Introduction
Unravelling past long-term histories of glacier advances and retreats is the only way to constrain how the Antarctic ice sheet will behave in the future in a context of increasing temperatures and its impact on sea-level rise (e.g. Ritz et al. Reference Ritz, Edwards, Durand, Payne, Peyaud and Hindmarsh2015). The East Antarctic Ice Sheet (EAIS) has the highest potential impact on eustatism, due to a combined effect of its low, mainly below sea level, continental basement elevation and its greater ice thickness (Fig. 1). However, the on-land erosional history of East Antarctica remains largely unconstrained between the Transantarctic Mountains and the Lambert Glacier (Zwartz et al. Reference Zwartz, Bird, Stone and Lambeck1998, Verleyen et al. Reference Verleyen, Hodgson, Milne, Sabbe and Vyverman2005, Mackintosh et al. Reference Mackintosh, White, Fink, Gore, Pickard and Fanning2007), and it relies largely on marine sediment analyses (e.g. Bentley et al. Reference Bentley, Cofaigh, Anderson, Conway, Davies and Graham2014). Constraining the past erosion history by means of airborne geophysical surveys (e.g. Aitken et al. Reference Aitken, Roberts, van Ommen, Young, Golledge and Greenbaum2016) remains speculative due to the absence of direct dating of the subglacial morphologies. Consequently, estimates of long-term (> 1 Ma) erosion of Antarctica are variable and generally ill-defined (see discussion in Rolland et al. Reference Rolland, Bernet, van der Beek, Gautheron, Duclaux and Bascou2019). On the one hand, the development of large periglacial basins during the Permian (Isbell et al. Reference Isbell, Henry, Gulbranson, Limarino, Fraiser and Koch2012) together with a major phase of exhumation of the Terre Adélie craton recorded at 350–300 Ma in the coastal region (i.e. Rolland et al. Reference Rolland, Bernet, van der Beek, Gautheron, Duclaux and Bascou2019) suggest that a significant part of the glacial erosion occurred during the Late Palaeozoic Ice Age (LPIA) (Fig. 2). On the other hand, post-LPIA extensional tectonics (e.g. Lisker & Olesch Reference Lisker and Olesch2003, Maritati et al. Reference Maritati, Aitken, Young, Roberts, Blankenship and Siegert2016, unpublished data 2019) and the largely unconstrained Cenozoic erosion (Thomson et al. Reference Thomson, Reiners, Hemming and Gehrels2013) may have also influenced the Antarctic topography and then the erosion pattern. In this context, moraines provide the opportunity to sample areas of inland East Antarctica largely hidden below the ice using thermochronology of basement rocks (e.g. Cox et al. Reference Cox, Thomson, Reiners, Hemming and van de Flierdt2010). Combining low-temperature thermochronology and terrestrial cosmogenic nuclide (TCN) dating of moraine boulders allows for the potential construction of the long-term erosion history of larger portions of the Antarctic continent.

Fig. 1. a. General topographical map of Antarctica, with the location of Terre Adélie. b. Location of the investigated moraines and thermochronological transect in Terre Adélie-George V Land, East Antarctica. Small circles represent samples dated by apatite fission-track and apatite He dating in Lisker & Olesch (Reference Lisker and Olesch2003) (yellow circles) and Rolland et al. (Reference Rolland, Bernet, van der Beek, Gautheron, Duclaux and Bascou2019) (red circles), undertaken on the Terre Adélie crystalline basement.

Fig. 2. Reconstruction of maximum glaciation during the Late Palaeozoic Ice Age (LPIA; at c. 300 Ma) based on glacier data compiled in Isbell et al. (Reference Isbell, Henry, Gulbranson, Limarino, Fraiser and Koch2012) and modified from Rolland et al. (Reference Rolland, Bernet, van der Beek, Gautheron, Duclaux and Bascou2019). Positions of several LPIA Antarctic ice sheets are suggested following ice-flow directions. The Terre Adélie-George V Land glacier (TA-GV) is proposed as one of the main Antarctic glaciers based on combined erosion along the Terre Adélie coast and constant ice-flow directions along the Transantarctic Mountains, while others remain speculative. The position of the South Palaeopole at 300 Ma is constrained from Torsvik et al. (Reference Torsvik, Müller, van der Voo, Steinberger and Gaina2008). AFT = apatite fission-track, AHe = apatite helium.
Here, we report combined apatite fission-track (AFT) and 10Be TCN dating of boulders from two moraines, Cap Prud'homme and Lacroix Nunatak in Terre Adélie (Fig. 1), in order to constrain the more inland erosion history of the East Antarctic basement. Based on the glacier flux maps, Cap Prud'homme is a frontal moraine located close to the Dumont D'Urville (DDU) French Base, while the Lacroix Nunatak moraine is a lateral moraine (Fig. 3). Both moraines record lithologies that are mostly different from the coastal region, including acidic volcanic rocks, sedimentary rocks and granites that do not occur along the coast (Peucat et al. Reference Peucat, Capdevila, Fanning, Ménot, Pécora and Testut2002), which reflects significant transport below the ice. Although their exact source region remains unknown, the glacier flux maps suggest a probable boulder transport of up to 500 km between 135 and 140°E as suggested by the upstream repartition of the channelized ice flow (Fig. 3) (Rignot et al. Reference Rignot, Mouginot and Scheuchl2011). The AFT datings from moraines are compared to those previously obtained by Rolland et al. (Reference Rolland, Bernet, van der Beek, Gautheron, Duclaux and Bascou2019) on a 600 km transect along the Terre Adélie-George V Land coast. These data are used to question the homogeneity of East Antarctic erosion towards the core of East Antarctica. The 10Be TCN datings are undertaken on the same boulders that have been dated by AFT to determine whether there could be any relationship between the exposure age and the source region. In addition, these datings are the first direct exposure datings undertaken on-land in Terre Adélie, and they allow us to question the age of deglaciation stages during the Quaternary.

Fig. 3. Glacial flux map of the Terre Adélie-George V Land coast from Rignot et al. (Reference Rignot, Mouginot and Scheuchl2011). Note that Lacroix Nunatak (LN) is located in a lateral moraine position, while the Cap Prud'homme (CP) moraine is placed in a more frontal position with respect to the main glacial flux. The position of the Last Glacial Maximum (LGM) glacial front at 20 ka is also indicated by the dashed black line. The probable source of boulders (some 400–500 km upstream) is estimated based on the highest ice velocities in the zone of focused glacial flow. Bathymetry is from Beaman et al. (Reference Beaman, O'Brien, Post and de Santis2011).
Geological setting: erosion and past geological history in East Antarctica
On the basis of aerogeophysical data, the topography of the Antarctic continent is shown to be complex, which is ascribed 1) to the erosion during early stages of glaciation (c. 30 Ma; Cox et al. Reference Cox, Thomson, Reiners, Hemming and van de Flierdt2010), 2) to efficient erosion by repeated large-scale glacier advances and retreats (e.g. Thomson et al. Reference Thomson, Reiners, Hemming and Gehrels2013, Aitken et al. Reference Aitken, Roberts, van Ommen, Young, Golledge and Greenbaum2016), 3) to pre-Cenozoic tectonics (e.g. Ferraccioli et al. Reference Ferraccioli, Finn, Jordan, Bell, Anderson and Damaske2011) and/or 4) former erosion phases inherited from the LPIA (Rolland et al. Reference Rolland, Bernet, van der Beek, Gautheron, Duclaux and Bascou2019).
In the Terre Adélie and George V Land region of East Antarctica (Fig. 1), bedrock exposures occur mainly along the coast, on small islands of < 1 km2. The Terre Adélie crystalline basement is made of high-grade metamorphic rocks (granulites) dating from the Neoarchaean (c. 2.5 Ga) and the Palaeoproterozoic (1.6–1.7 Ga; Duclaux et al. Reference Duclaux, Rolland, Ruffet, Ménot, Guillot and Peucat2008, Naumenko-Dèzes et al. Reference Naumenko-Dèzes, Rolland, Lamarque, Duclaux, Gallet, Bascou and Ménot2020), accreted to a Neoproterozoic to lower Cambrian block in the eastern George V Land region (Lamarque et al. Reference Lamarque, Bascou, Ménot, Paquette, Couzinié, Rolland and Cottin2018). Almost no post-Proterozoic deformation has been recognized except for discrete east to west faults and joints ascribed to rifting of the Antarctic Ocean during the Mesozoic (160–95 Ma). Thermochronological data obtained across the 600 km Terre Adélie coast (Rolland et al. Reference Rolland, Bernet, van der Beek, Gautheron, Duclaux and Bascou2019) show a weighted mean of 276 ± 4.3 Ma (AFT) and 235 ± 5.4 Ma (apatite helium ages). Temperature-time (T-t) cooling paths based on AFT data are in agreement with a phase of cooling between 350 and 300 Ma, interpreted as glacial erosion during the LPIA (Rolland et al. Reference Rolland, Bernet, van der Beek, Gautheron, Duclaux and Bascou2019). In the neighbouring Wilkes Basin, Maritati et al. (unpublished data 2019) report some zircon and apatite U-Th/He age T-t modelling consistent with a slightly later erosion compatible with rifting in the Permian-Triassic (280–220 Ma). Apart from the Cenozoic ages obtained along the Transantarctic Mountains, which suggests a zone of mantle upwelling (Balestrieri et al. Reference Balestrieri, Olivetti, Rossetti, Gautheron, Cattò and Zattin2020), similarly old AFT ages ranging from 350 to 250 Ma were obtained in the Miller Range of the Transantarctic Mountains, in the Lambert Glacier area (see review in Rolland et al. Reference Rolland, Bernet, van der Beek, Gautheron, Duclaux and Bascou2019). These data are in agreement with little erosion and tectonic denudation since Permian times and through Mesozoic and Cenozoic times, and thus are suggestive of mostly inefficient glacial erosion since the establishment of the polar ice sheet. In high-latitude regions, erosion is bound to be low due to the cold-based nature of glaciers, but was probably more efficient during the hottest Cenozoic interglacial periods (Thomson et al. Reference Thomson, Reiners, Hemming and Gehrels2013, Paxman et al. Reference Paxman, Jamieson, Hochmuth, Gohl, Bentley, Leitchenkov and Ferraccioli2019). In addition, the Antarctic topography has become, on average, progressively lower-lying since 34Ma due to ongoing subsidence below a 3 km ice cap (Paxman et al. Reference Paxman, Jamieson, Hochmuth, Gohl, Bentley, Leitchenkov and Ferraccioli2019), which led to less efficient erosion. However, it remains the case that few data have been obtained from most of East Antarctica, which hinders any precise reconstruction of its erosion history.
Analytical procedures
Apatite fission-track geochronology
Low-temperature thermochronology is applied to pinpoint the cooling and exhumation history of upper crustal rocks. This method permits accurate recalculating of the T-t cooling path of individual samples and reveals rapid or slow exhumation events, even in cases of long stagnation of rocks at intermediate (2–6 km) depths. We used AFT thermochronology, which is sensitive to temperatures ranging from ~60 to ~110°C for cooling rates of about 4°C/Myr, which would correspond to a hold time of ~13 Myr within the AFT partial annealing zone (PAZ). Fission tracks are linear damage zones in the mineral lattice produced by the spontaneous fission of 238U, which accumulate and are preserved through time when the host mineral cools below the closure temperature, which is ~60–120°C for apatite (for details, see the references in Rolland et al. Reference Rolland, Bernet, van der Beek, Gautheron, Duclaux and Bascou2019). We measured AFT ages of collected Terre Adélie samples at the ISTerre (Université Grenoble Alpes, France) fission-track laboratory. The results are shown in Table I.
Table I. Apatite fission-track data.

a Fission-track age is given as central age. Samples were analysed dry with a BX-51 Olympus microscope at 1250× magnification. Central ages were calculated with the Binomfit program of M. Brandon (see Ehlers et al. Reference Ehlers, Chaudhri, Kumar, Fuller, Willett and Ketcham2005) using a ζ value of 283.52 ± 14.46. n = number of grains, ρs = spontaneous track density, n s = number of spontaneous tracks, ρI = induced track density, n i = number of induced tracks, ρd = track density of the dosimeter glass (CN 5), P (χ2) = probability obtaining χ2 value (χ2 test) for n degrees of freedom (where n is the number of crystals minus 1), TL = number of measured track lengths, MTL = mean horizontal confined track length, Dpar = mean etch pit diameter of fission tracks, where each etch pit diameter was averaged from four measurements per analysed grain.
Temperature-time modelling
In case of monotonic cooling, the closure temperature can be converted into depth after estimating the palaeogeothermal gradient; the obtained cooling rate can then be translated into an exhumation rate. However, a long episode of slow cooling and/or a long hold time within the PAZ will lead to shortening of tracks at temperatures as low as 60°C (see references in Rolland et al. Reference Rolland, Bernet, van der Beek, Gautheron, Duclaux and Bascou2019), and thus to a younging of the apparent cooling age. In this case, the age has no geological meaning, but the sample T-t history can be modelled based on the track-length distribution. The T-t cooling path can be used to infer the corresponding main exhumation phases and the potential phases of tectonic stagnation. Modelling of the T-t history was undertaken using HeFTy software based on the AFT data. The T-t HeFTy modelling results are displayed in Fig. 4 and Table II (for details, see the reference in Rolland et al. Reference Rolland, Bernet, van der Beek, Gautheron, Duclaux and Bascou2019).
Table II. Summary of HeFTy modelling results based on apatite fission-track (AFT) data.

Results are based on 10 000 model runs. The MTL values are given as c-axis projected track lengths. The AFT ages calculated by HeFTy are pooled ages. Initial track lengths are estimated from mean etch pit diameters of fission tracks with 5.5 M HNO3. The GOF refers to the modelling quality value ranked between 0 and 1.
GOF = goodness of fit, MTL = mean horizontal confined track length.
Terrestrial cosmogenic nuclide 10Be dating
Cosmogenic nuclide 10Be dating of moraine boulders (Figs S1 & S2) was applied to highlight the most recent Cenozoic erosion and glacier history on the moraine boulders dated by AFT. Approximately 500 g of each rock sample was crushed and sieved to obtain pure quartz. The beryllium extraction was carried out at ISTerre using a protocol detailed in Darnault et al. (Reference Darnault, Rolland, Braucher, Bourlès, Revel, Sanchez and Bouissou2012). The 10Be measurements were obtained at ASTER, the French National Accelerator Mass Spectrometry (AMS) facility located at the Centre Européen de Recherche et d'Enseignement en Géosciences de l'Environnement (CEREGE, Aix-en-Provence), following the methods and calibrations described in Darnault et al. (Reference Darnault, Rolland, Braucher, Bourlès, Revel, Sanchez and Bouissou2012). The AMS results were corrected from the process blank with 10Be:9Be ratios of (5.4 ± 0.7) × 1015. The results are shown in Table III and summarized in Table IV, and they are plotted in Fig. 5. Ages have been computed using the online CREp calculator (see Table III for details). No corrections for snow or erosion have been taken into account because of low and temporary snowfalls rapidly cleared by catabatic winds and insignificant erosion induced by permanent freezing. The sample thickness correction has been calculated with a 2.65 density factor.

Fig. 5. Probability density distribution of 10Be cosmogenic ages as a function of the exposure age (see Table III for details). Five age peaks are distinguished at ~30 ka, ~14.7 ka, ~10 ka, ~3 ka and ~570 yr. LSD = Lifton-Sato-Dunai scaling.
Table III. Terrestrial cosmogenic nuclide results of the Lacroix Nunatak (samples quoted as NL) and Cap Prud'homme moraines (samples quoted as 16DDU).
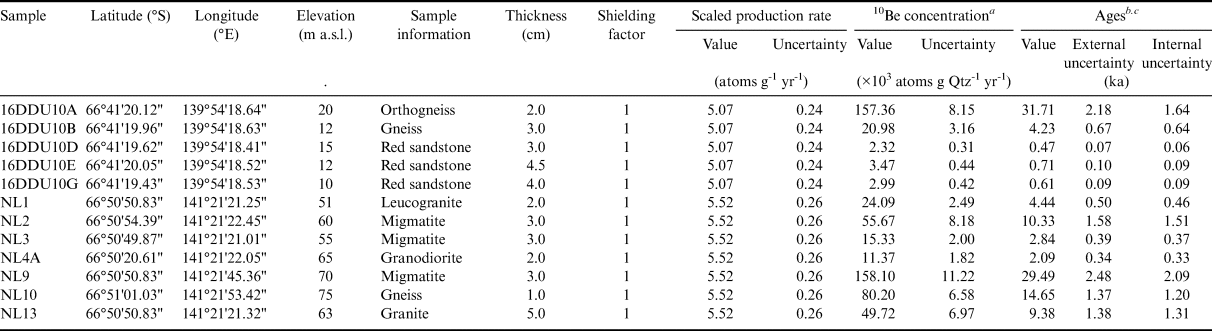
a Accelerator mass spectrometry (AMS) analyses have been carried out at the French AMS facility ASTER. The 10B concentrations were calibrated against the in-house Centre Européen de Recherche et d'Enseignement en Géosciences de l'Environnement (CEREGE) standard using the theoretical 10Be:9Be ratio of 1.191 × 10–11 and a 10Be half-life of 1.387 ± 0.012 106 years (Chmeleff et al. Reference Chmeleff, von Blanckenburg, Kossert and Jakob2010, Korschinek et al. Reference Korschinek, Bergmaier, Faestermann, Gerstmann, Knie and Rugel2010). Results have been corrected from the chemical blank (10Be:9Beblank = 5.39 ± 0.67 × 10–15). Propagated uncertainties include counting statistics, a conservative estimate of 0.5% for instrumental variability and the uncertainty of the standard deviation and chemical blank.
b Ages have been computed with the online CREp calculator using a worldwide production rate of 4.09 ± 0.19 at g-1 yr-1, the time-dependent scaling scheme Lal/Stone with ERA-40 and the geomagnetic record of Lifton (Reference Lifton2016).
c Internal uncertainties consider the analytical uncertainties, while the external uncertainties include ~4.5% uncertainty in the production rate and 8% uncertainty in the 10Be decay constant. The sample thickness correction has been calculated with a 2.65 density factor.
a.s.l. = above sea level.
Table IV. Summary of apatite fission-track (AFT) and cosmogenic nuclide dating results.

Undated samples are due to the absence or insufficient number of apatite (AFT) and quartz for 10Be dating.
Results
Apatite fission-track ages and T-t modelling
The moraine AFT ages obtained in this paper range from 230 to 345 Ma (Table I). We note that AFT ages of the Cap Prud'homme moraine samples are significantly older (335 ± 28 and 345 ± 17 Ma) than those of Lacroix Nunatak (248 ± 9 to 280 ± 17 Ma in samples NL1–6), apart from sample NL13 (330 ± 12 Ma).
The T-t modelling undertaken using fission-track lengths returned time-integrated T-t paths with a significant cooling in the Late Palaeozoic from > 150°C to ~50°C, in the range 350–300 Ma (Table II). Exhumation rates > 0.25 mm yr-1 are estimated for the 340–300 Ma time range, based on a standard continental geotherm in agreement with the cratonic nature of Terre Adélie. After this abrupt exhumation, which terminated at c. 300 Ma, the samples followed a more or less quiescent Mesozoic history. The T-t paths followed a slow exhumation < 0.005 mm yr-1 pattern during the Mesozoic until 40–30 Ma (Fig. 4). Regarding this very long stay at depths close to the apatite PAZ, the AFT ages should not be taken as geologically meaningful ages, and only the T-t models should be considered as representative of their thermal history. Finally, a small acceleration of cooling occurred since 40–30 Ma, which represents 1–2 km of exhumation in most samples.
Cosmogenic nuclide dating
The TCN 10Be ages obtained in the two moraines range from 31.8 to 0.47 ka and fall within five age groups (Fig. 5 & Table III), mostly spread after the Last Glacial Maximum (LGM): 1) a first age group at ~32 ka is represented by two samples, one sample from each moraine. The other TCN datings are post-LGM: 2) the oldest post-LGM age is of 14.7 ± 1.4 ka at Lacroix Nunatak. Following this age, three distinct Holocene age peaks are dated and at 3) 9.4–10.4 ka (n = 2), 4) 3.0 ± 0.2 ka (n = 4) and 5) 570 ± 48 yr (n = 3), respectively.
Discussion
Insights for the Late Palaeozoic to Mesozoic erosion history
As previously noted for basement samples dated by AFT along the Terre Adélie coast, the modelled T-t paths record a large amount of exhumation during Carboniferous times (Figs 4 & 6). The exhumation paths for the source regions of the two moraine samples are broadly similar to those of the Terre Adélie coast. Thus, this age span is slightly older than that of Permian rifting (e.g. Lisker & Olesch Reference Lisker and Olesch2003), and is more in agreement with that of the LPIA (e.g. Isbell et al. Reference Isbell, Henry, Gulbranson, Limarino, Fraiser and Koch2012). The LPIA started in the Late Devonian and extended until the early Late Permian (360–260 Ma), which represents the longest and most prominent glacial event in Earth's history (e.g. Qie et al. Reference Qie, Algeo, Luo and Herrmann2019). This glacial phase is ascribed to atmospheric CO2 drawdown or increased volcanism (Montañez et al. Reference Montañez, McElwain, Poulsen, White, DiMichele, Wilson and Hren2016). A sea-level drawdown of approximately -30 to -70 m is documented since 340 Ma, and peaked at 310–290 Ma (Rygel et al. Reference Rygel, Fielding, Frank and Birgenheier2008), which supports the hypothesis of an extensive polar ice sheet in this time range. The LPIA culminated during the Late Carboniferous, at 330–300 Ma, in the Southern Hemisphere (e.g. Isbell et al. Reference Isbell, Henry, Gulbranson, Limarino, Fraiser and Koch2012), when the South Pole was close to its present position (Fig. 2) (see discussions in Isbell et al. Reference Isbell, Henry, Gulbranson, Limarino, Fraiser and Koch2012). The similar AFT ages and cooling at 330–300 Ma obtained along the Terre Adélie coast and in the moraine boulders suggest a major erosion phase during the LPIA at the scale of East Antarctica (Fig. 3). Thermochronological studies undertaken around East Antarctica reveal similar cooling paths and ages (Arne Reference Arne1994, Fitzgerald Reference Fitzgerald1994, Lisker & Olesch Reference Lisker and Olesch2003). Taken together, these data are suggestive of a large ice cap that covered the whole East Antarctic continent in the Late Carboniferous. This is also supported by the erosion and transport of sediments in radial directions towards large peripheral glacial basins during the Permo-Carboniferous (Isbell et al. Reference Isbell, Henry, Gulbranson, Limarino, Fraiser and Koch2012, Rolland et al. Reference Rolland, Bernet, van der Beek, Gautheron, Duclaux and Bascou2019).

Fig. 6. Summary of time-temperature results using HeFTy modelling, with main tectonic events and glaciations of the Phanerozoic (Isbell et al. Reference Isbell, Henry, Gulbranson, Limarino, Fraiser and Koch2012 and references therein). The depth in kilometres or equivalent amount of exhumation is estimated based on a thermal gradient of 25°C km-1, in agreement with the crustal thickness of the Terre Adélie craton (Lamarque et al. Reference Lamarque, Barruol, Fontaine, Bascou and Ménot2015). APAZ = apatite partial annealing zone of fission tracks, LPIA = Late Palaeozoic Ice Age, P1–P4 = glaciation events occurring in Australia in the Permian (300–260 Ma).
Regional-scale Cenozoic erosion
Several aspects of the modelled T-t paths can be attributed to Cenozoic tectonics. The two Cap Prud'homme samples (16DDU10A and 10E) rapidly cooled to 20–30°C at c. 340 Ma, whereas the Lacroix Nunatak samples rapidly cooled to 50–60°C at between 350 and 300 Ma (Fig. 4). This difference is ascribed to a smaller Cenozoic erosion (< 1 km) in the source region of the dated Cap Prud'homme moraine samples than in that of the dated Lacroix Nunatak boulders (> 1.5–2.0 km) and of the Terre Adélie coast. Based on the current glacier flux maps (Fig. 3) (Rignot et al. Reference Rignot, Mouginot and Scheuchl2011), Cap Prud'homme appears to be positioned in a more frontal moraine position, whereas the Lacroix Nunatak moraine appears to be on the side of the glacial flux, positioned as a lateral moraine on the margins of some highlands (Fig. 3). During the Early Cenozoic, the significantly thinner ice sheet would preferentially drain through adjacent lowlands rather than the relatively highly elevated Terre Adélie craton (see Cox et al. Reference Cox, Thomson, Reiners, Hemming and van de Flierdt2010 for a similar discussion of other subglacial highlands in East Antarctica). Thus, one possible explanation would be that the Cap Prud'homme boulders are sourced from a higher-elevated area within the craton that may have been protected from high levels of erosion due to the presence of ice caps during the Cenozoic.
The glacier flux map suggests that moraine samples might have originated form a focused erosion loci located upstream of the Terre Adélie glacial flux (Fig. 3). Such narrow zones of focused channelized flow have been confirmed in the whole Antarctic ice sheet (e.g. Morlighem et al. Reference Morlighem, Rignot, Binder, Blankenship, Drews and Eagles2019). These narrow corridors could have been incised during the Mid-Pliocene warm period (e.g. Paxman et al. Reference Paxman, Jamieson, Hochmuth, Gohl, Bentley, Leitchenkov and Ferraccioli2019). These latter authors inferred a dynamic erosional environment based on the development of plateau features on the neighbouring Wilkes Subglacial Basin at c. 3.5 Ma. More samples would be necessary to test this hypothesis in Terre Adélie.
Cosmogenic nuclide dating of moraines reveals post-LGM glacier dynamics
The significance of the diverse TCN 10Be ages obtained in the two moraines, ranging from 31.8 to 0.47 ka (Fig. 4 & Table I), is still uncertain, as they might result from either distinct deglacial events or a combination of inheritance and a progressive Holocene deglacial process. Each moraine has to be considered separately to make a reasonable interpretation of this first batch of terrestrial cosmogenic ages (e.g. D'Arcy et al. Reference D'Arcy, Schildgen, Strecker, Wittmann, Duesing and Mey2019, Žebre et al. Reference Žebre, Sarıkaya, Stepišnik, Yıldırım and Çiner2019). Based on the most probable hypothesis that a moraine formed in a single deglacial stage (e.g. Putkonen & Swanson Reference Putkonen and Swanson2003, Heyman et al. Reference Heyman, Stroeven, Harbor and Caffee2011), we propose the following interpretation of our dataset:
• The Lacroix Nunatak moraine shows three ages spread between the Late Glacial to Early Holocene (14.7, 10.3 and 9.4 ka), which suggests a main deglaciation stage directly following the Younger Dryas cold event as elsewhere in Antarctica (e.g. Çiner et al. Reference Çiner, Yildirim, Sarikaya, Seong and Yu2019). Subsequently, it is most probable that the 29.5 ka age represents some inheritance from a previous deglacial stage, and is thus considered as not geologically meaningful and a minimum age for this previous event. By the same logic, the remaining boulders with ages ranging from 4.4 to 2.1 ka are considered as outliers, which may reflect a late exhumation process of these boulders. The fact that they show a relatively close cluster of values could be ascribed to a phase of increased ice depletion of the moraine related to a climatic cause.
• The Cap Prud'homme moraine shows a repartition of block ages that appears to be more random. Again, one age of 31.2 ka could be ascribed to inheritance as in Lacroix Nunatak. The other ages are much younger, with one of mid-Holocene age (4.2 ka) and all the rest being very young (0.5–0.7 ka). Therefore, one tentative interpretation could be that the main exhumation event is very recent one (c. 0.6 ka) for this moraine, and that the 4.2 and 31.2 ka ages represent some inheritance for the two blocks. Despite the fact that samples are just > 10 m above sea level, it is improbable that they could have been below sea level several ka ago, as a null vertical motion is measured by GPS in this area (Li et al. Reference Li, Ma, Zhang, Lei, Hao, Zhang and Li2020). Therefore, the young exhumation age of c. 0.6 ka could be ascribed to a complex exhumation dynamic of boulders in an ice matrix as reflected by the works of Tison et al. (Reference Tison, Petit, Barnola and Mahaney1993) and Genthon et al. (Reference Genthon, Lardeux and Krinner2007).
In conclusion, two main deglacial ages can be proposed from the two moraines: a significant early Holocene deglacial age at Lacroix Nunatak, most probably during the Younger Dryas (c. 11 ka) and a very young deglaciation age (c. 0.6 ka) in Cap Prud'Homme. Based on other evidence from offshore sediment cores and inland ice dynamics, we propose below a summary of possible evolution of the Terre Adélie coast since the LGM.
1) Based on the bathymetry maps compiled by Beaman et al. (Reference Beaman, O'Brien, Post and de Santis2011) and on the offshore sediment analysis of Domack et al. (Reference Domack, Jull, Anderson, Linick and Williams1989) and Crosta et al. (Reference Crosta, Debret, Denis, Courty and Ther2007), the palaeoglacier flow and maximum extent during the LGM are identified offshore of the Terre Adélie coast, where it reached at least the rim of the continental plateau (Figs 3 & 7c). This frontal LGM moraine thus stands at 80–130 km north of the current shoreline (Bentley et al. Reference Bentley, Cofaigh, Anderson, Conway, Davies and Graham2014). The orientation of basins suggests a dominant north-west-directed and episodic north-directed glacial flux during the LGM (Fig. 7).
2) Based on the age of the Lacroix Nunatak moraine, the oldest post-LGM age is 14.7 ± 1.4 ka at Lacroix Nunatak. However, with only one age, this sample could still reflect some partial inheritance and still needs confirmation. This age is similar to the main deglaciation age of meltwater pulse 1A ('MWP-1A'), which coincides with an abrupt deglacial warming recorded at Taylor Dome at ~14.6 ka (Fig. 7a) (Steig et al. Reference Steig, Morse, Waddington, Stuiver, Grootes and Mayewski2000) at the latitude of the potential glacial sources of the Terre Adélie glaciers. Similar ages are recorded by Balco et al. (Reference Balco, Todd, Goehring, Moening-Swanson and Nichols2019) in neighbouring Victoria Land and correlate with a rapid eustatic sea-level rise and deglaciation at higher latitudes. This is expressed both at South Hemisphere mid-latitudes and at North Hemisphere mid- to high latitudes, such as in the Alps (e.g. Darnault et al. Reference Darnault, Rolland, Braucher, Bourlès, Revel, Sanchez and Bouissou2012).
Following this age, three distinct Holocene-age peaks are dated as follows:
3) The first group is dated at 9.4–10.4 ka. These two Early Holocene ages at 9.4 ± 1.4 ka and 10.4 ± 1.6 ka correspond to the age of the Lacroix Nunatak moraine and may correspond to the deglaciation stage directly following the Younger Dryas cold event (e.g. Çiner et al. Reference Çiner, Yildirim, Sarikaya, Seong and Yu2019). This 10–11 ka age corresponds to the onset of an increased glacial flux, with rates > 4 cm ice yr-1 recorded at the Taylor Dome ice core (Fig. 7a) (Steig et al. Reference Steig, Morse, Waddington, Stuiver, Grootes and Mayewski2000). This reflects a change in climate dynamics for the EAIS, as also suggested by the marine records off the Terre Adélie coast (Bentley et al. Reference Bentley, Cofaigh, Anderson, Conway, Davies and Graham2014). This climatic phase is well expressed at the scale of the Antarctic continent (e.g. Çiner et al. Reference Çiner, Yildirim, Sarikaya, Seong and Yu2019), and is also largely documented at mid-latitudes (e.g. Darnault et al. Reference Darnault, Rolland, Braucher, Bourlès, Revel, Sanchez and Bouissou2012).
4) The second group of Holocene ages is recorded in four samples from the two moraines, spanning between 4.4 ± 0.5 and 2.1 ± 0.3 ka. This age range correlates with a phase of deglaciation that closely follows a stage of glacier re-advance at c. 5 ka (Steig et al. Reference Steig, Morse, Waddington, Stuiver, Grootes and Mayewski2000), most probably due to increased precipitation during the Holocene climate optimum. We propose that this climatic event might have led to the exhumation of moraine blocks.
5) Finally, the last Holocene age peak of 570 ± 48 yr is represented by three ages of the Cap Prud'homme moraine ranging between 470 ± 70 and 710 ± 100 yr. These ages are within the Little Ice Age (LIA) range, which is defined from ~1300 to 1850 yr ce. These latter ages are most probably ascribed to very recent deglaciation of boulders given the field situation (Fig. S1), with some inherited component. It might also reflect an accelerated retreat of the glacier tongue since the end of the LIA, which is in agreement with the observations of DDU in 1840, who described a glacier front at ~20 km north of its present position (Godard et al. Reference Godard, Reynes, Bascou, Ménot and Palmeri2017).

Fig. 7. a. The 10Be cosmogenic nuclide dating results (this paper) and post-Last Glacial Maximum (LGM) climatic model reconstructed for the Terre Adélie-George V Land domain from Steig et al. (Reference Steig, Morse, Waddington, Stuiver, Grootes and Mayewski2000). b. Variations of ice accumulation rate at the Taylor Dome since 16 ka (Steig et al. Reference Steig, Morse, Waddington, Stuiver, Grootes and Mayewski2000). c. George V digital elevation model bathymetric map of the Terre Adélie-George V Land coast and perspective view (towards the east) of the Terre Adélie coast, showing the prominence of the Adélie Bank, modified after Beaman et al. (Reference Beaman, O'Brien, Post and de Santis2011). The locations of the investigated moraines are indicated by yellow stars (CP = Cap Prud'homme, LN = Lacroix Nunatak). The direction of flow of glaciers during the LGM is indicated by the blue arrows and the frontal glacier grounding line during the LGM is shown by the bold dashed lines. The major glacial pathway for the Dumont D'Urville (DDU) area is towards the north-west along the Adélie Basin. Sediment deposits off the Adélie Bank suggest episodic glacial transport towards the north over the Adélie Bank during the LGM. LIA = Little Ice Age.
Considerations regarding the obtained 10Be ages from the perspective of future works
Most of the East Antarctic basement stands at elevations of < 0 m between 100 and 160°E (Fig. 1). This portion of the continent is potentially the most sensitive to sea-level fluctuations induced by the response of the EAIS to rising sea temperature, which is a central parameter in controlling the evolution of the ice cap. In this context, palaeoproxy records and long-term observational datasets are underused resources in terms of strategies for improving Antarctic climate projections for the twenty-first century and beyond (Bracegirdle et al. Reference Bracegirdle, Colleoni, Abram, Bertler, Dixon and England2019). Documenting the response of the EAIS to the environmental conditions that led to the deglaciation after the LGM will provide a basis for understanding and modelling its future response to climate warming over the coming centuries. The ice sheet was generally more extensive than was present during the global LGM (27–20 ka BP; Clark et al. Reference Clark, Dyke, Shakun, Carlson, Clark and Wohlfarth2009). Based on the sparse and mostly marine records along the East Antarctic coast, previous studies suggested that the EAIS responded with a delay of c. 6–8 ka to the increasing temperatures after the LGM (Bentley et al. Reference Bentley, Cofaigh, Anderson, Conway, Davies and Graham2014). As suggested by marine records, the EAIS response to deglaciation may have followed a three-stage model (Bentley et al. Reference Bentley, Cofaigh, Anderson, Conway, Davies and Graham2014):
1) Onset of ice sheet retreat as early as 18 ka bp in the Lambert/Amery system, perhaps due to an enhanced sensitivity related to a rapid post-glacial sea-level rise at that time (Golledge et al. Reference Golledge, Fogwill, Mackintosh and Buckley2012).
2) Retreat at some sites at 14 ka bp, coinciding with MWP-1a, during which the global sea level rose by between 16 and 25 m in ~400–500 years. However, Bentley et al. (Reference Bentley, Cofaigh, Anderson, Conway, Davies and Graham2014) see a small contribution of the Antarctic to the sea-level rise during MWP-1A.
3) The majority of ice-sheet retreat occurred at the onset of the Holocene (12 ka bp) in response to oceanic warming (Bentley et al. Reference Bentley, Cofaigh, Anderson, Conway, Davies and Graham2014) and coinciding with the Early Holocene warm period recorded in coastal ice cores and lake sediment records (Verleyen et al. Reference Verleyen, Hodgson, Sabbe, Cremer, Emslie and Gibson2011). This seems to be in disagreement with the inner EAIS glacial record of Taylor Dome (Fig. 7a) (Steig et al. Reference Steig, Morse, Waddington, Stuiver, Grootes and Mayewski2000), which may indicate that the EAIS responded more rapidly to MWP-1A than is suggested by the marine records. However, in accordance with the marine records, it seems that the Holocene deglacial signal is the most expressed from our 10Be TCN age dataset. Erosion of some of the former glacial remains during the Younger Dryas re-advance may also have hidden part of the moraine record.
Therefore, a more precise establishment of the EAIS behaviour in relation to climate change since the LGM requires further testing through measurement of past glacial extensions onshore of East Antarctica, especially in Terre Adélie. Our preliminary dataset shows that the 10Be dating technique is appropriate for this goal. The combined use of thermochronology on the same boulders may allow us to infer the domains preferentially eroded during each of the climatic phases, which may be used as a proxy of subglacial erosion dynamics over the long term, but this requires a large number of dated samples and a detailed geomorphological analysis of moraines.
Conclusion
Combined thermochronology and cosmogenic nuclide analysis of moraine blocks is shown to be a powerful tool for investigating both the past (Phanerozoic) and recent (Cenozoic-Quaternary) erosion history of hidden Antarctica. The AFT dating of moraine blocks in Terre Adélie seems to be in agreement with a major phase of (hot-based) glacial erosion during the LPIA between 350 and 300 Ma and a null erosion-exhumation during the Mesozoic. Furthermore, a moderate and focused local Cenozoic erosion (< 1.5–2.0 km) is recorded in samples both several hundreds of kilometres upstream and along the coast of the Terre Adélie craton, which is ascribed to a low level of erosion of the East Antarctic margin since the establishment of the polar ice sheet. This low level of erosion is possibly due to the omnipresence of cold-based glaciers since 34 Ma. The geometry of the glacial flux is suggestive of the development of over-deepening in narrow corridors in relation to the mid-Pliocene warm period some 400–500 km upstream of the investigated moraines. The 10Be cosmogenic nuclide surface exposure ages of the moraine boulders indicate a major deglaciation after the Younger Dryas, which might have commenced at c. 14.7 ka and become significantly amplified after 10 ka. The timing of retreats is found to be similar to phases observed in the Northern Hemisphere and at mid- to high latitudes.
Acknowledgements
We warmly thank F. Coeur and F. Senebier (GeoThermoChronology Platform, ISTerre) for mineral separation support and K. Kedadouche and G. Aumaitre for TCN analysis. C. Ritz and P. Valla are thanked for discussions. In addition, this manuscript was significantly enhanced following the constructive comments of A. Çiner and an anonymous reviewer.
Author contributions
YR conceived the study and wrote the paper. EV took part in the AFT and cosmogenic nuclide dating analyses during his Master's internship. MB supervised the AFT datings, prepared the samples and checked all of the results. JC supervised the cosmogenic datings. JB and CS assisted in field sampling. R-PM and GD provided support regarding the artwork and expertise in Antarctic geology.
Financial support
This work was supported by a LABEX grant from Université Grenoble Alpes and ISTerre. The Institut Paul-Émile Victor supported numerous field seasons in Terre Adélie through the Géologie du Craton de Terre Adélie (GEOLETA) and Architecture de la Lithosphère de la Terre Adélie (ARLITA) programmes.
Details of data deposit
The data can be retrieved at https://hal.archives-ouvertes.fr.
Supplemental material
Two supplemental figures will be found at https://doi.org/10.1017/S095410202000036X.