1. Introduction
Central East Greenland was the site of massive outpourings of tholeiitic flood basalt during the Early Palaeogene period as exemplified by the c. 7-km-thick Blosseville Kyst lava succession (Fig. 1) emplaced between 61 and 55 Ma (Storey, Duncan & Swisher, Reference Storey, Duncan and Swisher III2007). The extensive magmatism has generally been attributed to large degrees of mantle decompression melting, driven by both the impact of the Iceland mantle plume beneath the Greenland lithosphere and subsequent continental break-up and opening of the North Atlantic Ocean at 56–54 Ma (e.g. Saunders et al. Reference Saunders, Fitton, Kerr, Norry, Kent, Coffin and Mahoney1997; Storey, Duncan & Tegner, Reference Storey, Duncan and Tegner2007). Although the North Atlantic track of the hotspot is tightly constrained by the Greenland–Iceland Ridge, a c. 300-km-wide section of anomalously thick oceanic crust immediately seawards of the East Greenland rifted margin (Fig. 1), the path of the hotspot beneath Greenland and the timing of the track are still debated. Early models suggested that the mantle plume was centred directly under the ensuing rift during continental break-up (Brooks, Reference Brooks1973; White & McKenzie, Reference White and McKenzie1989; Saunders et al. Reference Saunders, Fitton, Kerr, Norry, Kent, Coffin and Mahoney1997). Plate-kinematic reconstructions, however, indicate that the plume axis was located under central Greenland during break-up and that the rifted margin first crossed the plume axis sometime after break-up (Duncan & Richards, Reference Duncan and Richards1991; Lawver & Müller, Reference Lawver and Müller1994; Torsvik et al. Reference Torsvik, Mosar and Eide2001). Bernstein et al. (Reference Bernstein, Kelemen, Tegner, Kurz, Blusztajn and Brooks1998) and Tegner et al. (Reference Tegner, Duncan, Bernstein, Brooks, Bird and Storey1998) argue that abundant post-break-up tholeiitic and alkaline magmatism at 50–47 Ma records this crossing. The magmatism during this period appears to have been confined to a c. 600 km broad region along the East Greenland coast between 66° and 70°N, with the strongest geochemical plume signatures occurring in a c. 150-km-broad zone centred near the Kangerlussuaq Fjord (Fig. 1). This is in good agreement with the width of the Greenland–Iceland Ridge (Bernstein et al. Reference Bernstein, Kelemen, Tegner, Kurz, Blusztajn and Brooks1998; Tegner et al. Reference Tegner, Duncan, Bernstein, Brooks, Bird and Storey1998).
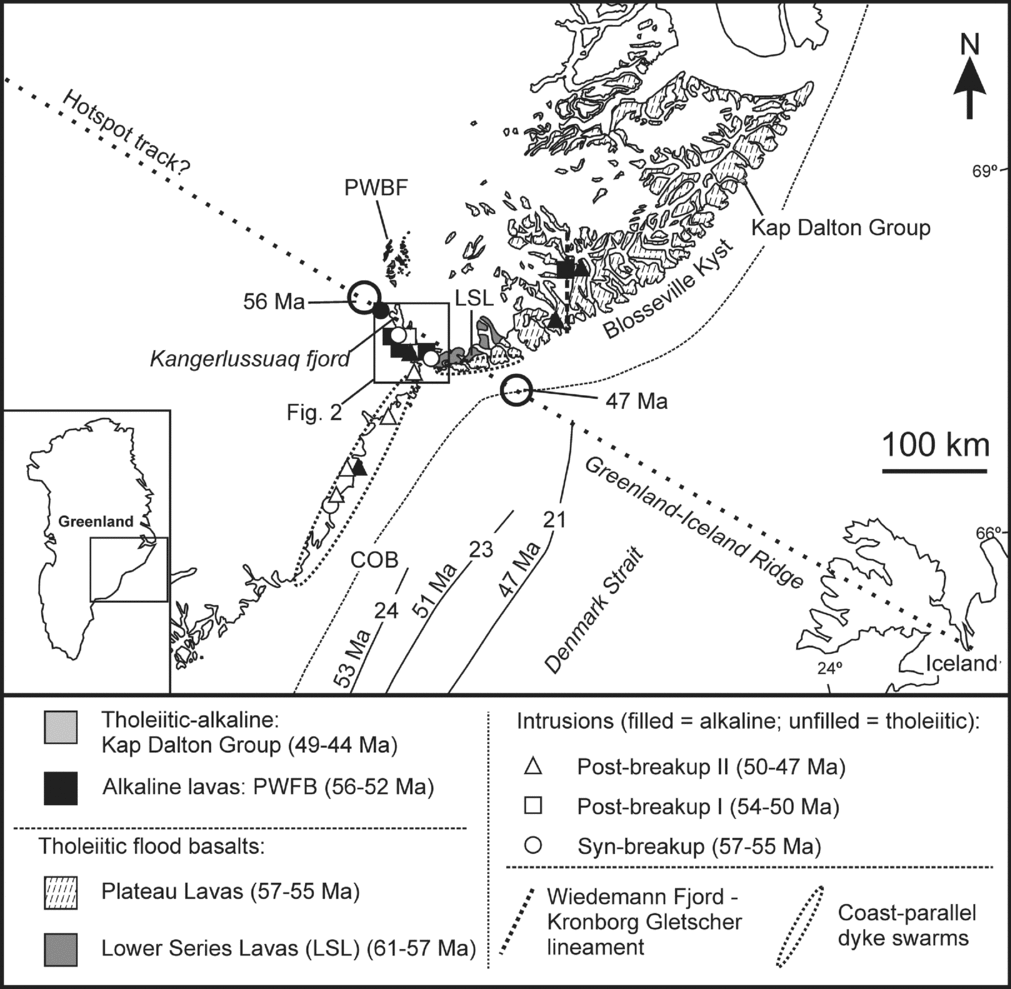
Figure 1. Simplified geological map of central East Greenland showing distribution of Palaeogene flood basalts and locations of major tholeiitic and alkaline intrusions arranged according to age. Only intrusions for which reliable radiometric data exist are shown. Post-47 Ma intrusions have been omitted for the sake of clarity. Base map modified after Myers, Dawes & Nielsen (Reference Myers, Dawes and Nielsen1988) and Hansen et al. (Reference Hansen, Pedersen, Duncan, Bird, Brooks, Fawcett, Gittins, Gorton, O’Day, Jolley and Bell2002). Age and compositional data from Nielsen (Reference Nielsen, Fitton and Upton1987), Tegner et al. (Reference Tegner, Duncan, Bernstein, Brooks, Bird and Storey1998, Reference Tegner, Brooks, Duncan, Heister and Bernstein2008), Peate et al. (Reference Peate, Baker, Blichert-Toft, Hilton, Storey, Kent, Brooks, Hansen, Pedersen and Duncan2003), Storey, Duncan & Tegner (Reference Storey, Duncan and Swisher III2007) and Larsen et al. (Reference Larsen, Pedersen, Sørensen, Watt and Duncan2013). Magnetic seafloor anomalies from Larsen (Reference Larsen and Sweeney1990) illustrate location of spreading axis at 53, 51 and 47 Ma. Location of coast-parallel dyke swarms after Myers (Reference Myers1980) and Klausen & Larsen (Reference Klausen, Larsen, Menzies, Klemperer, Ebinger and Baker2002). Dotted line shows possible hotspot track (modified from from Lawver & Müller, Reference Lawver and Müller1994) drawn to fit the Greenland–Iceland Ridge and the present-day position of the Iceland mantle plume beneath central Iceland. Proposed locations of the mantle plume axis at 56 and 47 Ma are shown by circles. COB – continent-ocean boundary; PWBF – Prinsen af Wales Bjerge Formation.
The Kangerlussuaq Fjord is a major tectonic lineament that has been interpreted as the failed arm of a triple junction. Flood basalt volcanism and intrusion of dyke swarms, followed by active seafloor spreading, occurred in two arms parallel to the present-day coastline (Brooks, Reference Brooks1973; Burke & Dewey, Reference Burke and Dewey1973; Karson & Brooks, Reference Karson, Brooks, MacNiocaill and Ryan1999). The third arm, now occupied by the Kangerlussuaq Fjord, initially developed a rift-like structure accompanied by intrusion of tholeiitic dykes, but the rifting was aborted before it reached the oceanic stage (Brooks & Platt, Reference Brooks and Platt1975; Nielsen, Reference Nielsen, Fitton and Upton1987). The fjord has many of the characteristics of continental rift valleys and hosts more than twenty exposed intrusive complexes of both tholeiitic and alkaline affinities spanning 57 to 40 Ma. It includes one of the largest syenitic intrusions in the world, the c. 800 km2 Kangerlussuaq Syenite (Wager, Reference Wager1965; Brooks, Reference Brooks1973; Nielsen, Reference Nielsen, Fitton and Upton1987; Riishuus et al. Reference Riishuus, Peate, Tegner, Wilson and Brooks2008; Tegner et al. Reference Tegner, Brooks, Duncan, Heister and Bernstein2008). More importantly, the fjord includes intrusions emplaced between 54 and 50 Ma, that is, in the transition period between continental break-up and the proposed crossing of the rifted margin over the plume axis at 50–47 Ma. This period is otherwise characterized by a dearth of magmatic records (Holm, Heaman & Pedersen, Reference Holm, Heaman and Pedersen2006; Tegner et al. Reference Tegner, Brooks, Duncan, Heister and Bernstein2008; Holwell et al. Reference Holwell, Selby, Boyce, Gilbertson and Abraham-James2012). The Kangerlussuaq Fjord therefore plays a key role in the magmatic evolution of East Greenland and presents an important link in understanding the rift-to-drift transition over the Iceland mantle plume during the opening of the North Atlantic Ocean.
Here we present isotope dilution thermal ionization mass spectrometry (ID-TIMS) U–Pb zircon ages from monzonites, syenites and alkali granites of the Kærven Complex, an early satellite intrusion of the Kangerlussuaq Syenite. The new ages are used in combination with published ages to refine and consolidate the magmatic history of the Kangerlussuaq Fjord and to re-evaluate the temporal and spatial pattern of post-break-up magmatism in relation to the Iceland hotspot track. Note that all uncertainties in the text are quoted at the 2σ level, and all cited 40Ar/39Ar ages are recalculated relative to the age of 28.201 Ma for the Fish Canyon sanidine standard and are normalized to a 40K decay constant of 5.463×10–10 (Min et al. Reference Min, Mundil, Renne and Ludwig2000; Kuiper et al. Reference Kuiper, Deino, Hilgen, Krijgsman, Renne and Wijbrans2008).
2. Geological background and previous geochronology
2.a. The Kangerlussuaq Fjord
The Kangerlussuaq Fjord is c. 80 km long and trends in a SSE-direction from the head of the fjord at c. 68°38′N and 32°34′W (Fig. 2). Palaeogene intrusions are abundant along the fjord in addition to several generations of dyke swarms (e.g. Brooks & Platt, Reference Brooks and Platt1975; Nielsen, Reference Nielsen1978). Most of the intrusions were emplaced at, or close to, the unconformity between Archaean basement and overlying Palaeogene flood basalts (Wager, Reference Wager1965; Nielsen, Reference Nielsen, Fitton and Upton1987). The alkaline Gardiner Complex is the northernmost intrusion (Fig. 2) and is dominated by ultramafic cumulates in addition to strongly undersaturated melilitolites, ijolites and carbonatites (Nielsen, Reference Nielsen, Fitton and Upton1987; Brooks, Reference Brooks2011). 40Ar/39Ar and Rb–Sr dating indicate emplacement between 56.9±0.3 and 55.1±1.2 Ma (Waight, Baker & Willigers, Reference Waight, Baker and Willigers2002; Tegner et al. Reference Tegner, Brooks, Duncan, Heister and Bernstein2008). An up to 150-m-thick sequence of alkaline lavas, formalized as the Prinsen af Wales Bjerge Formation and ranging in composition from nephelinite to trachybasalt, is exposed on nunataks 30–60 km further inland (Fig. 1; Hansen et al. Reference Hansen, Pedersen, Duncan, Bird, Brooks, Fawcett, Gittins, Gorton, O’Day, Jolley and Bell2002; Peate et al. Reference Peate, Baker, Blichert-Toft, Hilton, Storey, Kent, Brooks, Hansen, Pedersen and Duncan2003). The Prinsen af Wales Bjerge Formation is interbedded with the upper parts of the main sequence of break-up-related tholeiitic flood basalts exposed along the Blosseville coast, hereafter referred to collectively as the Plateau Lavas (Storey et al. Reference Storey, Duncan and Swisher III2007). 40Ar/39Ar dating indicates extrusion of the Prinsen af Wales Bjerge Formation between 55.2±1.0 and 53.4±1.2 Ma (Peate et al. Reference Peate, Baker, Blichert-Toft, Hilton, Storey, Kent, Brooks, Hansen, Pedersen and Duncan2003). The Kangerlussuaq Alkaline Complex (KAC) crops out 40–60 km SSE of the Gardiner Complex on the western side of the fjord and covers an area of more than 1000 km2 (Fig. 2). The complex is predominately alkaline and radiometric dating indicates intrusion over an extended period between c. 55 and 40 Ma (Section 2.b). The Kræmer Ø syenite is located on the eastern side of the fjord (Fig. 2), c. 20 km from the coast, and has been dated to 50.7±1.0 Ma by 40Ar/39Ar dating (Tegner et al. Reference Tegner, Brooks, Duncan, Heister and Bernstein2008). The tholeiitic Skaergaard intrusion crops out further SSE at the mouth of the fjord (Fig. 2). An age of 55.96±0.02 Ma has recently been obtained for this intrusion by U–Pb zircon dating (Wotzlaw et al. Reference Wotzlaw, Bindeman, Schaltegger, Brooks and Naslund2012). This age is in good agreement with 40Ar/39Ar ages obtained by Hirschman et al. (Reference Hirschmann, Renne and McBirney1997) on hornblende (55.8±0.3 Ma) and biotite (55.74±0.14 Ma). The tholeiitic Kap Edward Holm layered gabbro is located across the fjord from the Skaergaard intrusion but is significantly younger, as indicated by 40Ar/39Ar ages of hornblende and biotite ranging from 50.8±0.8 to 47.9±0.6 Ma (Nevle et al. Reference Nevle, Brandriss, Bird, McWiliams and O’Neill1994; Tegner et al. Reference Tegner, Duncan, Bernstein, Brooks, Bird and Storey1998). The complex is intruded at its southern and northern perimeters by several syenitic intrusions (Kap Boswell, Kap Deichmann, Hutchinson Gletscher Syenite 1 and 2; Fig. 2). Hutchinson Gletscher Syenite 1 has been dated by the 40Ar/39Ar method to 45.4±1.8 Ma (Nevle et al. Reference Nevle, Brandriss, Bird, McWiliams and O’Neill1994).

Figure 2. Geological map of the Kangerlussuaq Fjord, Greenland, showing major rock types and location of major plutons. Modified from Gleadow & Brooks (Reference Gleadow and Brooks1979), Riishuus et al. (Reference Riishuus, Peate, Tegner, Wilson, Brooks and Harris2006) and Tegner et al. (Reference Tegner, Brooks, Duncan, Heister and Bernstein2008).
Coast-parallel tholeiitic dykes dominate at the mouth of the fjord (Fig. 1) and include three main swarms as mapped by Nielsen (Reference Nielsen1978): THOL-1, TRANS-1 and ALK-1. The tholeiitic THOL-1 dykes were emplaced before rifting and formation of a prominent coast-parallel flexure (Karson & Brooks, Reference Karson, Brooks, MacNiocaill and Ryan1999) and have been interpreted as feeders to the Plateau Lavas. One of these dykes has been dated to 56.9±1.2 Ma by Tegner et al. (Reference Tegner, Duncan, Bernstein, Brooks, Bird and Storey1998). The TRANS-1 dykes are transitional between tholeiitic and alkaline compositions and comparable flood basalts have not been found in East Greenland (Hanghøj, Storey & Stecher, Reference Hanghøj, Storey and Stecher2003). They are considered to be contemporaneous with the Kap Edward Holm Complex (Hanghøj, Storey & Stecher, Reference Hanghøj, Storey and Stecher2003; Tegner et al. Reference Tegner, Brooks, Duncan, Heister and Bernstein2008), consistent with a 40Ar/39Ar age of 48.4±2.8 Ma obtained on one of these dykes (Lenoir, Féraud & Geoffrey, Reference Lenoir, Féraud and Geoffrey2003). The alkaline ALK-1 dykes have not been dated radiometrically, but cross-cutting relations indicate that they post-date the Kap Edward Holm Complex (Tegner et al. Reference Tegner, Brooks, Duncan, Heister and Bernstein2008). The coast-parallel dykes die out further inland where they are replaced by two radiating dyke swarms: THOL-2 and ALK-2 (Nielsen, Reference Nielsen1978). The tholeiitic THOL-2 swarm is found in the outer and middle reaches of the Kangerlussuaq Fjord, but dies out north of the Kærven Complex (Fig. 2; Holm, Heaman & Pedersen, Reference Holm, Heaman and Pedersen2006). The dykes trend parallel to the fjord in the middle reaches of the fjord but have more variable trends in the outer parts, suggesting a radial pattern centred on the fjord mouth c. 10 km SW of the Skaergaard intrusion (Fig. 2; Nielsen, Reference Nielsen1978). The dykes either cut or are cut by the Skaergaard intrusion, indicating syn-break-up emplacement at c. 56–55 Ma. This is consistent with an age of 54.7±0.4 Ma obtained by U–Pb baddeleyite dating of a THOL-2 dyke by Holm, Heaman & Pedersen (Reference Holm, Heaman and Pedersen2006; see Section 2.b). The ALK-2 dykes range in composition from alkali olivine basalt to trachyte (Brooks & Platt, Reference Brooks and Platt1975; Nielsen, Reference Nielsen1978). The swarm has a radial pattern centred on the Amdrup Fjord area (Fig. 2; Nielsen, Reference Nielsen1978). Zircon and titanite fission-track ages indicate emplacement between 41 and 36 Ma (Gleadow & Brooks, Reference Gleadow and Brooks1979).
2.b. The Kangerlussuaq Alkaline Complex (KAC)
The main constituent of the KAC is the roughly circular Kangerlussuaq Syenite (Fig. 2), which consists of an outer ring of nordmarkite (quartz alkali feldspar syenite), an inner ring of pulaskite (alkali feldspar syenite) and a core of foyaite (nepheline syenite) (Wager, Reference Wager1965; Kempe, Deer & Wager, Reference Kempe, Deer and Wager1970; Brooks & Gill, Reference Brooks and Gill1982; Riishuus et al. Reference Riishuus, Peate, Tegner, Wilson and Brooks2008). The nordmarkite zone, which comprises c. 90% of the intrusion (Wager, Reference Wager1965), has been dated to 53±6 Ma by whole-rock Rb–Sr dating (Holm, Reference Holm1991). The pulaskite zone comprises c. 10% of the complex and has been dated by several methods, including K–Ar, Rb–Sr mineral and whole-rock isochron dating; zircon and titanite fission-track dating; and 40Ar/39Ar biotite dating (Hamilton, Reference Hamilton1966; Beckinsale, Brooks & Rex, Reference Beckinsale, Brooks and Rex1970; Pankhurst, Beckinsale & Brooks, Reference Pankhurst, Beckinsale and Brooks1976; Gleadow & Brooks, Reference Gleadow and Brooks1979; Tegner et al. Reference Tegner, Brooks, Duncan, Heister and Bernstein2008). These methods have yielded ages clustering around 50 Ma but with errors that span 54–48 Ma. The 40Ar/39Ar age of 51.1±1.1 Ma obtained by Tegner et al. (Reference Tegner, Brooks, Duncan, Heister and Bernstein2008) is considered the most robust of these ages. The foyaite core comprises <1% of the intrusion and has not been dated radiometrically.
The KAC also includes several smaller intrusions which have been referred to as satellite intrusions (Fig. 2), although the exact genetic relationship between the satellites and the Kangerlussuaq Syenite is unclear (Nielsen, Reference Nielsen, Fitton and Upton1987; Riishuus et al. Reference Riishuus, Peate, Tegner, Wilson, Brooks and Harris2006). The satellite intrusions either cut or are cut by the Kangerlussuaq Syenite and have accordingly been divided into younger and older satellites, respectively.
The older satellites include the Kælvegletscher Ultramafic Complex, Admiraltinden Gabbro, Augite Syenite, Peak 2005 Syenite and the Kærven Complex (Fig. 2). The Kærven Complex crops out over a c. 10 km2 area at the northeast corner of KAC and is cut by the Kangerlussuaq Syenite along a c. 5-km-long, NW–SE-trending semi-linear contact (Fig. 3). The crescent-shaped Kærven Gabbro forms the eastern and oldest part of the Kærven Complex. The gabbro consists of several intrusive units with tholeiitic hypersthene-gabbro as the dominant rock type (Deer & Kempe, Reference Deer and Kempe1976; Holm, Heaman & Pedersen, Reference Holm, Heaman and Pedersen2006). To the north the gabbro cuts a small ultramafic intrusion of tholeiitic affinity (Holm & Prægel, Reference Holm and Prægel2006) and to the east it cuts an east–west-striking tholeiitic dyke, which in turn cuts a fjord-parallel tholeiitic dyke further east (Fig. 3). The latter is inferred to be part of the THOL-2 dyke swarm and a U–Pb baddeleyite age of 54.7±0.4 Ma has been obtained for the dyke by Holm, Heaman & Pedersen (Reference Holm, Heaman and Pedersen2006). The gabbro itself yields 40Ar/39Ar biotite and U–Pb zircon ages of 55.5±1.4 and 53.0±0.3 Ma, respectively (Fig. 3; Holm, Heaman & Pedersen, Reference Holm, Heaman and Pedersen2006; Tegner et al. Reference Tegner, Brooks, Duncan, Heister and Bernstein2008). To the west the gabbro is cut by a series of alkaline intrusions grading westwards from monzonite through syenite to alkali granite (Fig. 3). Two of the felsic intrusions gave 40Ar/39Ar amphibole ages of 54±2 and 53.1±1.3 Ma (Fig. 3; Holm, Reference Holm1991; Tegner et al. Reference Tegner, Brooks, Duncan, Heister and Bernstein2008).

Figure 3. Geological map of the Kærven Complex showing major intrusive units and location of the dated samples. Location and ages of previously dated samples are also shown. Age data from Holm, Heaman & Pedersen (Reference Holm, Heaman and Pedersen2006) and Tegner et al. (Reference Tegner, Brooks, Duncan, Heister and Bernstein2008).
The younger satellites include the Cirque 1320 Complex, Astrophyllite Bay Complex, Snout Series Granite, Biotite Granite, Bagnæsset Syenite Complex and Flammefjeld Complex (Fig. 2). These intrusions are concentrated around the Amdrup Fjord at the southeast margin of the KAC, and geochronology data indicate that most were emplaced between 46 and 48 Ma (Riishuus et al. Reference Riishuus, Peate, Tegner, Wilson, Brooks and Waight2005; Tegner et al. Reference Tegner, Brooks, Duncan, Heister and Bernstein2008). Exceptions are the Cirque 1320 and Flammefjeld complexes, for which Re–Os molybdenite ages of 52.7±0.3 and 39.7±0.2 Ma have been obtained (Brooks et al. Reference Brooks, Tegner, Stein and Thomassen2004; Holwell et al. Reference Holwell, Selby, Boyce, Gilbertson and Abraham-James2012).
3. Samples and intrusive units
Seven samples from six different intrusive units of the Kærven Complex were dated in this study by the ID-TIMS U–Pb technique (Fig. 3). The samples were selected with the aim of covering most of the major intrusive units of the complex. The dated units are monzonitic to granitic (SiO2 = 62–72 wt%; Fig. 4) and are exposed on either side of the Vindhulsdalen Valley (Fig. 3). They have accordingly been grouped into East and West units. The dated samples are metaluminous to weakly peralkaline [(Na + K)/Al = 0.76–1.01] and are characterized by high alkali contents (Fig. 4) and high Fe/(Fe + Mg) ratios (0.82–0.95) typical of ferroan, A-type granitoids (Frost & Frost, Reference Frost and Frost2008).

Figure 4. Whole-rock compositions of the Kangerlussuaq Fjord plotted in the total alkalis vs. silica classification diagram (Le Maitre et al. Reference Le Maitre, Streickeisen, Zanettin, Le Bas, Bonin, Bateman, Bellieni, Dudek, Efremova, Keller, Lameyre, Sabine, Schmid, Sørensen and Woolley2002). Alkaline–tholeiitic division line (dashed line) is from Irvine & Baragar (Reference Irvine and Baragar1971). Tholeiitic rocks are shown as white fields. Alkaline rocks are shown as grey fields. Samples dated in this study are shown as black dots. Abbreviations: PS + AS – Peak 2005 Syenite and Augite Syenite (older satellites of the KAC); PWBF – Prinsen af Wales Bjerge Formation; K-F – Kangerlussuaq Foyaite; K-P – Kangerlussuaq Pulaskite; K-N – Kangerlussuaq Nordmarkite. Younger KAC satellites include the Cirque 1320 Complex, Snout Series Syenite, Astrophyllite Bay Complex, Biotite Granite and Bagnæsset Syenite. Skaergaard granophyre data is from podiform pegmatites and segregation veins of the Skaergaard intrusion. The granophyres record late-stage fractionates of the Skaergaard melt (Larsen & Brooks, Reference Larsen and Brooks1994) and exemplify the evolved rocks of the tholeiitic trend. Alkaline and transitional dykes include the ALK-1, ALK-2 and TRANS-1 dyke swarms of Nielsen Reference Nielsen1978. Tholeiitic dykes include the THOL-1 and THOL-2 swarms. Data compiled from Nielsen (Reference Nielsen1978, Reference Nielsen, Fitton and Upton1987), McBirney (Reference McBirney1989), Larsen & Brooks (Reference Larsen and Brooks1994), Hansen et al. (Reference Hansen, Pedersen, Duncan, Bird, Brooks, Fawcett, Gittins, Gorton, O’Day, Jolley and Bell2002), Peate & Stecher (Reference Peate and Stecher2003), Peate et al. (Reference Peate, Baker, Blichert-Toft, Hilton, Storey, Kent, Brooks, Hansen, Pedersen and Duncan2003), Andreasen, Peate & Brooks (Reference Andreasen, Peate and Brooks2004), Riishuus et al. (Reference Riishuus, Peate, Tegner, Wilson, Brooks and Waight2005, Reference Riishuus, Peate, Tegner, Wilson, Brooks and Harris2006, Reference Riishuus, Peate, Tegner, Wilson and Brooks2008) and Holm, Heaman & Pedersen (Reference Holm, Heaman and Pedersen2006).
East Monzonite (sample 66454) is the easternmost felsic unit of the Kærven Complex and comprises several thin, monzonitic dykes emplaced along the western margin of the Kærven Gabbro (Fig. 3). The dykes can be seen cutting the Kærven Gabbro and locally contain gabbroic inclusions, indicating that the monzonite dykes are younger than the gabbro. The dated sample is porphyritic with c. 15% phenocrysts of patch perthite alkali feldspar (up to 8 mm long) enclosed in a medium-grained groundmass of plagioclase, microperthite, quartz, amphibole, clinopyroxene, biotite, Fe-Ti oxide, zircon and titanite (in order of decreasing abundance; see also online Supplementary Fig. S1a, available at http://journals.cambridge.org/geo).
East Syenite (sample 66503) is a red-brown, 250–300-m-wide syenitic dyke, which cuts both the East Monzonite and the Kærven Gabbro (Fig. 3). The syenite is coarse-grained and composed of microperthite, quartz, amphibole, fayalite, Fe-Ti oxide, clinopyroxene, zircon and apatite (online Supplementary Fig. S1b, available at http://journals.cambridge.org/geo).
East Granite (sample 40333c) is a 20–30-m-wide alkali granite dyke. The dyke intrudes the north–south-trending contact between the Kærven Gabbro and the East Syenite in the southern part of the complex (Fig. 3). It follows this contact for c. 1 km before turning northwest and cutting across the East Syenite in the central part of the complex. The rock is yellowish-white to light grey or pink in colour, with c. 10% phenocrysts of microperthite (typically 3–4 mm long) in a medium-grained groundmass of microperthite, quartz, amphibole, clinopyroxene, Fe-Ti oxide and zircon (online Supplementary Fig. S1c, available at http://journals.cambridge.org/geo).
West Granite 1 (sample 74111) crops out in the area south and west of the 1390 m western peak of Kærven (Fig. 3). The coarse-grained rock has a distinctive red colour and is composed of microperthite, quartz, amphibole, clinopyroxene, Fe-Ti oxide, fayalite, biotite and zircon (online Supplementary Fig. S2a, available at http://journals.cambridge.org/geo).
West Granite 2 (samples 74347 and 74349) is a c. 200-m-wide, north–south-trending, alkali granite dyke intruded into the southeast margin of West Granite 1 (Fig. 3). The dyke has c. 5% phenocrysts of microperthite (3–4 mm) in a medium-grained groundmass of microperthite, quartz, amphibole, Fe-Ti oxide, biotite and zircon (online Supplementary Fig. S2b, available at http://journals.cambridge.org/geo).
West Syenite (sample 74346) forms a c. 600-m-wide syenitic dyke. In the southern part of the complex the dyke trends c. north–south and is emplaced between West Granite 2 to the west and an older syenitic dyke of comparable width to the east (Fig. 3). The latter can be followed northeast across the Vindhulsdalen Valley, where it is cut by the East Granite unit. Further north the West Syenite changes to a NW–SE trend and is observed cutting West Granite 1. The rock is coarse grained and composed of microperthite, amphibole, Fe-Ti oxide, clinopyroxene, apatite and zircon (online Supplementary Fig. S2c, available at http://journals.cambridge.org/geo).
4. Analytical details
The samples were crushed and processed through a combination of panning, magnetic (Frantz isodynamic separator) and density (methylene iodide) separation techniques at the University of Copenhagen to yield heavy mineral concentrates. Zircons of similar size, shape and colour were then handpicked under a binocular microscope to produce a set of homogeneous, multi-grain aliquots.
The U–Pb analytical work was carried out at the University of Alberta and followed the procedures described in detail by Heaman, Erdmer & Owen (Reference Heaman, Erdmer and Owen2002). The selected zircon fractions were washed in warm 4N HNO3 prior to weighing with a UTM ultra-microbalance. The samples were spiked with a mixed 205Pb–235U tracer solution and dissolved in a HF–HNO3 mixture in teflon bombs at 230°C. Purified Pb and U aliquots were analysed on a VG354 multi-collector thermal ionization mass spectrometer (TIMS) using operating conditions outlined in Heaman et al. (Reference Heaman, Erdmer and Owen2002). All isotopic data were corrected for mass fractionation using the following values determined from the long-term reproducibility of the NBS981 and NBS U500 standards: 0.10% per atomic mass unit (amu−1) for Pb and 0.12% amu−1 for U. Blank correction was 2±1 pg Pb and 0.5±0.25 pg U, consistent with routine blank levels at the University of Alberta (Heaman et al. Reference Heaman, Böhm, Machado, Krogh, Weber and Corkery2011).
Common Pb in excess of blank was corrected for using compositions estimated from Pb isotopic analyses of alkali feldspar separates from the Kærven Complex (Table 1). The feldspars are characterized by low Pb isotope ratios and are considerably less radiogenic than Icelandic lavas (e.g. Hanan & Schilling, Reference Hanan and Schilling1997; Stracke et al. Reference Stracke, Zindler, Salters, McKenzie, Blichert-Toft, Albarède and Grönvold2003). Similar compositions have been reported from flood basalts and dykes in the Kangerlussuaq area, and from the Kælvegletscher Ultramafic Complex of the KAC (Figs 2, 3). The un-radiogenic Pb isotope compositions have been interpreted to reflect contamination of Iceland plume magmas by Archaean continental crust and/or contributions from metasomatized continental lithospheric mantle (e.g. Hansen & Nielsen, Reference Hansen and Nielsen1999; Hanghøj, Storey & Stecher, Reference Hanghøj, Storey and Stecher2003; Holm & Prægel, Reference Holm and Prægel2006; Peate et al. Reference Peate, Barker, Riishuus and Andreasen2008). Sample 66454 (East Monzonite) has been corrected using the composition: 206Pb/204Pb = 15.5±0.6 and 207Pb/204Pb = 14.91±0.10 (average of three monzonite samples from East Kærven; Table 1). All other samples have been corrected using the composition: 206Pb/204Pb = 16.22±0.11; 207Pb/204Pb = 15.02±0.05 (average of five syenite and granite samples from East and West Kærven).
Table 1. Pb isotopic compositions of alkali feldspar separates from the Kærven Complex

Feldspar separates were analysed using a VG Sector 54 TIMS at the University of Copenhagen following standard dissolution and ion exchange procedures. The data were corrected for mass fractionation using a value of 0.12±0.02 (2σ) % amu−1 based on replicate measurements of the NBS981 standard and the values of Todt et al. (Reference Todt, Cliff, Hanser and Hofmann1996).
A correction for 230Th disequilibrium has been applied using the equation of Schärer (Reference Schärer1984) and assuming a Th/Umagma ratio of 5 (average value estimated from whole-rock data). Calculations indicate that the correction is robust to large variations in magmatic Th/U. The correction increases the 206Pb/238U age by c. 0.1 Ma for all fractions, except the high Th/U fraction 74347–2 (Table 2) for which the correction is negligible. The overall effect of the correction is to increase the degree of concordance.
Table 2. ID-TIMS U–Pb zircon results from the Kærven Complex

Atomic ratios are corrected for spike, blank and common Pb (see Table 1). Blank composition: 206Pb/204Pb = 18.24±0.07; 207Pb/204Pb = 15.64±0.06; 208Pb/204Pb = 37.50±0.15. 206Pb/238U and 206Pb/207Pb values have been corrected for excess 206Pb assuming Th/U = 5 for the parent magma and using the equation of Schärer (Reference Schärer1984). acl – colourless; y – yellow; eu – euhedral; sub – subhedral, pr – prismatic; eq – equant; fr – fragments; [40] number of grains in fraction; blongest dimension; cthorium concentration is estimated from amount of 208Pb and 206Pb/238U age; and dTCPb = total common Pb (initial + blank).
Data plotting and age calculations were performed with the ISOPLOT software of Ludwig (Reference Ludwig2003) using decay constants of 1.55125×10–10 a−1 (238U) and 9.8485×10–10 a−1 (235U) and a 238U/235U ratio of 137.88 as recommended by Steiger & Jäger (Reference Steiger and Jäger1977). The uncertainties listed in Table 2 were calculated by propagation of all known sources of error and are quoted at the 2σ level.
5. U–Pb results
5.a. East Monzonite (sample 66454)
Two multi-grain zircon fractions were analysed from this sample. Fraction 66454–1 consists principally of euhedral, prismatic crystals, 100–200 μm long with a predominance of {100} and {101} crystal faces. The zircons of 66454–2 are more equant and show a combination of {100}, {110} and {101} crystal faces. The two fractions are characterized by very low U (58 and 45 ppm) and Pb (<1 ppm) contents and relatively high Th/U ratios (0.94 and 0.85; Table 2). Fraction 66454–1 plots within error of the concordia curve with a 206Pb/238U age of 53.2±0.2 Ma (Fig. 5). Fraction 66454–2 yields a 206Pb/238U age of 53.0±0.2 Ma, within error of fraction 66454–1. The latter is normally discordant and plots at a high angle to the concordia, which may indicate minor inheritance coupled with Pb loss.

Figure 5. Wetherill concordia diagram showing U–Pb results of zircon fractions from East Kærven.
5.b. East Syenite (sample 66503)
The zircons recovered from sample 66503 range from c. 100 to 300 μm in length and are generally colourless, euhedral, equant to prismatic crystals showing a combination of {101}, {100} and {110} crystal faces. The larger grains were sampled in fraction 66503–1, whereas the smaller grains were sampled in fraction 66503–2. The fractions have low to moderate U and Th contents (U = 341–448 ppm; Th/U = 0.68–0.71; Table 2). Fraction 66503–1 plots slightly to the right of the concordia with a 206Pb/238U age of 53.0±0.2 Ma (Fig. 5). Fraction 66503–2 is concordant and yields a 206Pb/238U age of 53.5±0.2 Ma. The spread in 206Pb/238U ages could be due to minor Pb loss or recycling of antecrysts (e.g. Miller & Wooden, Reference Miller and Wooden2004; Miller et al. Reference Miller, Matzel, Miller, Burgess and Miller2007).
5.c. East Granite (sample 40333c)
The zircon crystals extracted from sample 40333c form a fairly homogeneous population of small (55–175 μm long), euhedral, prismatic grains with a predominance of {100} and {101} crystal faces (see online Supplementary Fig. S3a, available at http://journals.cambridge.org/geo). The crystals are generally colourless and clear, although some contain small needle-shaped mineral inclusions. The zircons have relatively low U and Th contents (U = 282–368 ppm; Th/U = 0.61–0.69; Table 2). The two analysed fractions plot within error of each other and yield a combined concordia age of 53.4±0.1 Ma (MSWD = 1.6, concordance + equivalence; Fig. 5). This age is within error of an 40Ar/39Ar age (54±2 Ma) obtained on amphibole from the same sample (Holm, Reference Holm1991).
5.d. West Granite 1 (sample 74411)
The zircons recovered from sample 74111 are morphologically similar to the zircons of 40333c; they are slightly turbid and more subhedral however, and prism fragments are more common (see online Supplementary Fig. S3b, available at http://journals.cambridge.org/geo). The analysed fractions have low to moderate U and Th contents (U = 387–487 ppm; Th/U = 0.77–0.88; Table 2). The discordant fraction 74111–1 yields a 206Pb/238U age of 56.5±0.4 Ma (not shown). The analysis is compromised by a high common Pb content (362 pg Pb), making it highly sensitive to the common Pb correction. We suspect that this may be due to laboratory contamination and the fraction will therefore not be considered further. Fraction 74111–2 plots within error of the concordia with a 206Pb/238U age of 53.7±0.1 Ma (Fig. 6). This fraction also has a relatively high common Pb content (30 pg Pb), but has a much higher proportion of radiogenic Pb (206Pb/204Pb = 933) and is therefore less sensitive to the common Pb correction. For example, the 206Pb/238U age decreases by a maximum of 0.1 Ma if up to 30 pg Pb blank is assumed.

Figure 6. Wetherill concordia diagram showing U–Pb results of zircon fractions from West Kærven.
5.e. West Granite 2 (samples 74347 and 74349)
Zircons from sample 74349 occur as colourless, euhedral, slender prisms (75–175 μm) with a predominance of {100} and {101} crystal faces (see online Supplementary Fig. S3c; available at http://journals.cambridge.org/geo). The analysed fractions have low U and Th contents (U = 293–307 ppm; Th/U = 0.53–0.63). Fraction 74349–1 is within error of the concordia with a 206Pb/238U age of 53.8±0.8 Ma (Fig. 6). The large age uncertainty reflects a proportionally low content of radiogenic Pb (64% of total Pb) and a small sample size. The discordant fraction 74349–2 yields a somewhat older 206Pb/238U age of 55.2±0.2 Ma, presumably due to inheritance.
Zircons from sample 74347 are slightly shorter (50–125 μm) than the zircons from 74349 but are otherwise morphologically similar. Fraction 74347–2 has the highest U and Th contents observed in this study (U = 970 ppm; Th/U = 7.7) and is highly discordant with a 207Pb/206Pb age of 1346±161 Ma, indicating inclusion of an inherited Precambrian component. Fraction 74347–1 is also discordant, but markedly less so than fraction 74347–2 and yields a 206Pb/238U age of 53.2±0.2 Ma (Fig. 6).
5.f. West Syenite (sample 74346)
Fractions 74346–1 and 74346–2 are characterized by yellowish, prismatic to equant, subhedral zircons dominated by {100} and {101} crystal faces. The analysed fractions have low U and Th contents (U = 118–240 ppm; Th/U = 0.37 and 0.43). The U–Pb results are within error of the concordia curve, albeit marginally so (<5% probability of concordance), and yield 206Pb/238U ages of 52.3±0.2 and 53.2±0.3 Ma, respectively (Fig. 6). The age spread may reflect Pb loss and/or recycling of antecrysts.
6. Discussion
6.a. Age constraints from the Kærven Complex
Concordant analyses from East Kærven yield 206Pb/238U ages between 53.5±0.2 and 53.2±0.2 Ma. Absolute age differences could not be resolved with the technique used in this study, suggesting intrusion within a relatively short time span (c. 100–200 ka). Cross-cutting relationships, however, indicate that the East Granite is the youngest of the dated East Kærven units, whereas the East Monzonite is the oldest. The concordia age of the East Granite (53.4±0.1 Ma) unit is within error of a previous 40Ar/39Ar age estimate (Holm, Reference Holm1991); we therefore consider this a robust minimum age for the East Kærven units and propose that most of East Kærven was emplaced between c. 53.5 and 53.3 Ma. The 206Pb/238U ages from East Kærven are younger than the 55.5±1.4 Ma 40Ar/39Ar age obtained by Tegner et al. (Reference Tegner, Brooks, Duncan, Heister and Bernstein2008) for the Kærven Gabbro and is consistent with field relations, which indicate that the East Kærven units post-date the gabbro. However, U–Pb baddeleyite dating of a gabbro pegmatite from the northern margin of the Kærven Gabbro (Fig. 3) has yielded an age of 53.0±0.3 Ma (Holm, Heaman & Pedersen, Reference Holm, Heaman and Pedersen2006), indicating that gabbroic magmatic activity continued penecontemporaneously with intrusion of the East Kærven units. Intrusion of the gabbro pegmatite, however, does not appear to have affected the K–Ar system of the gabbro sample dated by Tegner et al. (Reference Tegner, Brooks, Duncan, Heister and Bernstein2008). We therefore surmise that the gabbro pegmatite records a relatively small pulse of mafic magma intruded after most of the gabbro had cooled below the closure temperature for Ar in biotite (c. 300°C; McDougall & Harrison, Reference McDougall and Harrison1988).
Concordant analyses from West Kærven yield ages between 53.8±0.8 and 52.3±0.3 Ma and are within error of an 40Ar/39Ar age of 53.1±1.3 Ma obtained from the westernmost Kærven unit by Tegner et al. (Reference Tegner, Brooks, Duncan, Heister and Bernstein2008) (Fig. 3). The U–Pb ages mostly overlap with the ages from East Kærven and suggest coeval emplacement of the East and West Kærven units. The West Syenite could be slightly younger, but the data are inconclusive due to a large spread in 206Pb/238U ages.
6.b. Age of the Kangerlussuaq Syenite
The Kærven Complex is cut to the south by the Kangerlussuaq Syenite (Figs 2, 3). The syenite was originally mapped by Wager (Reference Wager1965) who noted a lack of internal intrusive contacts and interpreted the intrusion to have formed by fractional crystallization inwards from the margins of a single large magma chamber. Kempe et al. (Reference Kempe, Deer and Wager1970) later reported gradational variations in modal contents between the different zones mapped by Wager (Reference Wager1965), and concurred with a temporal evolution from early (outer) nordmarkite to late (inner) pulaskite and foyite.
A more complicated model is presented by Riishuus et al. (Reference Riishuus, Peate, Tegner, Wilson and Brooks2008) involving concurrent assimilation and fractional crystallization and recharge with more primitive magma, but with the same overall temporal pattern. However, Holm, Prægel & Egeberg (Reference Holm, Prægel and Egeberg1991) report abrupt rather than gradual changes in mineralogy as the nordmarkite-pulaskite boundary is traversed and propose that the Kangerlusuaq Syenite is in fact composed of a number of discrete intrusive units. This is supported by the occurrence of intrusive sheets of pulaskite within the nordmarkite zone in the Amdrup Fjord region (C.K. Brooks, personal communication cited in Nielsen Reference Nielsen, Fitton and Upton1987). Unfortunately, most efforts on dating the Kangerlussuaq Syenite have focused on the pulaskite zone; the 53±6 Ma whole-rock Rb–Sr age of Holm (Reference Holm1991) is to our knowledge the only radiometric age from the nordmarkite zone. A maximum age of c. 53.5 Ma is however provided by the U–Pb results for East Kærven and minimum age constraints are provided by the satellite complexes that intrude the eastern margin of the Kangerlussuaq Nordmarkite. Existing geochronlogical data indicates that most of the younger satellites were intruded between 48 and 46 Ma.
However, Holwell et al. (Reference Holwell, Selby, Boyce, Gilbertson and Abraham-James2012) recently obtained a Re–Os age of 52.7±0.3 Ma for molybdenite-bearing quartz veins cutting the Cirque 1320 Complex (Fig. 2). This method has been shown to be a remarkably robust chronometer under almost all geological conditions when applied to molybdenite (Stein et al. Reference Stein, Markey, Morgan, Hannah and Scherstén2001). The Re–Os age is significantly older than the 51.1±1.1 Ma 40Ar/39Ar age reported by Tegner et al. (Reference Tegner, Brooks, Duncan, Heister and Bernstein2008) on biotite from the Kangerlussuaq Pulaskite. However, given the relatively low closure temperature for Ar in biotite and the size of the Kangerlussuaq Syenite, the 40Ar/39Ar age should probably be considered a cooling age rather than a crystallization age.
We therefore infer that the Kangerlussuaq Nordmarkite was intruded between c. 53.5 and 52.7 Ma. If the single-intrusion model (e.g. Wager Reference Wager1965; Riishuus et al. Reference Riishuus, Peate, Tegner, Wilson and Brooks2008) is correct, then the age of the nordmarkite can be considered representative of the entire Kangerlussuaq Syenite. If not (e.g. Holm, Reference Holm1991), we opine that the pulaskite, and possibly also the foyaite, was intruded penecontemporaneously with the nordmarkite, although more precise age determinations of the pulaskite are required for confirmation. The ages of the Augite and Peak 2005 Syenite intrusions (Fig. 1), which pre-date the Kangerlussuaq Syenite, are not well constrained. However, based on geochemical similarities (Riishuus et al. Reference Riishuus, Peate, Tegner, Wilson, Brooks and Harris2006), we infer that they were intruded shortly before the Kangerlussuaq Syenite. We therefore propose that most of the alkaline part of the KAC was intruded between c. 53.5 and 52.7 Ma, prior to the crossing of the rifted margin over the axis of the Iceland mantle plume between 50 and 47 Ma (Bernstein et al. Reference Bernstein, Kelemen, Tegner, Kurz, Blusztajn and Brooks1998; Tegner et al. Reference Tegner, Brooks, Duncan, Heister and Bernstein2008).
6.c. Regional perspective
The chronology of the Kangerlussuaq Fjord lineament has recently been reviewed by Tegner et al. (Reference Tegner, Brooks, Duncan, Heister and Bernstein2008). These workers concluded that activity in the fjord was continuous from c. 55 to 40 Ma and therefore different from the coastal area further south where magmatic activity was episodic and confined to the time windows 56–54, 50–47 and 37–35 Ma. They note, however, that most of the activity in the Kangerlussuaq Fjord occurred within the time windows quoted above and interpret the post-break-up alkaline intrusions as products of off-axis magmatism in relation to a northwards-propagating mid-ocean ridge, in addition to influences from the Iceland mantle plume. We agree with Tegner et al. (Reference Tegner, Brooks, Duncan, Heister and Bernstein2008) that activity in the Kangerlussuaq Fjord was to a large extent continuous. However, in light of the new age constraints discussed in the previous section (see also Fig. 7), it appears that most of the alkaline activity occurred prior to 50 Ma. It is also noteworthy that the oldest pre-50 Ma alkaline rocks are found north of Kærven in the inner parts of the fjord and further inland (Gardiner Complex and Prinsen af Wales Bjerge Formation), but become progressively younger towards the mouth of the fjord (Figs 1, 7). This indicates that the locus of alkaline magmatism migrated in a SSE direction. The post-50 Ma alkaline activity appears to have been more episodic and confined to the outer parts of the fjord and in the coastal region.

Figure 7. Summary of precise (≤2.5% 2σ error) U–Pb, 40Ar/39Ar, Rb–Sr and Re–Os ages for alkaline (filled symbols) and tholeiitic (unfilled symbols) intrusions of the Kangerlussuaq Fjord compiled from the literature. Data from Nevle et al. (Reference Nevle, Brandriss, Bird, McWiliams and O’Neill1994), Hirschman et al. (Reference Hirschmann, Renne and McBirney1997), Tegner et al. (Reference Tegner, Duncan, Bernstein, Brooks, Bird and Storey1998, Reference Tegner, Brooks, Duncan, Heister and Bernstein2008), Riishuus et al. (Reference Riishuus, Peate, Tegner, Wilson, Brooks and Waight2005), Holm, Heaman & Pedersen (Reference Holm, Heaman and Pedersen2006), Holwell et al. (Reference Holwell, Selby, Boyce, Gilbertson and Abraham-James2012), Wotzlaw et al. (Reference Wotzlaw, Bindeman, Schaltegger, Brooks and Naslund2012) and this study (concordia age for East Granite). Error bars indicate 2σ errors. Also shown are age ranges of Plateau Lavas (tholeiitic) and Prinsen af Wales Bjerge Formation (PWBF) lavas (alkaline) (including 2σ errors; data from Peate et al. Reference Peate, Baker, Blichert-Toft, Hilton, Storey, Kent, Brooks, Hansen, Pedersen and Duncan2003; Storey, Duncan & Tegner Reference Storey, Duncan and Swisher III2007). Age 50.0±0.6 for Kap Edward Holm Complex is weighted average of five 40Ar/39Ar ages (Nevle et al. Reference Nevle, Brandriss, Bird, McWiliams and O’Neill1994). Y-axis indicates fjord-parallel distance from the mouth of the Kangerlussuaq Fjord at c. 68°01’N and 31°45’W. Distances have been calculated by projecting intrusion centres onto a NNW–SSE-trending line through the Kangerlussuaq Fjord. Distance to Prinsen af Wales Bjerge Formation lavas is with reference to crater site mapped by Hansen et al. (Reference Hansen, Pedersen, Duncan, Bird, Brooks, Fawcett, Gittins, Gorton, O’Day, Jolley and Bell2002). Abbreviations: cc – Cirque 1320 complex; sss – Snout Series Syenite; abc – Astrophyllite Bay Complex; bg – Biotite Granite; KEH – Kap Edward Holm Gabbro Complex.
To explain this pattern, we propose that the pre-50 Ma alkaline intrusions and lavas record the track of the Iceland hotpot during the rift-to-drift transition. The proposed hotspot track is in accordance with plate-tectonic reconstructions which generally involve passage of the Iceland hotspot through or close to the Kangerlussuaq Fjord (Fig. 1; e.g. Lawver & Müller, Reference Lawver and Müller1994; Torsvik et al. Reference Torsvik, Mosar and Eide2001; Braun et al. Reference Braun, Kim, Csatho and von Frese2007). It is also consistent with local dome-like uplift in the Kangerlussuaq area around 50 Ma, as proposed by Gleadow and Brooks (Reference Gleadow and Brooks1979) on the basis of field evidence and fission-track thermochronology. Assuming that the plume axis reached the coast at c. 50 Ma (Bernstein et al. Reference Bernstein, Kelemen, Tegner, Kurz, Blusztajn and Brooks1998; Tegner et al. Reference Tegner, Duncan, Bernstein, Brooks, Bird and Storey1998) but was located c. 50 km further inland at c. 53.5 Ma, as recorded by the Kærven Complex discussed here, leads to an estimated average NW-directed continental drift of c. 1.5 cm a−1. This is considerably slower than the 4.4 cm a−1 half-spreading rate suggested by Larsen & Saunders (Reference Larsen, Saunders, Saunders, Larsen and Wise1998) for the initial stages of seafloor spreading; however, it is in agreement with more recent results which indicate that the half-spreading rate dropped from an initial 2.2 cm a−1 to 1.5 cm a−1 after anomaly 24 (c. 53 Ma), before stabilizing at 1.0 cm a−1 after anomaly 21 (c. 47 Ma) (Smallwood & White, Reference Smallwood, White, Jolley and Bell2002).
Using these rates and assuming that the plume was positioned beneath Kærven at 53.5 Ma, we can backtrack the position of the plume to an area near the Gardiner Complex and the Prinsen af Wales Bjerge Formation at c. 56 Ma (Fig. 1). This is consistent with the oldest ages determined for these centres (Fig. 7). On the other hand, at c. 47 Ma the plume would have been positioned roughly beneath the continent–ocean boundary (Fig. 1).
The pre-50 Ma alkaline centres of the Kangerlussuaq Fjord likely record low-degree melts produced by upwelling of the mantle plume beneath a relatively thick lithospheric lid, as suggested by Peate et al. (Reference Peate, Baker, Blichert-Toft, Hilton, Storey, Kent, Brooks, Hansen, Pedersen and Duncan2003) for the Prinsen af Wales Bjerge Formation. This process may have been facilitated by thinning of the lithosphere during the rifting attempt recorded by the THOL-2 dykes and the tholeiitic Kærven Gabbro. Conversely, passing of the mantle plume axis beneath thinner lithosphere at the East Greenland rifted margin at 50–47 Ma resulted in greater degrees of melting and a broader melting column. This led to renewed tholeiitic magmatism in the coastal region (e.g. Kap Edward Holm Complex), as well as minor transitional to alkaline activity (TRANS-1 and ALK-1 dykes, and younger satellites of the KAC; Figs 1, 7).
The rifted margin had migrated away from the plume axis at c. 47 Ma, resulting in a decline in magmatism. Sporadic alkaline magmatic activity is however recorded in the Kangerlussuaq Fjord between c. 45 and 35 Ma by the ALK-2 dykes, the Hutchinson Gletscher Syenite 1 and the Flammefjeld complex (Brooks et al. Reference Brooks, Tegner, Stein and Thomassen2004; Tegner et al. Reference Tegner, Brooks, Duncan, Heister and Bernstein2008; Fig. 7). The cause of this final phase of activity is unclear, but we speculate that the northwards propagation of the proto-Reykjanes Ridge/Kolbeinsey Ridge system at c. 49–47 Ma may have played a role (Gaina, Gernigon & Ball, Reference Gaina, Gernigon and Ball2009). Minor alkaline activity in the Wiedemann Fjord – Kronborg Gletscher lineament north of Kangerlussuaq between c. 52 and 36 Ma and the 49–44 Ma tholeiitic–alkaline Kap Dalton Group sequence (Fig. 1) may also have been related to this event (Tegner et al. Reference Tegner, Brooks, Duncan, Heister and Bernstein2008; Larsen et al. Reference Larsen, Pedersen, Sørensen, Watt and Duncan2013). Alternatively, this phase was related to a late episode of epeirogenic uplift and erosion culminating at c. 35 Ma, as indicated by fission-track thermochronology (Gleadow & Brooks, Reference Gleadow and Brooks1979), possibly in response to plate tectonic reorganization associated with cessation of seafloor spreading in the Labrador Sea (Brooks et al. Reference Brooks, Tegner, Stein and Thomassen2004; Tappe et al. Reference Tappe, Foley, Stracke, Romer, Kjarsgaard, Heaman and Joyce2007; Tegner et al. Reference Tegner, Brooks, Duncan, Heister and Bernstein2008).
7. Conclusions
Zircons from six monzonitic, syenitic and granitic intrusive units of the eastern and western parts of the alkaline Kærven Complex have been dated by the U–Pb technique. Zircons from East Kærven units yield concordant and overlapping U–Pb ages, indicating intrusion within a relatively short time span between c. 53.5 and 53.3 Ma. Zircons from West Kærven show a greater spread in ages but are generally within range of the East Kærven zircons, indicating that most of the felsic units are of similar age.
The U–Pb ages from East Kærven are younger than the 55.5±1.4 Ma 40Ar/39Ar age of Tegner et al. (Reference Tegner, Brooks, Duncan, Heister and Bernstein2008) for the tholeiitic Kærven Gabbro, consistent with field evidence indicating that the felsic units largely post-date the gabbro. Minor gabbroic activity may, however, have occurred penecontemporaneously with the felsic intrusions as indicated by the 53.0±0.3 Ma U–Pb age for a gabbro pegmatite by Holm, Heaman & Pedersen (Reference Holm, Heaman and Pedersen2006).
The U–Pb ages of the Kærven Complex and the Re–Os age of the Cirque 1320 Complex (Holwell et al. Reference Holwell, Selby, Boyce, Gilbertson and Abraham-James2012) constrain the Kangerlussuaq Nordmarkite to have been intruded between c. 53.5 and 52.7 Ma, and we infer that most of the KAC was intruded during this time window.
The alkaline intrusions of the Kangerlussuaq Fjord are considered to record the track of the Iceland hotspot during the rift-to-drift transition of the East Greenland rifted margin. Our calculations indicate that the axis of the plume was positioned in the area around the Gardiner Complex and the Prinsen af Wales Bjerge Formation during continental break-up. At c. 47 Ma the plume axis was positioned roughly beneath the continent–ocean boundary.
Acknowledgements
LMH acknowledges financial support from NSERC (Discovery and Major Resources Support Grants). J. Schultz is gratefully thanked for assistance in the laboratory and help with mineral picking. G. Hatchard and J. LeBlanc are thanked for help with TIMS analyses at the University of Alberta. Mineral separation was carried out by P. Venslev (University of Copenhagen). The manuscript has benefited from constructive criticism by F. Corfu and an anonymous reviewer, for which we are sincerely grateful.
Supplementary material
To view supplementary material for this article, please visit http://dx.doi.org/10.1017/S0016756815000448.