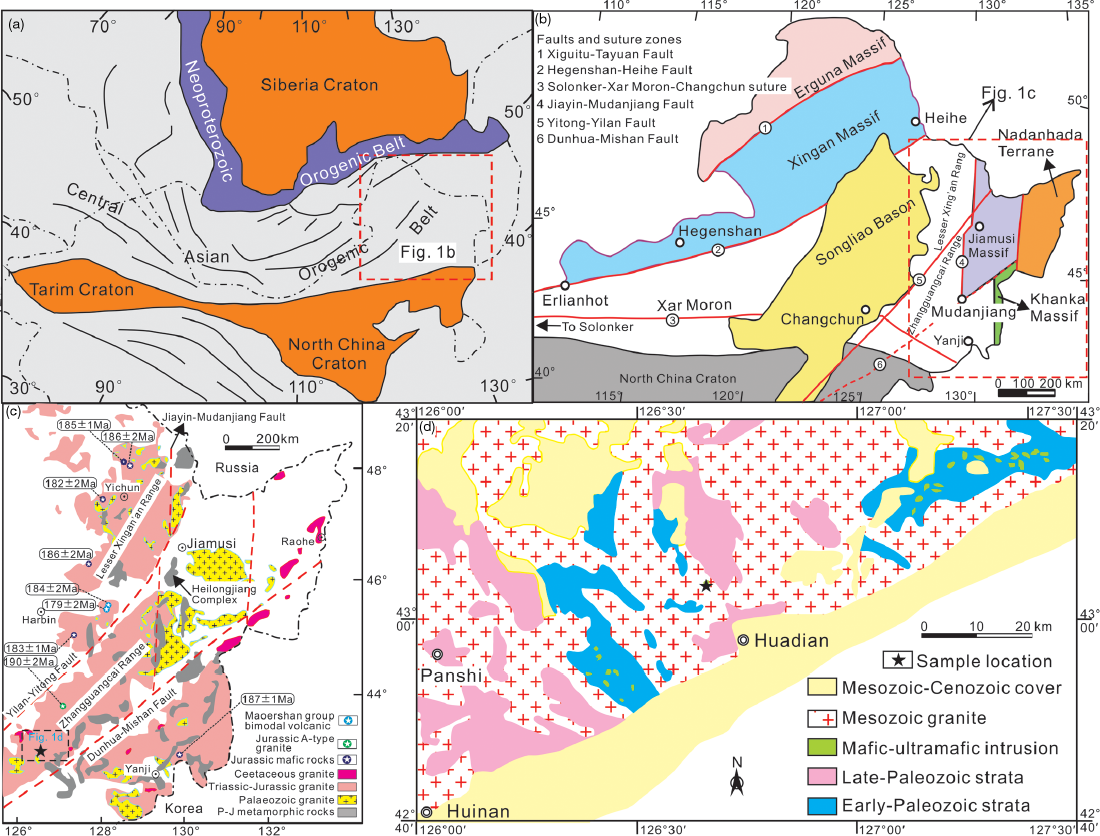
Fig. 1. Simplified tectonic maps of the Central Asian Orogenic Belt. (a) CAOB (modified after Sun et al. Reference Sun, Long, Cai, Jiang, Wang, Yuan, Zhao, Xiao and Wu2009). (b) NE China (modified after Wu et al. Reference Wu, Jahn, Wilde and Sun2000). (c) Distribution of Phanerozoic intrusions in NE China (modified after Guo et al. Reference Guo, Li, Fan, Li, Zhao, Huang and Xu2015). Data source: Jurassic mafic intrusions (Yu et al. Reference Yu, Wang, Xu, Gao and Pei2012; Guo et al. Reference Guo, Li, Fan, Li, Zhao, Huang and Xu2015); Jurassic A-type granite (Wu et al. Reference Wu, Sun, Li, Jahn and Wilde2002); the Maoershan group bimodal volcanic rocks (Tang et al. Reference Tang, Xu, Wang, Gao and Cao2011). (d) Geological sketch map of the Dabaizigou dolerite porphyrite in the Zhangguangcai Range (modified after JBGMR, 1989).
In this study we present new zircon U–Pb ages, whole-rock geochemistry and Sr–Nd–Hf isotopic compositions of a Lower Jurassic, OIB-like Dabaizigou (DBZG) porphyritic dolerite pluton in the Zhangguangcai Range. These new data and our discussion provide significant constraints for the source, origin and timing of magmatism, and help us elucidate a better understanding of the tectonomagmatic evolution of the Xing’an Mongolian Orogenic Belt, and ancient plate activities in NE Asia. Our new tectonic model provides a regionally coherent explanation for the mode and tempo of the Early Jurassic magmatism and its ESE migration through time across NE China.
2. Geological background and petrography
The XMOB is a collage of terranes that were amalgamated through the accretion of a series of micro-continental blocks during the Phanerozoic (Tang et al. Reference Tang, Wang, He and Shao1995; Li, Reference Li2006; Wu et al. Reference Wu, Yang, Lo, Wilde, Sun and Jahn2007; Zhou & Wilde, Reference Zhou and Wilde2013), including the Erguna and Xing’an massifs in the northwest, the Songnen Block in the central region, and the Jiamusi and Khanka blocks in the east. The Jiamusi Block contains two main tectonostratigraphic units, the Mashan and Heilongjiang complexes. The Mashan Complex and its deformed granitoids make up the bulk of the Jiamusi Massif. The extant data suggest a U–Pb zircon age of c. 500 Ma for its metamorphism (Wilde et al. Reference Wilde, Dorsett-Bain and Liu1997, Reference Wilde, Zhang and Wu2000; Wilde, Reference Wilde2003). The geology of the Heilongjiang Complex, which occurs close to the fault-bounded contact between the Jiamusi and Songnen blocks, is interpreted to mark the onset of the palaeo-Pacific plate subduction (Zhou et al. Reference Zhou, Wilde, Zhang and Zhao2009). The Songnen Block is separated from the Jiamusi Block by the Mudanjiang Suture, and contains the Songliao Basin in its central area, the Lesser Xing’an Range in the northeast, and the Zhangguangcai Range in the southeast (Zhou & Wilde, Reference Zhou and Wilde2013) (Fig. 1b). The DBZG porphyry dolerite pluton, which is the focus of this study, occurs in the Zhangguangcai Range, characterized by voluminous Phanerozoic granitic rocks (Wu et al. Reference Wu, Jahn, Wilde, Lo, Yui, Lin, Ge and Sun2003a, b). Exposed strata in the Zhangguangcai Range include the Silurian–Devonian Xiaosangedingzi and Huangyingtun groups, the Early Carboniferous Lujuantun Group, the middle Carboniferous Mopanshan Group, the Late Carboniferous Shizuizi Group, the Early Permian Yilaxi and Fanjiatun Groups, and the Late Permian Nuanmutiaozi Group (Fig. 1d; JBGMR, 1989). Over half of the area is covered by Phanerozoic granites that intrude into Late Palaeozoic to Late Mesozoic strata.
Type locality of the DBZG pluton is near Dabaizigou village in the Huadian district of Jilin City and is exposed over an area of ~2 km2 with a N–S orientation (Fig. 1c). The pluton comprises mainly porphyritic dolerite with an ophitic texture (Fig. 2a, b), and contains phenocrysts of euhedral–subhedral plagioclase crystals (2–3 mm long, 15–20% of the rock mass) and interstitial, anhedral or subhedral clinopyroxene (1–2 mm, 10–15%). The matrix (65–70%) includes clinopyroxene (0.05–0.1 mm, 15–20%), orthopyroxene (0.05–0.1 mm, ~5%), plagioclase (0.03–0.05 mm, 25–30%), K-feldspar (0.02–0.05 mm, 3–5%), and minor accessory minerals such as zircon, magnetite and apatite.

Fig. 2. Representative photomicrographs of the Dabazigou diabase. Pl, plagioclase; Cpx, clinopyroxene.
3. Analytical methods
3.a. Zircon U–Pb dating
Representative zircon grains were separated from the DBZG porphyritic dolerite by standard density and magnetic separation techniques at Langfang Chengxin Geological Service, Hebei Province, China. Their external and internal structures were observed microscopically under transmitted and reflected light, and by cathodoluminescence (CL) methods at the Micro-area Laboratory, Institute of Geology, Chinese Academy of Geological Sciences (IGCAGS), Beijing, China.
U–Pb dating of zircon separates was performed by laser ablation – inductively coupled plasma – mass spectrometry (LA-ICP-MS) at the Ministry of Natural Resources Key Laboratory of Metallogeny and Mineral Assessment, Institute of Mineral Resources, Chinese Academy of Geological Sciences (CAGS), Beijing, China, following the techniques described by Wu et al. (Reference Wu, Yang, Xie, Yang and Xu2006) and Hou et al. (Reference Hou, Li, Zou, Qu, Shi and Xie2007), with an ablation spot of ~32 μm in diameter.
3.b. Major- and trace-element analyses
Whole-rock major- and trace-element compositions were determined at the China National Research Center of Geoanalysis, CAGS. Sample preparation involved the trimming of altered surfaces, washing with deionized water, and crushing and powdering in an agate mill. Major elements were analysed by X-ray fluorescence (XRF) spectrometry. Analytical accuracy is estimated to be ±1% for SiO2 and ±2% for other oxides. Trace elements including REE were determined by ICP-MS. Analytical uncertainties and detailed analytical procedures are described by Qi et al. (Reference Qi, Hu and Gregoire2000). Analytical precision for trace elements was better than ±5%.
3.c. Rb–Sr and Sm–Nd isotopic analyses
Rb–Sr and Sm–Nd isotopic analyses were performed at the Centre of Modern Analysis, Nanjing University, Nanjing, China, using a VG 354 MS. Sample powders were spiked with mixed isotopic tracers before dissolution in HF–HNO3–HClO4. Analysis of NBS987 and La Jolla standards over the past 5 years has yielded mean 87Sr/86Sr and 143Nd/144Nd ratios of 0.710342 ± 0.000040 and 0.511840 ± 0.000008, respectively. Procedural blanks were <1 ng for Sr and <60 pg for Nd (Wang et al. Reference Wang, Yang, Chen, Zhang and Rao2007).
3.d. Zircon Hf isotopic analyses
Zircon Hf analyses of the grains used for U–Pb dating were performed by LA-ICP-MS (Neptune Plus and Compex pro 193nm LA system) at the Continental Tectonics and Dynamics Laboratory, IGCAGS. Ablation-spot diameter was 44 or 32 μm, depending on the zircon size. Zircon standard GJ-1 was used as a reference material. Detailed procedures are described by Hou et al. (Reference Hou, Li, Zou, Qu, Shi and Xie2007). The weighted-mean 176Hf/177Hf ratio of GJ-1 was 0.282015 ± 0.000008 (2σ, n = 10), consistent with reported values (Hou et al. Reference Hou, Li, Zou, Qu, Shi and Xie2007). Data acquisition protocols are described by Wu et al. (Reference Wu, Yang, Xie, Yang and Xu2006). The following parameters were used in calculations of ɛ Hf(t) and Hf model ages: (176Lu/177Hf)DM = 0.0384, (176Hf/177Hf)DM = 0.28325 (Griffin et al. Reference Griffin, Pearson, Belousova, Jackson, van Achterbergh, O’Reilly and Shee2000); (176Lu/177Hf)CHUR = 0.0332, (176Hf/177Hf)CHUR,0 = 0.282772 (Blichert-Toft et al. Reference Blichert-Toft, Chauvel and Albarède1997); (176Lu/177Hf)CC = 0.015 (Griffin et al. Reference Griffin, Wang, Jackson, Pearson, O’Reilly, Xu and Zhou2002); and λLu = 1.867 × 10–11 a–1 (Söderlund et al. Reference Söderlund, Patchett, Vervoort and Isachsen2004).
4. Analytical results
4.a. U–Pb zircon geochronology
Zircon grains separated from porphyritic dolerite sample DBZG-1 are subhedral to euhedral, prismatic, contain clear oscillatory zoning, and have Th/U ratios of 0.29–1.31, indicative of a magmatic origin. They yield a weighted-mean 206Pb/238U age of 187.6 ± 1.5 Ma (n = 22; MSWD = 0.65; Table 1; Fig. 3), which is interpreted as the crystallization age of the DBZG porphyritic dolerite pluton.

Fig. 3. Representative U–Pb concordia diagram for zircon grains from mafic sample DBZG-01. Inset shows the locations of ablation spots.
Table 1. LA-ICP-MS U–Pb isotopic data for zircons from the Dabazigou mafic dykes

4.b. Major- and trace-element geochemistry
Whole-rock geochemical compositions of the analysed dolerite samples are given in Tables 2 and 3. Dolerite rocks have relatively low and uniform SiO2 (46.18–48.39 wt %), Na2O (2.99–4.77 wt %) and K2O (0.41–1.33 wt %) contents, with MgO contents of 7.15–9.31 wt % and Mg numbers of 60–64. All samples plot in the alkaline-basalt field in total-alkalis v. SiO2 (TAS), and Zr/TiO2 v. Nb/Y diagrams (Fig. 4), unlike the Lower Jurassic calc-alkaline basalts of the Zhangguangcai Range.

Fig. 4. Geochemical classification of the DBZG mafic rocks. (a) SiO2 v. (Na2O + K2O) and (b) Nb/Y v. Zr/TiO2 (×10–4) (Winchester & Floyd, Reference Winchester and Floyd1977).
Table 2. Major (%) element data for the Dabazigou mafic dykes

Note: LOI: Loss on ignition; Mg# = 100 × Mg2 ± (Mg2+ + Fe2+).
Table 3. Trace element (ppm) data for the Dabazigou mafic dykes

The dolerites are characterized by smooth chondrite-normalized REE patterns, displaying moderate LREE enrichment similar to OIB, with a narrow range of Eu/Eu* (0.70–1.03) and (La/Yb)N (5.67–6.52) values (Table 3; Fig. 5a). The primitive-mantle-normalized trace-element diagram (Fig. 5b) indicates weak Nb–Ta enrichment and no obvious Zr, Hf or Ti depletion, similar to but lower values than average OIB. However, these obtained values are significantly higher than those of subduction-zone basalts. The analysed samples have Nb/U ratios of 31–37, similar to those of average OIB (47 ± 10; Hofmann et al. Reference Hofmann, Jochum and Seufert1986).

Fig. 5. (a) Chondrite-normalized REE patterns, and (b) primitive-mantle-normalized trace-element spider diagrams for the DBZG diabase porphyrite. Normalizing values for chondrite and primitive mantle, and OIB, N-MORB and E-MORB values are from Sun & McDonough (Reference Sun, McDonough, Saunders and Norry1989). Other data sources are: Middle Okinawa Trough (Shinjo et al. Reference Shinjo, Chung, Kato and Kimura1999); Andean back-arc basalt (Hernando et al. Reference Hernando, Aragón, Frei, González and Spakman2014); subduction-zone basalt, with the lower and upper limits defined by ‘average’ low-K and high-K basalts, respectively (Tatsumi & Eggins, Reference Tatsumi and Eggins1995; Xia, Reference Xia2014).
4.c. Rb–Sr and Sm–Nd isotopic compositions
DBZG porphyritic dolerite samples have (87Sr/86Sr)i values of 0.7033–0.7044 and relatively uniform positive ɛ Nd(t) values (2.3–3.2; Table 4; Fig. 6a), suggesting that their magmas had a depleted-mantle source. They have yielded a corresponding single-stage Nd model age (TDM) of 0.75–0.92 Ga.

Fig. 6. (a) Sr–Nd isotopic diagram, and (b) ɛ Hf(t) v. U–Pb zircon age diagram for the Dabaizigou mafic intrusion in NE China. Data sources: MORB, OIB and mantle array (Zhang et al. Reference Zhang, Sun, Zhou and Ying2005); I-type granitoids (Li et al. Reference Li, Guo, Li, Li and Zhao2014; Guo et al. Reference Guo, Li, Fan, Li, Zhao, Huang and Xu2015); Mashan Group (Wu et al. Reference Wu, Jahn, Wilde and Sun2000); Early Jurassic mafic intrusive rocks in the Yanji area (Guo et al. Reference Guo, Li, Fan, Li, Zhao, Huang and Xu2015); Permian mafic intrusive rocks in the Yanji area (Guo et al. Reference Guo, Li, Fan, Li, Zhao and Huang2016); Early Permian olivine–gabbro of the Zhuangguangcai Range (Feng et al. Reference Feng, Liu, Niu and Yang2018); Early Jurassic mafic rocks of the Lesser Xing’an Range (Yu et al. Reference Yu, Wang, Xu, Gao and Pei2012). CAOB, Central Asian Orogenic Belt; YFTB, Yanshan Fold-and-Thrust Belt (Yang et al. Reference Yang, Wu, Shao, Wilde, Xie and Liu2006).
Table 4. Sr–Nd isotopic ratios for the representative Dabazigou mafic dykes and Zhongxiantun granites

Chondrite Uniform Reservoir (CHUR) values (87Rb/86Sr = 0.0847, 87Sr/86Sr = 0.7045, 147Sm/144Nd = 0.1967, 143Nd/144Nd = 0.512638) are used for the calculation. λ Rb = 1.42 × 10−11 a−1 (Steiger & Jäger, Reference Steiger and Jäger1977); λ Sm = 6.54 × 10−12 a−1 (Lugmair & Harti, Reference Lugmair and Harti1978).
4.d. Zircon Hf isotopic composition
The DBZG porphyritic dolerite samples have 176Lu/177Hf ratios of 0.001008–0.011259, (176Hf/177Hf)i ratios of 0.282895–0.283063 and ɛHf(t) values of 8.5–17.1 (Table 5; Fig. 6b). Their single-stage Hf model ages (TDM1) range between 502 Ma and 269 Ma (discarding one analysis, spot 9, of 149 Ma).
Table 5. Zircon Hf isotope date for the Dabazigou mafic dykes and Zhongxiantun granites

ɛ Hf(t) = 10 000{[(176Hf/177Hf)S – (176Lu/177Hf)S × (e λt – 1)]/[(176Hf/177Hf)CHUR,0 – (176Lu/177Hf)CHUR × (e λt – 1)] –1}
T DM1 = 1/λ × ln{1 + (176Hf/177Hf)S – (176Hf/177Hf)DM]/[(176Lu/177Hf)S – (176Lu/177Hf)DM]}
ƒLu/Hf = (176Lu/177Hf)S/(176Lu/177Hf)CHUR-1
The 176Hf/177Hf and 176Lu/177Hf ratios of chondrite and depleted mantle at the present are 0.282772 and 0.0332, 0.28325 and 0.0384, respectively (Blichert-Toft & Albarède, Reference Blichert-Toft and Albarède1997; Griffin et al. Reference Griffin, Pearson, Belousova, Jackson, van Achterbergh, O’Reilly and Shee2000). λ = 1.867 × 10–11 a–1 (Söderlund et al. Reference Söderlund, Patchett, Vervoort and Isachsen2004). (176Lu/177Hf)C = 0.015, t = crystallization age of zircon.
5. Mantle melt source and melting conditions
The DBZG porphyritic dolerite pluton yields a narrow range of low SiO2 contents (46.18–48.39 wt %), indicating that its magmas were derived from an ultramafic mantle source (Liu et al. Reference Liu, Hu, Gao, Feng, Qi, Zhong, Xiao, Qi, Wang and Coulson2008). The variable MgO contents (7.15–9.31 wt %) and Mg numbers (60–64) of the analysed samples are lower than those of primitive magmas, pointing to the important role of fractional crystallization during its magmatic evolution. Zr shows negative correlations with Al2O3, Fe2O3, MgO, Ni and Cr (Fig. 7a, b, c, g, h), suggesting that K-feldspar and ferromagnesian minerals such as olivine and clinopyroxene were the major fractionating phases. In contrast, the fractionation of plagioclase and accessory minerals such as apatite and Fe–Ti oxides appears to have been less significant in view of the positive correlations between Zr and P2O5, TiO2 and Sr (Fig. 7d, e, f), and also the lack of strong Eu anomalies (Eu/Eu* = 0.70–1.03). The observed positive correlation between V and TiO2 indicates hornblende fractionation (Fig. 7i; Guo et al. Reference Guo, Li, Fan, Li, Zhao and Huang2016).

Fig. 7. Zr v. Al2O3, Fe2O3, MgO, P2O5, TiO2, Sr, Ni, Cr and V diagrams for the Early Jurassic mafic intrusion in the Zhangguangcai Range, NE China.
Mantle-derived magmas generally assimilate crustal materials during their ascent or storage in a crustal magma chamber (Altunkaynak et al. Reference Altunkaynak, Dilek, Genç, Sunal, Gertisser, Furnes, Foland and Yang2012; Zhang et al. Reference Zhang, Yuan, Long, Sun, Huang, Du and Wang2016). Palaeozoic granitoids in the Songnen–Zhangguagncai Range show negative ɛ Nd(t) values and highly radiogenic Sr isotopic compositions (Wu et al. Reference Wu, Jahn, Wilde and Sun2000), and the Mashan Group, which may represent the lower-crustal protolith adjacent to the Zhangguangcai Range, shows evolved Sr–Nd isotopic compositions (Fig. 6a; Wu et al. Reference Wu, Jahn, Wilde and Sun2000). Crustal contamination can lead to mafic magmas with enriched Sr–Nd isotopic compositions and cause significant Nb–Ta depletion in basaltic rocks (Rudnick & Fountain, Reference Rudnick and Fountain1995; Feng et al. Reference Feng, Liu, Zhong, Feng, Coulson, Qi, Yang and Yang2012, Reference Feng, Liu, Feng, Yang, Yang, Tang and Yang2015). The range of (87Sr/86Sr)i values (0.7033–0.7044) of the DBZG mafic rocks implies a contribution from crustal sources; however, their relatively homogeneous Nd isotopic compositions and lack of correlation between MgO contents and ɛ Nd(t) values (not shown) are inconsistent with the effects of significant degrees of crustal contamination. Furthermore, the weak Zr–Hf depletion, weak enrichment in Ta, and uniform Nb/La (0.99–1.18) and Nb/Th (8.96–10.25) ratios, irrespective of Mg numbers, also exclude the possibility of significant crustal contamination. Therefore, we have further scrutinized our rock samples to better constrain the nature of their melt source.
5.a. Mantle melt source
The Lower Jurassic DBZG mafic rocks have relatively uniform Nd and Hf isotopic compositions with ɛ Nd(t) and ɛ Hf(t) values of 2.3–3.2 and 8.5–17.1, respectively. On a Sr–Nd isotopic diagram and a ɛ Hf(t) v. U–Pb zircon age diagram, all the analysed rocks plot above the mantle array (Vervoort et al. Reference Vervoort, Patchett, Blichert-Toft and Albarède1999) and east-CAOB fields (Fig. 6a, b), and slightly above the Lower Permian olivine–gabbro from the Zhangguangcai Range and the Lower Jurassic mafic rocks of the Lesser Xing’an Range (Yu et al. Reference Yu, Wang, Xu, Gao and Pei2012; Feng et al. Reference Feng, Liu, Niu and Yang2018). These geochemical features indicate that magmas of the Lower Jurassic DBZG dolerite pluton originated from partial melting of an isotopically depleted mantle source. However, in a primitive-mantle-normalized trace-element diagram (Fig. 5b) the HFSE patterns are higher than those of subduction-zone basalts and mafic rocks of the Lesser Xing’an and Zhangguangcai ranges, but similar to those of OIB (Fig. 5b). In a Th/Yb v. Nb/Yb diagram (Fig. 8f), mafic rocks metasomatized by melts and fluids derived from slab dehydration or subducted sediments plot in the oceanic-arc and continental-arc fields. However, all of the DBZG samples plot along the mid-ocean-ridge basalt (MORB)–OIB array, consistent with their magmas having been derived from a geochemically enriched mantle in an intraplate setting, and with the mantle source being largely unaffected by subduction-related fluids and/or melts (Bi et al. Reference Bi, Ge, Yang, Wang, Tian, Liu, Xu and Xing2017). This result is also compatible with the high Zr/Y ratios and tectonic discrimination diagrams (Fig. 8a, b, c, d, e). Furthermore, in a La/Nb v. La/Ba diagram (not shown), the DBZG mafic rocks plot in the OIB field (Hernando et al. Reference Hernando, Aragón, Frei, González and Spakman2014) based on over 900 analyses of OIB from various ocean islands (Fitton et al. Reference Fitton, James and Leeman1991). It is, therefore, concluded that the magma source of the DBZG mafic rocks was significantly different from that of other Early Jurassic mafic intrusions in the Lesser Xing’an Range that exhibit arc-like trace-element patterns. The latter may have been derived from partial melting of a depleted lithospheric mantle that was metasomatized by slab-derived fluids in a back-arc setting. The DBZG mafic rocks have, on the other hand, a geochemical signature more similar to that of alkaline OIB and alkaline continental basalts.

Fig. 8. Tectonic discrimination diagrams for the DBZG mafic rocks. (a) Ti/100 v. Zr v. 3Y diagram (Pearce & Cann, Reference Pearce and Cann1973); (b) 2Nb v. Zr/4 v. Y diagram (Meschede, Reference Meschede1986); (c) Hf/3 v. Th v. Ta diagram (Wood, Reference Wood1980); (d) Ti/1000 v. V diagram (Pearce, Reference Pearce2014); (e) Zr v. Zr/Y diagram (Pearce & Norry, Reference Pearce and Norry1979); (f) Nb/Yb v. Th/Yb diagram (Dilek & Furnes, Reference Dilek and Furnes2011; Pearce, Reference Pearce2014).
Two main mantle sources have been proposed for alkaline OIB, namely a metasomatized lithospheric mantle (Niu & O’Hara, Reference Niu and O’Hara2003; Pilet et al. Reference Pilet, Hernandez, Sylvester and Poujol2005, Reference Pilet, Baker and Stolper2008, Reference Pilet, Baker, Müntener and Stolper2011) and the asthenosphere (Fitton et al. Reference Fitton, James and Leeman1991; Farmer, Reference Farmer, Holland and Turekian2003). Pilet et al. (Reference Pilet, Baker, Müntener and Stolper2011) proposed that the origin of alkaline lavas may be related to lithospheric metasomatism, and further suggested that amphibole-bearing veins in a lithospheric mantle may be important components of the source of alkaline OIB and continental basalts (Hernando et al. Reference Hernando, Aragón, Frei, González and Spakman2014). Amphibole-rich veins are interpreted as the cumulates produced by low-degree melting of the underlying asthenosphere during its ascent (Pilet et al. Reference Pilet, Baker, Müntener and Stolper2011). In a modelling study, Pilet et al. (Reference Pilet, Baker, Müntener and Stolper2011) reported that the geochemical signatures of such veins include positive Nb anomalies relative to U and LREEs (due to the compatibility of Nb in amphibole), and low HREE contents and high (La/Yb)N ratios (due to a garnet-bearing asthenospheric source of metasomatizing fluids). However, we note that these signatures are absent among the geochemical treats of the DBZG mafic rocks, just like the post-caldera basalts of the Payún Matrú volcanic field (PMVF) in the Andean back-arc of western Argentina (Hernando et al. Reference Hernando, Aragón, Frei, González and Spakman2014). We infer, therefore, that a metasomatized lithospheric mantle contribution was not significant in the petrogenesis of the DBZG mafic magmas. We posit, however, that an asthenospheric mantle contribution was important.
5.b. Partial melting conditions
REE and HFSE contents and ratios are not significantly affected by fluid influx or mantle depletion because they are moderately incompatible during partial melting of upper mantle peridotites, according to their partition coefficients (Pearce & Peate, Reference Pearce and Peate1995; Johnson, Reference Johnson1998; Münker, Reference Münker2000). Moreover, Y and Yb contents are buffered in primary melts by residual garnet during melting of mantle peridotites (Johnson, Reference Johnson1998). Thus, melts originating from partial melting of upper mantle peridotites in the garnet stability field display high La/Yb and Sm/Yb ratios, and low Yb contents (Liu et al. Reference Liu, Su, Hu, Feng, Gao, Coulson, Wang, Feng, Tao and Xia2010). In contrast, spinel has similar partition coefficients for Yb, Gd and Dy, with only slight fractionation during melting in the spinel stability field (Green, Reference Green2006; Zhang et al. Reference Zhang, Yuan, Long, Sun, Huang, Du and Wang2016). REE and HFSE contents are, therefore, controlled mainly by the degree of partial melting; the mantle compositions are widely used to discriminate garnet–lherzolite sources from spinel–lherzolite sources and to decipher the degree of mantle melting (e.g. Gurenko & Chaussidon, Reference Gurenko and Chaussidon1995; Münker, Reference Münker2000; Green, Reference Green2006; Zhao & Zhou, Reference Zhao and Zhou2009). In the La v. La/Sm and La/Yb v. Yb diagrams (Fig. 9a, b), the DBZG mafic samples plot in a transition zone between spinel–lherzolite and garnet–lherzolite melting curves, indicating the derivation of their magmas from partial melting of a deeper mantle source in the garnet-facies stability zone and continued melting in the shallower spinel-facies zone, as part of a parabolic melt system with ~10% partial melting (Khogenkumar et al. Reference Khogenkumar, Singh, Bikramaditya Singh, Khanna, Singh and Singh2016). Applying the melting column concept (Plank & Langmuir, Reference Plank and Langmuir2012) at the depth of the spinel–garnet transition for the peridotite solidus at 75–80 km (McKenzie & O’Nions, Reference McKenzie and O’Nions1991; Robinson & Wood, Reference Robinson and Wood1998), we know that in an extensional setting upwelling asthenosphere rises to a relatively shallow level of <80 km, whereby it undergoes decompression melting.

Fig. 9. (a) La v. La/Sm diagram showing melt curves and lines obtained using the non-modal batch melting model (Aldanmaz et al. Reference Aldanmaz, Yaliniz, Guçtekin and Goncuoglu2008). The parameters and common geochemical reservoirs used in the diagram are as described in Aldanmaz et al. (Reference Aldanmaz, Pearce, Thirlwall and Mitchell2000). The enriched mantle (EM) is assumed to have incompatible element concentrations similar to that proposed in Aldanmaz & Köprübaşi (Reference Aldanmaz and Köprübaşi2006). The mantle array is defined using melt-residual compositional trends from depleted MORB mantle (DMM) and primitive mantle (PM; similar in composition to CI chondrite) compositions. Mantle depletion and enrichment trends are defined by melt extraction from the mantle (towards the residues) and melt addition to the mantle (or influx of material from outside the mantle prior to solid-state mixing and homogenization) respectively. The straight-line array represents mixing between melts from enriched (EM) and depleted mantle (DMM) components, marked by curved lines. (b) Yb v. La/Yb diagram for mafic volcanics of the DBZG mafic rocks. Melt curves are point-average, non-modal, fractional melts of garnet and spinel lherzolites (garnet lherzolite: 0.598 ol, 0.211 opx, 0.076 cpx, 0.115 gt that melts in the proportions 0.05 ol, 0.20 opx, 0.30 cpx, 0.45 gt; spinel lherzolite: 0.578 ol, 0.270 opx, 0.119 cpx, 0.033 sp that melts in the proportions 0.10 ol, 0.27 opx, 0.50 cpx, 0.13 sp (Thirlwall et al. Reference Thirlwall, Upton and Jenkins1994; Baker et al. Reference Baker, Menzies, Thirlwall and MacPherson1997)), and the scale melting fraction is from Rotolo et al. (Reference Rotolo, Castorina, Cellura and Pompilio2006). Distribution coefficients were taken from McKenzie & O’Nions (Reference McKenzie and O’Nions1991). The MORB and OIB fields from Baker et al. (Reference Baker, Menzies, Thirlwall and MacPherson1997) and El-Rahman et al. (Reference El-Rahman, Polat, Dilek, Fryer, El-Sharkawy and Sakran2009) are shown for comparison.
6. Tectonic implications and model
6.a. Possible tectonic settings of OIB-type magmatism
Two main tectonic scenarios have been proposed for the genesis of OIB-type mafic rocks: (i) the ascent of a mantle plume (Burke & Wilson, Reference Burke and Wilson1976; Richards et al. Reference Richards, Duncan and Courtillot1989; Griffiths & Campbell, Reference Griffiths and Campbell1991), such as those OIB alkaline basalts observed in the early and late phases of Hawaiian eruptions (Frey et al. Reference Frey, Wise, Garcia, West, Kwon and Kennedy1990; Moore & Clague, Reference Moore and Clague1992; Borgia & Treves, Reference Borgia, Treves, Parson, Murton and Browning1992; Wanless et al. Reference Wanless, Garcia, Rhodes, Weis and Norman2006) and in some ophiolitic melanges (i.e. Tankut et al. Reference Tankut, Dilek and Onen1998; Sarifakioglu et al. Reference Sarifakioglu, Dilek and Sevin2017); and (ii) tectonic extension of continental lithosphere (McKenzie & Bickle, Reference McKenzie and Bickle1988; Xu, Reference Xu2007), including lithospheric extension and thinning of orogenic crust during a post-collision stage or in a back-arc setting (Dilek & Whitney, Reference Dilek and Whitney2000; Kadioglu et al. Reference Kadioglu, Dilek, Foland, Dilek and Pavlides2006). The former mechanism has been invoked to explain the origin of Mesozoic–Cenozoic alkaline basalts in eastern China (Fan & Hooper, Reference Fan and Hooper1991; Tang et al. Reference Tang, Zhang and Ying2007; Zhang et al. Reference Zhang, Zheng and Zhao2009). A typical case of the latter mechanism is the origin of back-arc basin basalts in the Andes (Hernando et al. Reference Hernando, Aragón, Frei, González and Spakman2014). The ascent of a mantle plume would generally result in large-scale and intensive mafic magmatism over a short period of time; consequently, this mechanism is not favoured for the present study area. A post-collision environment would produce intense magmatism of diverse compositions, including basaltic magmatism displaying within-plate basalt geochemical affinity, bimodal magmatism and A2-type felsic magmatism (von Blanckenburg & Davies, Reference von Blanckenburg and Davies1995; Luca, Reference Luca2004; Dilek & Altunkaynak, Reference Dilek and Altunkaynak2007; Dilek et al. Reference Dilek, Imamverdiyev and Altunkaynak2010), similar to that observed in the present study area, where the Maoershan Group bimodal magmatism (Tang et al. Reference Tang, Xu, Wang, Gao and Cao2011) occurred along with A-type granitoids (Wu et al. Reference Wu, Sun, Li, Jahn and Wilde2002) during the Early Jurassic. However, this magmatic assemblage may also form in a back-arc setting. The intrusion of the DBZG mafic rocks occurred 30–40 Ma later than the onset of final amalgamation between the Siberia and North China Cratons (NCC) during the Late Permian or Early Triassic (Zhou et al. Reference Zhou, Wilde, Zhang and Zhao2009; Wang et al. Reference Wang, Xu, Xu, Gao and Ge2015). We, therefore, propose that emplacement of the Dabaizhgou dolerite pluton was related to subduction of the palaeo-Pacific ocean plate beneath East Asia, rather than the Siberia–NCC collision event.
6.b. New tectonic model
We present a new tectonic model here based on our geochemical and geochronological data and interpretations from the Dabaizhgou pluton, combined with the extant data from other magmatic and metamorphic rock assemblages in the Songnen–Zhuangguagncai mountain range in NE China (Fig. 10). The following observations and boundary conditions are used in the construction of this model:
-
(a) There is a well–developed, NNE-trending magmatic belt with both plutonic and volcanic suites, which show progressive younging in age from the west (201–198 Ma) to the east (186–182 Ma). This eastward-younging, Early Jurassic magmatic system has been widely interpreted as the manifestation of the WNW–directed subduction of the palaeo-Pacific Plate beneath the Asian continent (Wu et al. Reference Wu, Yang, Lo, Wilde, Sun and Jahn2007; Yu et al. Reference Yu, Wang, Xu, Gao and Pei2012; Zhou et al. Reference Zhou, Cao, Wilde, Zhao, Zhang and Wang2014; Qin et al. Reference Qin, Lai, Li, Ju, Zhu and Zhao2016; Xu et al. Reference Xu, Zhang, Shi, Brix, Huhma, Chen, Zhang and Zhou2017; Tang et al. Reference Tang, Xu, Wang and Ge2018; Zheng & Dai, Reference Zheng and Dai2018).
-
(b) The Early Jurassic plutonic rocks display significant changes in their geochemical characteristics and isotopic fingerprints. The relatively oldest (201–198 Ma) monzogranite intrusions in the west have high SiO2, K2O and Rb contents, depleted whole-rock Sr–Nd–Pb isotopic compositions, and zircons with depleted Lu–Hf isotopic values, reminiscent of adakite-like melts (Qin et al. Reference Qin, Lai, Li, Ju, Zhu and Zhao2016). Their magmas are considered to have been derived from partial melting of subducted melanges at the subducting plate interface dipping at shallow angles to the WNW (Fig. 10). The younger (186–182 Ma) mafic to felsic plutons in the east have calc-alkaline affinities with LILE enrichment and HFSE depletion patterns, characteristic of island-arc rocks; their magmas were produced by partial melting of a subduction-metasomatized mantle wedge above a steeply dipping palaeo-Pacific slab (Yu et al. Reference Yu, Wang, Xu, Gao and Pei2012). Similarly, the Early Jurassic calc-alkaline volcanic rocks in the Dongning–Wangqing–Hunchun region (Xu et al. Reference Xu, Ge, Pei, Meng, Yu and Yang2008), east of the Dunhua–Mishan fault (eastern Heilongjiang–Jilin Provinces), represent the products of island-arc magmatism that formed above this WNW-dipping subduction zone (Tang et al. Reference Tang, Xu, Wang and Ge2018).
-
(c) From the onset of the subduction of the palaeo-Pacific plate beneath the Asian continent in the latest Triassic – Early Jurassic (~201–198 Ma) and onwards the subducting slab experienced rollback and steepening through time (Deng et al. Reference Deng, Liu, Wang, Dilek and Liang2017; Tang et al. Reference Tang, Xu, Wang and Ge2018). This slab rollback and higher angles of subduction caused lithospheric thinning in the upper plate and produced an extended island-arc – back-arc system (Fig. 10).
-
(d) Rapid slab retreat in the Early Jurassic (from ~200 Ma to 180 Ma) induced mantle return flow and asthenospheric upwelling into the extended upper mantle to fill the slab-free region created by the eastward migration of the slab (Fig. 10; Liu et al. Reference Liu, Levander, Zhai, Porritt and Allen2012). This asthenospheric upwelling led to the partial melting of a fertile lherzolitic source in the garnet–spinel stability field (Fig. 10) and to the production of OIB-like melts, which formed the magmas of the DBZG and other mafic plutons. Heat and melt flux driven by the rising asthenosphere further facilitated the lithospheric extension in the upper continental plate due to thermal weakening.
-
(e) There is an exhumed, latest Triassic – Early Jurassic accretionary prism complex to the east of the 186–182 Ma island-arc rocks that is interpreted to have formed and evolved with the eastward-migrating palaeo-Pacific plate subduction (Wu et al. Reference Wu, Yang, Lo, Wilde, Sun and Jahn2007; Zhou et al. Reference Zhou, Cao, Wilde, Zhao, Zhang and Wang2014). The Heilongjian metamorphic complex in the Jiamusi massif and the Nadanhada terrane to the east (Fig. 1) represent this Early Jurassic accretionary prism complex.

Fig. 10. An interpretive tectonic model for the development of the Dabaizigou pluton in the Zhangguangcai mountain range in NE China from OIB-type melts originated from partial melting of the asthenospheric mantle. Rollback of the WNW-subducting palaeo-Pacific slab towards the east led to its steepening in time, which in turn triggered mantle corner flow and asthenospheric uprising. Collectively, slab rollback and asthenospheric heat flux caused lithospheric scale extension in the continental upper plate.
Our model depicts an incipient continental back-arc tectonic setting for the magmatic development of the DBZG mafic pluton. However, the DBZG mafic rocks are characterized by OIB-type geochemistry, which is different from typical oceanic back-arc basalts with geochemical features characteristic of both MORB and island-arc volcanic basalts (e.g. Gribble et al. Reference Gribble, Stern, Bloomer, Stüben, O’Hearn and Newman1996; Ma et al. Reference Ma, Cao, Zhou and Zhu2015). Nevertheless, the Quaternary pre- and post-caldera basaltic rocks of the Payún Matrú volcanic field (PMVF) in the back-arc setting of the Southern Andes in western Argentina show geochemical similarities to both the DBZG mafic rocks and OIBs (Hernando et al. Reference Hernando, Aragón, Frei, González and Spakman2014). In tectonic discrimination diagrams (Fig. 8), both the DBZG mafic rocks and the PMVF basalts plot in the within-plate alkaline basalt field, although the PMVF samples display the effects of moderate crustal contamination. Similarly, basalts from the middle Okinawa Trough (west of the Ryukyu Islands), which represents an incipient intracontinental back-arc basin, also display E-MORB-type (or OIB-like) geochemical features (Shinjo et al. Reference Shinjo, Chung, Kato and Kimura1999). Thus, our proposed incipient continental back-arc tectonic setting for the Early Jurassic mafic magmatism in NE China is analogous to some of the well-documented cases of OIB-type volcanism and extensional tectonics in other modern continental back-arc environments.
7. Conclusions
Our focused study of the whole-rock geochronological, geochemical and Sr–Nd–Hf isotopic features of the mafic Dabaizhgou pluton in NE China has led us to the following conclusions:
-
(1) The crystallization age of the DBZG pluton is 187 Ma (Early Jurassic), which marks a short period of mafic magmatism in the region. Geochemically, the plutonic rocks have OIB-type magma source and display high trace-element contents and Nb–Ta enrichment. They represent within-plate alkali basalt affinity, with no subduction influence or major crustal contamination effects.
-
(2) Magmas of the DBZG pluton were derived from partial melting of the upwelling asthenospheric mantle, which was driven by eastward rollback of the palaeo-Pacific slab. Decompression melting of the rising asthenospheric mantle lherzolites initially occurred in the garnet stability field (>75–80 km) and then continued upward in the spinel stability field (<75 km). The resultant alkali basaltic melts were transported into upper crustal depths along extensional fault systems, and hence avoided long residence times in the crust and crustal contamination.
-
(3) The heat and melt flux associated with this asthenospheric uprising in the Early Jurassic thermally weakened the continental lithosphere of the North China Craton and facilitated broad back-arc extension in an intracontinental setting behind an eastward-propagating island-arc magmatism in NE China.
-
(4) The onset of the palaeo-Pacific oceanic plate subduction beneath the eastern margin of continental Asia in the latest Triassic – Early Jurassic and the changing slab geometry from flat to steeply dipping subduction zone geometry controlled the mode, tempo and geochemical character of the magmatism in the continental upper plate. The DBZG pluton represents a critical period of this magmatism, during which slab rollback induced corner flow and asthenospheric upwelling triggered partial melting deeper in the upper mantle, outside of the mantle wedge of the palaeo-Pacific subduction zone.
Author ORCIDs
Yildirim Dilek 0000-0003-2387-9575
Acknowledgements
The research was funded by grants from the National Natural Science Foundation of China (41773029; 41672063; 41573022; 41720104009; 41373029), the Geological Survey (DD20160023-01) and the Foundation of MLR (201511022). We thank Cailai Wu, Zheng Wang, Min Lei, Bin Shi and Xiangzhen Xu for their assistance with Lu–Hf isotopic analysis and Zircon CL methods. We thank Professor Yujiro Ogawa and editor Susie Bloor for their editorial help and consideration, and two anonymous reviewers for their constructive reviews. Y. Dilek gratefully acknowledges the Lishiguang Scholarship Funding through the Chinese Academy of Geological Sciences in Beijing.