Introduction
Ascaris lumbricoides is the most prevalent helminth causing human disease, recording nearly 500 million cases a year in subtropical and tropical regions globally (Prevention, 2015; Disease, 2020) (Fig. 1). Ascariasis is an infection of poverty with high prevalence in low- and middle-income countries (LMICs) in Africa, Latin America, South East Asia and South Asia (Disease, 2020). However, ascariasis has also been reported in poverty-stricken regions within high-income countries (HICs) including the United States.
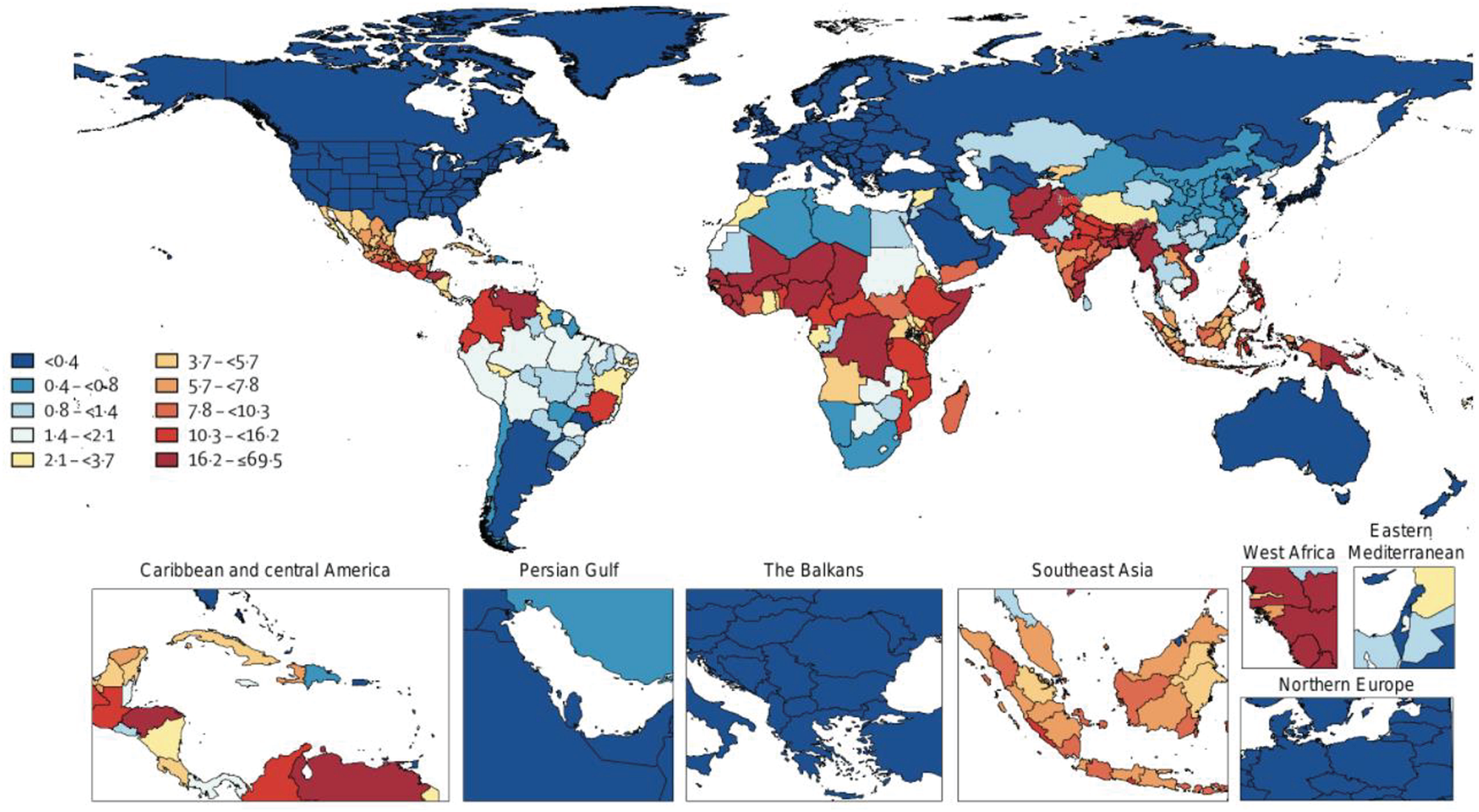
Fig. 1. Geographic distribution of Ascaris-associated DALYs per 100 000 from the Global Burden of Disease Collaborative Network, 2019. Institute for Health Metrics and Evaluation (IHME), Seattle, United States, 2020 (http://www.healthdata.org/results/gbd_summaries/2019/ascariasis-level-4-cause).
Historic reports have cited prevalence as high as 49.4% in school-aged children in the Southeast United States. However, in more recent years, the lack of robust epidemiologic monitoring within HICs has limited the current understanding of on-going transmission in these regions (Starr and Montgomery, Reference Starr and Montgomery2011). Similar to other helminth infections, Ascaris spp. infects people living in extreme poverty, with insufficient waste management and inadequate sanitation measures, which allows for ubiquitous contamination of the environment with infectious Ascaris spp. eggs. Subsequent infection leads to long-term morbidity, which limits school achievement and job productivity, leading to a cycle of poverty within the community. The social and economic consequences of ascariasis at both the individual and community-level can be devastating.
Children harbour the highest disease prevalence and the highest disease burden of Ascaris infection (Disease, 2020). Ascaris burden peaks in pre-school and school-aged children before gradually tapering off into adulthood. The reasons behind the predilection for children remain largely unknown but may reflect both behavioural adaptations as well as acquired immunity as children age into adulthood (Weatherhead and Hotez, Reference Weatherhead and Hotez2015). Immunity to Ascaris occurs after repeated infection; however, this immunity is only partial and does not prevent against re-infection leading to persistent, but reduced disease burden in adulthood (Colombo and Grencis, Reference Colombo and Grencis2020). Approximately 2090 deaths occur annually as a result of ascariasis globally, with the highest death rates occurring in children aged 1–4 years old (Disease, 2020). Despite the lower mortality rates compared to other infectious pathogens, ascariasis causes significant morbidity, which can be measured by disability-adjusted life years (DALYs). Ascariasis alone accounts for over 750 000 DALYs a year, with disability peaking in children 5–9 years old (Disease, 2020) (Fig. 2).

Fig. 2. Years of life lost (YLL) and years of healthy life lost to disability (YLD) from ascariasis globally from the Global Burden of Disease Collaborative Network, 2019. Institute for Health Metrics and Evaluation (IHME), Seattle, United States, 2020 (http://www.healthdata.org/results/gbd_summaries/2019/ascariasis-level-4-cause).
The high rate of disability, particularly in young children, highlights the critical need for novel interventions to prevent life-long health consequences in Ascaris endemic regions.
Pathogenesis
Ascaris spp. is transmitted from human-to-human through the fecal–oral transmission route. Embryonated eggs found in contaminated environments (i.e. soil and homes) are ingested. Infective stage 3 larvae (L3) hatch from the eggs, penetrate the intestinal mucosa and enter the circulatory system. The larvae are carried via host blood from the liver to the lungs. While in the lungs, the larvae undergo considerable growth and alter their transcriptomic signature. Subsequently, the lung L3 reach the airways, ascend the bronchotracheal tree, are swallowed and enter back into the host small intestines completing the larval migratory cycle, as fully developed L4 stages. The larvae develop into large macroscopic adult worms, measuring up to 35 cm in length, within the intestinal lumen (Prevention, 2015). Adult worms subsequently live for 1–2 years within the host. Although adult female worms shed up to 400 000 eggs per day into the host feces, the eggs must embryonate within, preferably, moist, warm soil prior to becoming infectious (Prevention, 2015). The dependency on this soil stage requirement for completion of the life cycle is why ascariasis, in addition to trichuriasis and hookworm infection, is termed a soil-transmitted helminth (STH).
Ascaris suum, the porcine roundworm, can also cause human infection. The life cycle of both A. lumbricoides and A. suum can be completed in both humans and pigs. Both organisms can be transmitted from human to human, without direct pig exposure (Da Silva Alves et al., Reference Da Silva Alves, Conceição and Leles2016). As a result, outbreaks of A. suum infection in humans have occurred globally, including in HICs (Miller et al., Reference Miller, Colby, Manning, Hoenig, McEvoy, Montgomery, Mathison, de Almeida, Bishop, Dasilva and Sears2015). Ascaris lumbricoides and A. suum are morphologically indistinguishable with minimal genetic variance leading to an on-going debate if they are indeed separate species (Leles et al., Reference Leles, Gardner, Reinhard, Iñiguez and Araujo2012; Zhou et al., Reference Zhou, Chen, Niu, Ouyang and Wu2020). Sequencing of the mitochondrial genomes as well as miRNA profiles have shown a high degree of relatedness between A. lumbricoides and A. suum (Liu et al., Reference Liu, Wu, Song, Wei, Xu, Lin, Zhao, Huang and Zhu2012; Shao et al., Reference Shao, Xu, Alasaad, Song, Peng, Tao and Zhu2014). Both A. lumbricoides and A. suum share an A and T nucleotide bias in their genomes with most genetic differences leading to synonymous mutations (Liu et al., Reference Liu, Wu, Song, Wei, Xu, Lin, Zhao, Huang and Zhu2012; Da Silva Alves et al., Reference Da Silva Alves, Conceição and Leles2016). Gene sequencing demonstrates tight phylogenetic clustering, further suggesting shared ancestry (Liu et al., Reference Liu, Wu, Song, Wei, Xu, Lin, Zhao, Huang and Zhu2012). While A. lumbricoides and A. suum may be the same organism, at minimum they both represent significant zoonotic potential. As such, elimination strategies should be formulated with a ‘One Health’ approach, targeting human and animal reservoirs to impede transmission between human and animal populations (Betson and Stothard, Reference Betson and Stothard2016; Da Silva Alves et al., Reference Da Silva Alves, Conceição and Leles2016).
Clinical manifestations
Ascariasis can cause a spectrum of diseases from asymptomatic to severe malnutrition, impairment in cognitive development and growth restriction (Ezeamama et al., Reference Ezeamama, Friedman, Acosta, Bellinger, Langdon, Manalo, Olveda, Kurtis and McGarvey2005; Rajagopal et al., Reference Rajagopal, Hotez and Bundy2014; Pabalan et al., Reference Pabalan, Singian, Tabangay, Jarjanazi, Boivin and Ezeamama2018). Morbidity is associated with the acute larval migration stage as well as the chronic adult gastrointestinal stage. During acute larval migration through the lungs, Ascaris larvae stimulate an eosinophilic pneumonitis termed Loeffler's syndrome. Long-term consequences of larval migration through the lungs include asthma and potentially chronic lung disease. Animal studies have shown that mice infected with Ascaris spp. can have prolonged allergic airway phenotypes (Nogueira et al., Reference Nogueira, Gazzinelli-Guimarães, Barbosa, Resende, Silva, de Oliveira, Amorim, Oliveira, Mattos, Kraemer, Caliari, Gaze, Bueno, Russo and Fujiwara2016; Gazzinelli-Guimaraes and Nutman, Reference Gazzinelli-Guimaraes and Nutman2018; Weatherhead et al., Reference Weatherhead, Porter, Coffey, Haydel, Versteeg, Zhan, Gazzinelli Guimarães, Fujiwara, Jaramillo, Bottazzi, Hotez, Corry and Beaumier2018). Furthermore, wheezing episodes in Ascaris endemic regions are commonly attributed to allergic sensitization to Ascaris (Chico et al., Reference Chico, Vaca, Rodriguez and Cooper2019). Ascaris infection may also contribute to long-term lung disease, exacerbating underlying lung pathology and aggravating pulmonary dysfunction. However, more information is needed to clearly understand the long-term consequences of larval migration through the host lung tissue (Oliveira et al., Reference Oliveira, da Paixão Matias, Kraemer, Gazzinelli-Guimarães, Santos, Amorim, Nogueira, Freitas, Caliari, Bartholomeu, Bueno, Russo and Fujiwara2019). Chronic intestinal ascariasis is associated with gastrointestinal symptoms including nausea, vomiting and diarrhoea. Individuals with high worm burden, particularly young children, are at high-risk of intestinal obstruction and intestinal perforation (de Silva et al., Reference de Silva, Guyatt and Bundy1997); Mishra et al., Reference Mishra, Agrawal, Joshi, Sanghvi, Shah and Parelkar2008). Additionally, adult intestinal worms can move into the biliary ductal system causing hepatobiliary disease, including pancreatitis and cholecystitis (Jourdan et al., Reference Jourdan, Lamberton, Fenwick and Addiss2018).
Current management strategies
The significant impact on childhood morbidity and life-long chronic disease has led to the development of global partnerships to reduce Ascaris worm burden and to target disease control and elimination. Introduction of preventive chemotherapy through mass drug administration (MDA) programmes provides regular, repeated administration of a single-dose tablet of albendazole or mebendazole (benzimidazoles) to high-risk groups based on local disease prevalence. The goal of MDA programmes is to reduce worm burden in order to reduce morbidity (Weatherhead et al., Reference Weatherhead, Hotez and Mejia2017; Weatherhead, Reference Weatherhead2019). Preventive chemotherapy is provided on an annual or biannual basis in young children (12–23 months of age), pre-school-aged children (24–59 months of age), school-aged children and women of child-bearing age living in endemic regions with prevalence >20% (World Health Organization, 2017). Regular preventive chemotherapy, known as deworming, has been associated with an increase in weight and height in children (World Health Organization, 2017). Expansion of preventive chemotherapy MDA programmes to provide therapy more frequently and/or to a broader range of the community may provide additional power to interrupt transmission dynamics in endemic regions. However, frequent and widespread use of anthelminthic drugs is associated with the development of anthelminthic resistance (Farrell et al., Reference Farrell, Coffeng, Truscott, Werkman, Toor, de Vlas and Anderson2018). Mutations in the beta-tubulin isotype-1 gene have been shown to induce resistance in many nematodes, including Ascaris, to benzimidazole anthelminthic therapy. Although the frequency of this mutation for Ascaris spp. currently remains low in humans, heightened anthelminthic pressure in a community can enhance resistance development (Furtado et al., Reference Furtado, Medeiros, Zuccherato, Alves, de Oliveira, da Silva, Miranda, Fujiwara and Rabelo2019). Thus, MDA scale-up strategies to eliminate Ascaris are likely to create additional hurdles. Introduction of water, sanitation and hygiene (WASH) programmes may also benefit Ascaris control efforts. Implementation of sanitation standards, frequent handwashing policies and access to clean water can significantly reduce Ascaris infection within endemic communities (Strunz et al., Reference Strunz, Addiss, Stocks, Ogden, Utzinger and Freeman2014). As a result, WASH programmes continue to play a critical role in developing sustainable control efforts. However, the effects of WASH programmes are not immediate and require long-term funding (Vaz Nery et al., Reference Vaz Nery, Pickering, Abate, Asmare, Barrett, Benjamin-Chung, Bundy, Clasen, Clements, Colford, Ercumen, Crowley, Cumming, Freeman, Haque, Mengistu, Oswald, Pullan, Oliveira, Einterz Owen and Brooker2019). The implementation of MDA policies and WASH programmes has led to a modest reduction in disease burden and overall morbidity globally over the past decade. From 2010 to 2019, DALYs associated with ascariasis decreased by 17.4% (Disease, 2020). Despite the reduction of disease burden, control and eradication is unlikely to be achieved using MDA and WASH programmes alone.
Ascaris eggs are hardy, difficult to eradicate and remain viable in the environment for years (Jourdan et al., Reference Jourdan, Lamberton, Fenwick and Addiss2018). People living in endemic regions are repeatedly exposed to Ascaris eggs directly from their environment, leading to re-infection. Individuals commonly become re-infected as early as 3 months post treatment. By 12 months post-treatment there is a 95% re-infection rate in communities with high Ascaris prevalence, providing further evidence that the current eradication strategies are insufficient (Jia et al., Reference Jia, Melville, Utzinger, King and Zhou2012). In the setting of high rates of re-infection and the potential for drug resistance development, preventive and/or therapeutic vaccine technologies will be essential for disease eradication. To date, Ascaris vaccine progression has been limited, but new platforms to identify vaccine targets may provide innovative avenues in vaccine discovery.
The complex Ascaris–host immune response interface as the basis for vaccine development
The host immune response to Ascaris is parasite developmental-stage specific, leading to either inflammation or immunomodulation. Larval migration through the host is an essential survival phase of Ascaris. Attempts to control and eradicate the larvae by the host are dependent on a coordinated innate and adaptive immune response. Antigens from the larvae or from their secreted products, excretory secretory (ES) products or extracellular vesicles (EVs) cause local damage during larval migration. The acute damage to host tissue induces release of alarmins, interleukin (IL)-25, thymic stromal lymphopoietin and IL-33, that promote activation and regulation of innate cells including neutrophils, eosinophils, type 2 innate lymphoid cells and alternatively activated macrophages as well as CD4+ type 2 helper cells (Th2). This polarized type-2 immune response leads to high concentrations of IL-4, IL-5, IL-9 and IL-13 (Cooper et al., Reference Cooper, Chico, Sandoval, Espinel, Angel, Kennedy, Urban, Griffin and Nutman2000; Gazzinelli-Guimaraes and Nutman, Reference Gazzinelli-Guimaraes and Nutman2018; Weatherhead et al., Reference Weatherhead, Gazzinelli-Guimaraes, Knight, Fujiwara, Hotez, Bottazzi and Corry2020). Type-2 cytokines cause hypercontractility of smooth muscle, goblet cell hyperplasia, mucous production, influx of immune cells such as eosinophils and isotype switching to immunoglobulin E (IgE) that aids in parasite killing and expulsion in early infection. However, once larvae reach the intestinal lumen and develop into adult worms, they create an immunomodulatory environment. Adult Ascaris ES products contain immune mimicry markers such as transforming growth factor-β that activate regulatory T cells (Treg), creating a more immune tolerate environment and allowing for chronic long-standing intestinal infection (Gazzinelli-Guimaraes and Nutman, Reference Gazzinelli-Guimaraes and Nutman2018).
Ideally, the goal of an Ascaris vaccine development programme is to identify vaccine targets that mimic the host immune response elicited during natural infection while avoiding the long-term morbidity that occurs with natural infection. Currently, immune-mediated larval killing and/or expulsion mechanisms serve as the major immunologic basis for Ascaris vaccine development. Ascaris re-infection studies using experimental animal models have been instrumental in understanding stage-specific, immune-mediated larval killing mechanisms. There are likely two major host immune sites that contribute to the host immune barrier against ascariasis, the post-hepatic lungs and intestines and the pre-hepatic intestines, that could be targeted for vaccine development purposes (Magalhães et al., Reference Magalhães, Nogueira, Gazzinelli-Guimarães, Oliveira, Kraemer, Gazzinelli-Guimarães, Vieira-Santos, Fujiwara and Bueno2021). The post-hepatic lungs serve as an immune barrier to larval migration by immune-mediated larval killing mechanisms. Using a mouse model, a partial protective immune response associated with larval killing can be developed with repeated infection, reducing the parasite burden in the lungs by 72–90% as well as reducing the associated clinical morbidity (Urban, Alizadeh and Romanowski, Reference Urban, Alizadeh and Romanowski1988; Masure et al., Reference Masure, Wang, Vlaminck, Claerhoudt, Chiers, Van den Broeck, Saunders, Vercruysse and Geldhof2013b; Nogueira et al., Reference Nogueira, Gazzinelli-Guimarães, Barbosa, Resende, Silva, de Oliveira, Amorim, Oliveira, Mattos, Kraemer, Caliari, Gaze, Bueno, Russo and Fujiwara2016). Multiple exposures of mice to A. suum lead to a significant increase in cellularity in the lung tissue and airways, characterized by an increase in lymphocytes, M2-macrophages and eosinophils. Pulmonary eosinophils have specifically been associated with larval killing and prohibition of larval development in the lungs (Nogueira et al., Reference Nogueira, Gazzinelli-Guimarães, Barbosa, Resende, Silva, de Oliveira, Amorim, Oliveira, Mattos, Kraemer, Caliari, Gaze, Bueno, Russo and Fujiwara2016; Gazzinelli-Guimaraes et al., Reference Gazzinelli-Guimaraes2019). Additionally, Nogueira et al. (Reference Nogueira, Gazzinelli-Guimarães, Barbosa, Resende, Silva, de Oliveira, Amorim, Oliveira, Mattos, Kraemer, Caliari, Gaze, Bueno, Russo and Fujiwara2016) demonstrated that pulmonary-associated protection generated by Ascaris re-infection was associated with a systemic mixed Th2/Th17 immune response. Additionally, the post-hepatic intestine also provides protective immunity relying on activation of gut motility to create a prohibitive environment for Ascaris larval to develop into adult worms. Using a pig model at the time which L4 are becoming established in the pig intestine and transitioning into adult worms, gastric transit time is significantly decreased leading to larval expulsion (Masure et al., Reference Masure, Wang, Vlaminck, Claerhoudt, Chiers, Van den Broeck, Saunders, Vercruysse and Geldhof2013b). Intestinal expulsion is likely driven by STAT-6 dependent upregulation of type-2 cytokines IL-4 and IL-13, leading to increased luminal fluids (weep) and muscle contractility (sweep). The weep and sweep mechanism makes the intestinal lumen an inhospitable environment for the helminth parasite to develop (Anthony et al., Reference Anthony, Rutitzky, Urban, Stadecker and Gause2007). Pre-hepatic immunity in the intestines can also develop after repeated infection. Masure et al. (Reference Masure, Vlaminck, Wang, Chiers, Van den Broeck, Vercruysse and Vercruysse2013a, Reference Masure, Wang, Vlaminck, Claerhoudt, Chiers, Van den Broeck, Saunders, Vercruysse and Geldhof2013b) demonstrated that pigs continually exposed to infective A. suum eggs develop an almost sterilizing immunity (99.7% reduction in number of larvae) (Masure et al., Reference Masure, Vlaminck, Wang, Chiers, Van den Broeck, Vercruysse and Vercruysse2013a). The generated immunity was associated with eosinophilia, mastocytosis and goblet cell hyperplasia in the caecum suggesting the pre-hepatic intestinal mucosa provide a primary barrier to invading larvae. Targeting host immune-mediated larval killing and/or immune-mediated expulsion in animal models has provided significant insight into Ascaris vaccine development pathways. Identification and use of immunogenic Ascaris antigens for vaccine development that enhance larval killing and/or larvae expulsion will prevent the maturation of Ascaris larvae into adult worms and, in effect, prevent chronic infection.
Animal models used for Ascaris vaccine development
Several animal models have been used to identify and evaluate Ascaris vaccine targets, including guinea pigs, rabbits, chicken (Ascaridia galli), mice, piglets and pigs. Although pigs are a natural host and are competent models for both the larval migration phase and chronic adult worm phase, their use in vaccine development is limited by the physical and financial challenges of working with and housing larger animals. As a result, mouse models have evolved as the primary in vivo animal system used in the development and evaluation of Ascaris vaccines. However, although mouse models do support the completion of the larval migration phase, the model does not support the development of adult intestinal worms, limiting its usefulness (Lewis et al., Reference Lewis, Behnke, Stafford and Holland2005). Embryonated Ascaris eggs, classically A. suum, are given to mice by oral gavage and larval migration proceeds along the same pathway as described in humans but at an accelerated time scale; larval burden peaks in the mouse lungs at day 8 post infection and larvae are evacuated from the host on day 14 post infection (Gazzinelli-Guimarães et al., Reference Gazzinelli-Guimarães, Gazzinelli-Guimarães, Silva, Mati, Dhom-Lemos, Barbosa, Passos, Gaze, Carneiro, Bartholomeu, Bueno and Fujiwara2013). Susceptibility of mice to Ascaris eggs vary based on the genetic background of the mouse species. C57BL/6j, which generally have a polarized type-1 immune response, are more susceptible to Ascaris larval migration marked by high worm burdens in the lungs compared to other strains, including Balb/c and CBA mice, which are more resistant to larval migration (Lewis et al., Reference Lewis, Behnke, Stafford and Holland2005, Reference Lewis, Behnke, Cassidy, Stafford, Murray and Holland2007). Resistant strains, however, have been found to have more prolific immune responses, particularly in the lungs, with elevated concentrations of neutrophils, lymphocytes and eosinophils and type-2 cytokines compared to susceptible strains (Lewis et al., Reference Lewis, Behnke, Cassidy, Stafford, Murray and Holland2007). Mouse models remain the major mechanism for Ascaris vaccine development because of their ease of use and the ability to evaluate the impact of vaccines on the larval migration cycle, the over-arching goal of Ascaris vaccine development programmes.
Historical review of Ascaris vaccine development and lead candidates
Decades of scientific literature indicate that efficient, protective immunity against nematodes, including parasites from the order Ascaridida, can be induced using extracts and suspensions of living and dead parasites (Sprent and Chen, Reference Sprent and Chen1949; Soulsby, Reference Soulsby1957). However, the results of these studies are complicated by non-specific immunity and uncontrolled clinical consequences limiting their use. But, technological advancements in the field have allowed for expansion beyond whole extracts or parasites to engage a more sophisticated, standardized and specific vaccine-induced immunity. A historical overview highlighting the parasite targets, vaccine types, animal models, protocols and major findings (Table 1) helps guide the discussion on how Ascaris vaccine technology has evolved and what could occur in the future (Fig. 3).

Fig. 3. Timeline of Ascaris targets and animal models used in the Ascaris vaccine development process. To date there have been no vaccine candidates that have moved from pre-clinical animal models into human clinical trials. Created with BioRender.com.
Table 1. Historical and systematic summary of the pre-clinical trials on Ascaris vaccine

*reduction of parasite burden; s.c., subcutaneous route; i.v., intravenous route; i.p., intraperitoneal route; i.m., intramuscular route; i.n., intranasal; p.o., per os; ES, excretory-secretory; EP, isolated from Ascaris suum eggs; L2,3ESP, L2 developing in vitro to L3; L3,4ESP, L3 developing in vitro to L4; #data not shown; CTB, cholera toxin B; FCA, Freund's complete adjuvant; FIA, Freund's incomplete adjuvant; CytRx, TiterMax Gold adjuvant; MPLA, monophosphoryl lipid A; CT, cholera.
In the 1950s and early 1960s, crude extract from different developmental stages of Ascaris was used in vaccine pre-clinical trials as either a positive control for protection or as a tool to elucidate the immunological mechanisms of protective immunity (Gazzinelli-Guimarães et al., Reference Gazzinelli-Guimarães, Gazzinelli-Guimarães, Nogueira, Oliveira, Barbosa, Amorim, Cardoso, Kraemer, Caliari, Akamatsu, Ho, Jones, Weatherhead, Bottazzi, Hotez, Zhan, Bartholomeu, Russo, Bueno and Fujiwara2018). Subsequently, standardized pre-clinical trials in guinea pig models demonstrated that immunizations with the crude extract of Ascaris eggs or larvae provide a 28–77% larval reduction in infectious challenge models, indicating a relative protection and potential use of Ascaris antigens as vaccine targets (Soulsby, Reference Soulsby1957; Taffs, Reference Taffs1960). In the late 1970s and early 1980s, ultraviolet-attenuated (UV) parasite models, achieved by feeding pigs UV-irradiated fully embryonated eggs, were used to elicit protection. Tromba (Reference Tromba1978) demonstrated that pigs that were fed eggs attenuated by short-wave UV radiation developed resistance to challenge infection. Corroboratively, Urban and Tromba (Reference Urban and Tromba1982) showed that oral inoculation of pigs with 10 000 eggs irradiated by exposures of 150, 100 and 75 μW-min cm2 resulted in 88% protection. Furthermore, adjusting the number of UV treatments provided additional protection, reducing parasite burden by up to 94% (Urban and Tromba, Reference Urban and Tromba1984). These early studies using UV-attenuated eggs highlighted the fact that protective immunity against Ascaris could be induced. Following the use of UV-attenuated eggs, more specific Ascaris-derived products, such as larval stage ES products as well as purified fractions of the whole parasite, were evaluated (Urban, Reference Urban and Romanowski1985; Frontera et al., Reference Frontera, Carrón, Serrano, Roepstorff, Reina and Navarrete2003). Studies found that specific Ascaris worm products could provide up to 88% protection, suggesting more targeted antigens could play a role in inducing protective immunity.
In the early 1990s and 2000s, advances in molecular biology and genomics provided a new perspective for Ascaris vaccine development: instead of using live-attenuated or irradiated parasites or their products, recombinant proteins could be used. To identify potential antigenic targets, Ascaris proteins were screened in vitro and the most promising antigens were selected to move into pre-clinical trials based on their reactogenicity to immune serum from infected pigs. Several immunogenic recombinant proteins identified from A. suum, including As14 (Tsuji et al., Reference Tsuji, Suzuki, Kasuga-Aoki, Matsumoto, Arakawa, Ishiwata and Isobe2001), As16 (Tsuji et al., Reference Tsuji, Suzuki, Kasuga-Aoki, Isobe, Arakawa and Matsumoto2003; Wei et al., Reference Wei, Versteeg, Liu, Keegan, Gazzinelli-Guimarães, Fujiwara, Briggs, Jones, Strych, Beaumier, Bottazzi, Hotez and Zhan2017), As24 (Islam et al., Reference Islam, Miyoshi and Tsuji2005b), As37 (Tsuji et al., Reference Tsuji, Kasuga-Aoki, Isobe, Arakawa and Matsumoto2002; Versteeg et al., Reference Versteeg, Wei, Liu, Keegan, Fujiwara, Jones, Asojo, Strych, Bottazzi, Hotez and Zhan2020), enolase-1 (Chen et al., Reference Chen, Yuan, Xu, Zhou, Zhang, Zhang, Wang, Yan, Lin and Zhu2012) and AsPPase (Islam et al., Reference Islam2005a, Reference Islam, Miyoshi and Tsuji2005b) have been expressed in different recombinant protein systems, and used in pre-clinical trials. Vaccine efficacy of these recombinant proteins in mouse models has varied from 38 to 77%. Alone, recombinant protein-based vaccines are poorly immunogenic. Ascaris recombinant proteins can induce an antigen-specific antibody response polarized towards a type-2 immune phenotype (elevated IgG1 levels), which is thought to be advantageous in the protection against helminths, however this response is weak. Coupling recombinant proteins with immunogenic adjuvants provide a more robust immune profile. Use of adjuvants such as alum (alhydrogel™), which promotes a type-2 immune response, enhances the magnitude and durability of immunity. Coupling Ascaris recombinant proteins with bacterial agents such as cholera toxin B (CTB), that classically induces a mixed type-1 and type-2 immune response, was shown to promote vigorous mucosal antigen-specific IgA production, high levels of IgG1 and lower levels of IgG2a in addition to a 64% reduction in L3 recovery from vaccinated mice (Tsuji et al., Reference Tsuji, Suzuki, Kasuga-Aoki, Matsumoto, Arakawa, Ishiwata and Isobe2001). Adjuvants are now essential components of recombinant vaccine technology to generate a strong antigen-specific systemic immune response (Yamamoto et al., Reference Yamamoto, McGhee, Hagiwara, Otake and Kiyono2001; Chen et al., Reference Chen, Colditz, Glenn and Tsonis2002). Varying dosages, intervals between doses and different adjuvants have all been found to have significant impact on recombinant protein candidate performance.
Promising recombinant protein Ascaris vaccine targets: As14, As16 and As37
Tsuji et al. (Reference Tsuji, Suzuki, Kasuga-Aoki, Matsumoto, Arakawa, Ishiwata and Isobe2001) isolated cDNA encoding a 14-kDa antigen (As14) as well as a 16 kDa antigen (As16) from A. suum L3 larval stages. Both proteins are found in larvae and adult worms as well as in ES products and are under continued investigation as vaccine targets. To test the efficacy of As14 and As16, the proteins were expressed in an Escherichia coli system and coupled with CTB. The recombinant vaccine products were administered intranasally to mice and generated a 64% (As14) and 58% (As16) protection following challenge with A. suum in a mouse model (Tsuji et al., Reference Tsuji, Suzuki, Kasuga-Aoki, Matsumoto, Arakawa, Ishiwata and Isobe2001). Recombinant As16 coupled with CTB and administered to pigs was also partially protective against A. suum challenge (Tsuji et al., Reference Tsuji, Miyoshi, Islam, Isobe, Yoshihara, Arakawa, Matsumoto and Yokomizo2004). Furthermore, Wei et al. (Reference Wei, Versteeg, Liu, Keegan, Gazzinelli-Guimarães, Fujiwara, Briggs, Jones, Strych, Beaumier, Bottazzi, Hotez and Zhan2017) demonstrated that rAs16 formulated with the adjuvant Montanide ISA720 or alum induced protection against A. suum in mice and was associated with a type-2-skewed immune response and stunted larval development. However, when rAs16 was formulated with monophosphoryl lipid A (MPLA) or Addavax, known for a more predominant type-1 immune polarization, vaccinated mice were not protected against Ascaris challenge infection (Wei et al., Reference Wei, Versteeg, Liu, Keegan, Gazzinelli-Guimarães, Fujiwara, Briggs, Jones, Strych, Beaumier, Bottazzi, Hotez and Zhan2017).
The third candidate under investigation, 37 kDa protein (As37) isolated from A. suum infective eggs (Tsuji et al., Reference Tsuji, Kasuga-Aoki, Isobe, Arakawa and Matsumoto2002) is a member of the Ig superfamily and contains three Ig domains. Pre-clinical trials in mice found that recombinant As37 formulated with AddaVax™ adjuvant provided 48.7% worm reduction after challenge with A. suum infective eggs, and the protection was associated with a mixed Th1/2-type immune response characterized by high levels of IL-4, IL-5, IL-10 and IL-13 as well as IgG1 and IgG2a antibodies (Versteeg et al., Reference Versteeg, Wei, Liu, Keegan, Fujiwara, Jones, Asojo, Strych, Bottazzi, Hotez and Zhan2020). Additionally, sequence alignment of As37 with its homologues in other nematodes ranged from 80 to 100% similarity. Although anti-As37 strongly recognized hookworm and Trichuris muris homologues, it did not recognize human tissue, suggesting As37 may be a candidate for a pan-helminth vaccine (Versteeg et al., Reference Versteeg, Wei, Liu, Keegan, Fujiwara, Jones, Asojo, Strych, Bottazzi, Hotez and Zhan2020).
Finally, As14, As16 and As37 have recently been used together as a multivalent epitope-based vaccine in pre-clinical trials against Ascaris infection (de Castro et al., Reference de Castro, de Almeida, Cardoso, Oliveira, Nogueira, Reis-Cunha, Magalhaes, Zhan, Bottazzi, Hotez, Bueno, Bartholomeu and Fujiwara2021). The most immunogenic epitopes from the three selected proteins were predicted using bioinformatic tools. The peptides with the highest prediction scores were then selected for the construction of a chimeric protein. Notably, mice immunized with this novel chimeric protein alone exhibited only a 42.9% reduction in larval burden after Ascaris challenge, while mice immunized with the chimeric protein plus MPLA lead to a 74% larval reduction in addition to higher IgG responses and reduced lung inflammation. These data suggest that adjuvanted chimeric proteins containing more than one epitope offer a novel approach to the development of Ascaris vaccines, opening the door for creating intricate vaccine targets that can provide a more robust, targeted protective immunity.
Discussion and future directions
Although several vaccine candidates have been identified, developed and evaluated in pre-clinical animal trials, there is no vaccine candidate for Ascaris that has advanced into human clinical trials. Vaccine development requires significant time, financial investment as well as political and community interest. As ascariasis is the most prevalent-neglected tropical disease worldwide and associated with significant morbidity, it should be a major global interest to develop a vaccine to prevent and/or control infection. However, this has not been the case and researchers have struggled to fund and gain public interest in this endeavour. As a result, increasing awareness of ascariasis and other neglected tropical diseases (NTDs), including in HICs, has been a focus of public health campaigns. In the United States, public health campaigns have led to the proposition of new legislation termed ‘STOP: Study, Treat, Observe and Prevent Neglected Disease of Poverty’ Act in 2019 which aimed, in part, to accelerate the development of new control tools such as vaccines against NTDs (Hotez and Booker, Reference Hotez and Booker2020). Increasing political and social recognition of these diseases globally may lead to future funding and support to aid in vaccine development, particularly a pan-helminthic vaccine.
There remain significant barriers to the development of an Ascaris vaccine. Despite decades of progress in antigen discovery, results of pre-clinical testing of vaccine candidates have largely been underwhelming (as outlined in Table 1). Thus, further investigation in novel vaccine targets remains a critical step in the vaccine development process. Additionally, helminths are complex, multicellular organisms that have an intricate, helminth-specific and developmental-stage specific relationship with the host immune system (Weatherhead et al., Reference Weatherhead, Gazzinelli-Guimaraes, Knight, Fujiwara, Hotez, Bottazzi and Corry2020). Unfortunately, much of the helminth–host immune response interface remains unknown, which has limited discovery of new antigens and impeded the development of efficacious, directed and safe Ascaris vaccines. To date, most of the selected Ascaris antigens have been largely limited to worm or ES proteins, however undiscovered proteins may exist in other compartments such as in EVs. Further analysis of EVs, their role in communication with the host and development of immunity may provide insight into antigens of interest (Mekonnen et al., Reference Mekonnen, Pearson, Loukas and Sotillo2018). Another limitation in Ascaris vaccine development is the difficulty in selecting an appropriate target to induce protective immunity that does not simultaneously elicit an IgE response or an autoimmune response in the human host. This requires down-selecting of proteins with significant homology to common allergens and human proteins as well as down-selecting of proteins that induce strong IgE in natural infection. For example, pre-existing circulating hookworm-specific protein IgE from natural infection was found to result in urticarial reactions in individuals immunized with Na-ASP-2, a hookworm-specific protein previously tested in a human clinical trial (Diemert et al., Reference Diemert, Pinto, Freire, Jariwala, Santiago, Hamilton, Periago, Loukas, Tribolet, Mulvenna, Correa-Oliveira, Hotez and Bethony2012). Furthermore, although adjuvanted recombinant proteins have risen as lead targets for Ascaris vaccine development, the induced immune response, even with currently available adjuvants, may be insufficient for Ascaris control or eradication efforts (Noon and Aroian, Reference Noon and Aroian2017). Identifying innovative adjuvant platforms that can enhance vaccine efficacy must be a priority moving forward if recombinant proteins remain the main stay in Ascaris vaccine development programmes.
Modern vaccine technologies that lead to the discovery of novel immunogenic antigens may accelerate the development of a human Ascaris vaccine in the near future. Rapid advancement in the fields of transcriptomics and proteomics have provided an algorithmic pipeline to predict in silico the most immunogenic and antigenic B- and T-cell epitopes from Ascaris parasites based on their molecular and structural features (de Castro et al., Reference de Castro, de Almeida, Cardoso, Oliveira, Nogueira, Reis-Cunha, Magalhaes, Zhan, Bottazzi, Hotez, Bueno, Bartholomeu and Fujiwara2021). With this technology, it has become possible to screen the entire parasite without the need to maintain the parasite life cycle in vivo. This approach in Ascaris vaccine development, termed ‘reverse vaccinology’ (Rappuoli, Reference Rappuoli2000) was first utilized within the meningococcal B vaccine pipeline (Sette and Rappuoli, Reference Sette and Rappuoli2010). The reverse vaccinology principle is based on expressed transcriptome sequences or the entire protein repertoire to find new potential vaccine candidates, instead of using immunoassays to identify strongly immunogenic proteins against immune sera. This approach allows for streamlined exclusion of potential homologous immunogenic proteins to common allergens or human proteins. Protein microarray profiling also provides a high-throughput screening mechanism compared to traditional immune-screening techniques. Protein microarrays can evaluate multiple antigens to identify and quantify immunodominant antigens of vaccine interest (Peacock, Reference Peacock2014).
New vaccine platforms such as nucleic acid based vaccines (DNA and RNA) have recently gained substantial stride in vaccinology and could play an important role in Ascaris vaccine development. DNA vaccines have been investigated in other helminths such as schistosomiasis but have had minimal advancement to date in Ascaris vaccine development (Da'dara and Harn, Reference Da'dara and Harn2005). The use of mRNA technology has more recently emerged as a high-throughput technology with success in other pathogens of global importance. mRNA platforms allow for standardized production and purification of vaccine targets and can be multi-valent, induce strong, directed immune responses without adjuvant and are non-infectious. However, these technologies may be limited by high-cost and need for specialized production, manufacturing and storage which may not be conducive to parasite endemic regions particularly in LMICs (Versteeg et al., Reference Versteeg, Almutairi, Hotez and Pollet2019).
Moving forward, a vaccine remains the most likely tool to aid in global eradication of ascariasis. Advances in Ascaris genomics, transcriptomics and proteomics may provide the needed link to identify immunogenic products that are developmental-stage specific. This information would be essential to build vaccines that directly target the migrating larval stages, which would prevent both larvae-induced morbidity and the development of adult intestinal worms. These technologies may also provide the path forward for the development of a multi-valent pan-helminthic vaccine to support global STH elimination programmes. A pan-helminthic vaccine would be a major step in eradication of several parasites that share significant geographic overlap and help lead to reduction in over-all morbidity within parasite-endemic regions (Zhan et al., Reference Zhan, Beaumier, Briggs, Jones, Keegan, Bottazzi and Hotez2014). New technologies and investment in vaccine development on a global scale may overcome vaccine development roadblocks and bring us closer to an effective, safe and affordable Ascaris vaccine.
Acknowledgements
ACGG is a research fellow supported by CNPq (Brazilian National Council for Scientific and Technological Development). Tim Weatherhead edited the manuscript.
Author contributions
ACGG, PHGG and JW conceived and designed the study. ACGG, PHGG and JW wrote the article.
Financial support
This study was supported by NIH K08 AI143968-01 and by the Division of Intramural Research, NIH.
Conflict of interest
The authors declare that the research was conducted in the absence of any commercial or financial relationships that could be construed as a potential conflict of interest.