Introduction
Embryo culture media modifications play a crucial role in the improvement of assisted reproductive technology (ART) outcomes, as demonstrated following the addition of various factors into the media. Currently defined culture media used for human and bovine in vitro embryo production (IVP) do not contain all factors that are found in vivo, including endocrine hormones (Lane & Gardner, Reference Lane and Gardner2007). Recently, the supplementation of thyroid hormones (THs), which are present in serum and biological fluids at trace levels, has been shown to improve embryo production in cattle (Ashkar et al., Reference Ashkar, Semple, Schmidt, St John, Bartlewski and King2010a,Reference Ashkar, Bartlewski, Singh, Malhi, Yates, Singh and Kingb). We have reported previously that TH supplementation of in vitro culture (IVC) media significantly increased blastocyst formation rate, and the average cell numbers of blastocysts, while supplementation significantly decreased overall numbers of apoptotic cells in the embryos (Ashkar et al., Reference Ashkar, Semple, Schmidt, St John, Bartlewski and King2010a). Moreover, TH supplementation enhanced post-cryopreservation viability of embryos.
Sufficient evidence exists to indicate that the early bovine embryo possesses sufficient maternal molecular machinery to genomically respond to THs. We previously identified THs at the in vivo site of early embryo development in bovine oviduct and uterine horn. Expression of thyroid hormone receptors (TR) in both oocytes and granulosa cells has been reported in multiple species, including cattle (Zhang et al., Reference Zhang, Carrillo and Darling1997; Ashkar et al., Reference Ashkar, Semple, Schmidt, St John, Bartlewski and King2010a, Reference Ashkar, Bartlewski, Singh, Malhi, Yates, Singh and Kingb). Although THs influence gene expression and the associated mRNA levels may not directly represent molecular functionalities, these have been used previously and are considered markers of embryo competency (Cagnone et al., Reference Cagnone, Dufort, Vigneault and Sirard2012).
Thyroxine (T4) and triiodothyronine (T3) are produced and released from the thyroid gland to mediate several biological processes that are important for developing embryos including growth regulation, metabolism and cell differentiation (Oetting & Yen, Reference Oetting and Yen2007; Harper & Seifert, Reference Harper and Seifert2008). THs have been shown previously to be present at the site of oocyte maturation and fertilization (Ashkar et al., Reference Ashkar, Bartlewski, Singh, Malhi, Yates, Singh and King2010b) and with improved in vitro embryo development in cattle (Ashkar et al., Reference Ashkar, Semple, Schmidt, St John, Bartlewski and King2010a). Furthermore, it has been proven that they influence early embryonic development in amphibians, and fetal development in mammals (Power et al., Reference Power, Llewellyn, Faustino, Nowell, Bjornsson, Einarsdottir, Canario and Sweeney2001; Morvan-Dubois et al., Reference Morvan-Dubois, Fini and Demeneix2013). However, our knowledge of the effect of THs on the transcriptome in mammals prior to implantation is limited. TH-mediated effects mainly occur through genomic mechanisms at various subcellular targets and compartments including: the nucleus, plasma membrane, cytoplasm and cytoplasmic organelles (Cheng et al., Reference Cheng, Leonard and Davis2010; Wu & Koenig, Reference Wu and Koenig2000; Zhang & Lazar, Reference Zhang and Lazar2000). In vertebrates, regulation of gene expression is generally mediated by intra-nuclear isoforms of TRs: alpha and beta (Lazar, Reference Lazar1993). In fact, T3 may itself act as a transcription factor (Yen et al., Reference Yen, Ando, Feng, Liu, Maruvada and Xia2006).
Modulation of cell metabolism may underlie or contribute to in vitro embryo survival and competency. Thyroid hormones are the major regulators of cell metabolism and it is well known that mitochondria are the primary site of TH action (Harper & Seifert, Reference Harper and Seifert2008). Embryo ATP content and mitochondrial numbers have been correlated with embryo competency and development in human, mouse and cattle species (Nagai et al., Reference Nagai, Mabuchi, Hirata, Shoda, Kasai, Yokota, Shitara, Yonekawa and Hoshi2006; Van Blerkom, Reference Van Blerkom2009, Reference Van Blerkom2011; Jeseta et al., Reference Jeseta, Ctvrtlikova Knitlova, Hanzalova, Hulinska, Hanulakova, Milakovic, Nemcova, Kanka and Machatkova2014;). The addition of dichloroacetic acid (DCA) to IVC media has been shown to stimulate mitochondrial activity, and has resulted in increased blastocyst rate, and in higher quality embryos that contained more ATP (Mtango et al., Reference Mtango, Harvey, Latham and Brenner2008; McPherson et al., Reference McPherson, Zander-Fox and Lane2014). Mtango et al. reported on reduced mitochondrial related transcript levels in rhesus monkey embryos derived from standard, non-supplemented in vitro media (Mtango et al., Reference Mtango, Harvey, Latham and Brenner2008). Therefore, THs might contribute to the regulation of mitochondrial function in the in vitro cultured embryo.
In cattle, sex-associated gene expression in the preimplantation embryo has been reported previously (Peippo et al., Reference Peippo, Farazmand, Kurkilahti, Markkula, Basrur and King2002; Bermejo-Alvarez et al., Reference Bermejo-Alvarez, Rizos, Rath, Lonergan and Gutierrez-Adan2010). Furthermore, shifts in the sex ratio among IVP bovine embryos that reach the blastocyst stage have also been demonstrated (Avery et al., Reference Avery, Madison and Greve1991; Xu et al., Reference Xu, Yadav, King and Betteridge1992; Bousquet et al., Reference Bousquet, Twagiramungu, Morin, Brisson, Carboneau and Durocher1999; Kochhar et al., Reference Kochhar, Peippo and King2001). This effect was maintained at birth after embryo transfer (Camargo et al., Reference Camargo, Freitas, de Sa, de Moraes Ferreira, Serapiao and Viana2010). As this phenomenon has been reported across multiple animal species (Milki et al., Reference Milki, Jun, Hinckley, Westphal, Giudice and Behr2003), in vitro-produced male embryos are thought to grow faster and exhibit greater viability than females (Avery et al., Reference Avery, Madison and Greve1991; Kochhar et al., Reference Kochhar, Peippo and King2001). However, the mechanisms or triggers associated specifically with this aspect of in vitro development are not known.
The main objective of this study was to characterize the effect of THs on the bovine embryonic transcriptome to better understand early embryo development in the presence of THs. A bovine-specific microarray (Robert et al., Reference Robert, Nieminen, Dufort, Gagne, Grant, Cagnone, Plourde, Nivet, Fournier and Paquet2011) was used to conduct a large-scale analysis of gene expression with a focus on those genes associated with biological functions related to mitochondrial function, embryo quality, developmental rates and survival, as well as embryo sex ratio. Functional analysis software was used to categorize those gene clusters that were associated with TH-related processes. The blastocyst stage was chosen as an endpoint for this study as it is an ideal time point for embryo transcription analysis, as the maternal transcriptome is at its minimum and embryonic genome is being transcribed during this period (Gad et al., Reference Gad, Besenfelder, Havlicek, Holker, Cinar, Rings, Dufort, Sirard, Schellander and Tesfaye2012). It should be noted that in mammals, maternal transcriptome and ooplasm content are the main sources of mRNA for zygote development during the zygote to 4-cell stage (Sylvestre et al., Reference Sylvestre, Robert, Pennetier, Labrecque, Gilbert, Dufort, Léveillé and Sirard2013). Therefore, in order for the zygote to reach the blastocyst stage, it must begin generating transcripts from its own genome (Goossens et al., Reference Goossens, Van Soom, Van Poucke, Vandaele, Vandesompele, Van Zeveren and Peelman2007). In cattle, transcription has been shown as early as the late zygote or 2-cell stage (Plante et al., Reference Plante, Plante, Shepherd and King1994; Memili & First Reference Memili and First2000), which was followed by a burst of transcription at the 8- to 16- cell stages (Goossens et al., Reference Goossens, De Spiegelaere, Stevens, Burvenich, De Spiegeleer, Cornillie, Van Zeveren, Van Soom and Peelman2012); in this case THs treatment significantly increased the number of blastocysts that could be obtained in IVP systems (Ashkar et al., Reference Ashkar, Semple, Schmidt, St John, Bartlewski and King2010a).
Materials and methods
Experimental design
Bovine oocytes were retrieved post-mortem from ovaries obtained from the Canadian Food Inspection Agency and the University of Guelph Animal Care Committee approved local slaughterhouse (Cargill Meat Solutions, Guelph, ON, Canada), and were subjected to a standard IVF protocol. Presumptive zygotes were divided equally into two groups: the control group cultured in standard IVC synthetic oviduct fluid (SOF) system; and the treated group cultured in IVC supplemented with free forms of THs (T3-triiodothyronine and T4-thyroxine). This experiment was conducted in four different trials and approximately 1100 oocytes were used. Blastocysts were harvested on day 8 post insemination.
Chemicals
All chemicals were purchased from Sigma-Aldrich Chemical Company (Oakville, ON, Canada) unless stated otherwise.
In vitro embryo production
Oocytes were aspirated and subjected to in vitro maturation (IVM), in vitro fertilization (IVF) and in vitro culture (IVC) as previously described by Mastromonaco et al. (Reference Mastromonaco, Favetta, Smith, Filion and King2007) and Ashkar et al. (Reference Ashkar, Semple, Schmidt, St John, Bartlewski and King2010a). In brief, cumulus–oocyte complexes (COCs) were collected from visible ovarian follicles greater than 2 mm in diameter by aspiration into HEPES-buffered Ham's F-10 plus 2% steer serum (Cansera; Rexdale, ON, Canada). Oocytes with several layers of cumulus cells and homogenous cytoplasm were then randomly assigned into control and treated groups. COCs were matured in 80 μl drops (20 oocytes per drop) of TCM199 medium + 2% steer serum (IVM) supplemented with 1 μg/ml of estradiol, 0.5 μg/ml of bFSH and 1 μg/ml of bLH (NIH, Washington, DC, USA) (IVM + H) under silicone oil (Paisley Products, Scarborough, ON, Canada) for 20–22 h at 38.5°C in 5% CO2 in air. Co-incubation with sperm was carried out in 80 μl drops of IVF TALP under silicone oil (Paisley Products, Scarborough, ON, Canada) for 18 h at 38.5°C in 5% CO2 in air. Frozen–thawed sperm was prepared by swim-up in 5 ml of sperm TALP and centrifugation at 2000 g for 10 min. The oocytes were inseminated with a final concentration of 1 × 106 motile frozen–thawed sperm/ml (Eastgene, Guelph, ON, Canada).
In vitro culture
Presumptive embryos from microdroplets were collected and transferred into a 15 ml conical tube containing 2 ml sperm TALP. Embryos were then vortexed for approximately 60 s to remove the cumulus cells, after which they were washed twice in sperm–HEPES TALP and twice in SOF. Half of the prospective embryos were put in the treatment group which received SOF supplemented with 50 ng/ml of each T3 and T4 (Ashkar et al., Reference Ashkar, Semple, Schmidt, St John, Bartlewski and King2010a; Costa et al., Reference Costa, Cordeiro, Silva, Sastre, Santana, Sa, Sampaio, Santos, Adona and Miranda2013). The embryos were transferred to their respective 30μl SOF droplets, with or without TH supplement. Each droplet contained approximately 30 embryos, and was incubated at 38.5°C, 5% CO2 and 5% O2. Each replicate of the experiments began with matched pools of oocytes for controls and treated embryos, which were followed until the blastocyst stage.
Embryo developmental rates and sex ratio
Blastocyst formation rates on day 8 were calculated as a percentage of presumptive zygotes using ANOVA and GLM tests. Whole pools of blastocysts rather that selected blastocysts from larger pools were processed for sex determination. Sex ratio in 96 individual blastocysts from treated and 50 from control groups in four different IVF runs in similar morphological state was determined by the amplification of the Y-chromosome linked testis specific transcript Y-encoded (TSPY) gene and the autosomal control GAPDH gene (Hamilton et al., Reference Hamilton, Combe, Caudle, Ashkar, Macaulay, Blondin and King2012; Macaulay et al., Reference Macaulay, Hamilton, King and Bartlewski2013). A Z-test was used to calculate a Z-value for each group, which resulted in 90% of reliability in the ratio differences.
Gene array analysis
Groups of five day 8 expanded blastocysts with similar morphology were harvested from four biological replicates and, to unify the gene array protocol and permit future meta-analysis, the standard operating procedure (SOP) provided by the NSERC funded EmbryoGene group, located at Laval University (http://embryogene.ca/), was followed.
RNA extraction, amplification labelling and hybridization
Total RNA from each replicate was extracted and purified using the PicoPure RNA Isolation Kit (Life Science) as described by Robert et al. (Reference Robert, Nieminen, Dufort, Gagne, Grant, Cagnone, Plourde, Nivet, Fournier and Paquet2011). After DNase digestion (Qiagen), RNA concentration and quality were assessed using a Bioanalyzer 2100 (Agilent) and the remaining sample was stored at –80°C.
To perform microarray hybridization, purified RNA was amplified by two rounds of in vitro T7 transcription using the RiboAmp HSPlus RNA Amplification Kit (Life Science) and labelled with Cy3 and Cy5 using the ULS Fluorescent Labeling Kit (Kreatech, Durham, NC, USA). The resulting antisense RNA (825 ng per replicate) was hybridized on the Agilent-manufactured EmbryoGene design slides (Cagnone et al., Reference Cagnone, Dufort, Vigneault and Sirard2012). The hybridizations were done using a two-colour design with full dye-swap. Hybridization took place for 17 h at 65°C after which slides were washed for 1 min in gene Expression Wash Buffer 1 at room temperature and 3 min in gene Expression Wash Buffer 2 (42°C) following 10 s in 100% acetonitrile (room temperature), and finally 30 s in Stabilization and Drying Solution (Agilent). Slides were scanned with a Power Scanner (Tecan) and features extraction was done with Array-pro6. (Media Cybernetics).
Microarray data analysis
Microarray data analysis was performed using FlexArray version 1.6 software (Blazejczyk et al., Reference Blazejczyk, Miron and Nadon2007; http://genomequebec.mcgill.ca/FlexArray). Data were submitted to a Normexp background subtraction, Loess within-array normalization, and finally statistically analyzed using the Limma R package (R version 2.12.1 and Limma version 3). Genes were considered differentially expressed between TH-treated and untreated groups at a fold-change greater than 1.5 with a P-value <0.05.
The resulting list of annotated genes was further analyzed for enrichment in Gene Ontology (GO) terms using the various tools implemented in the WEB-based Gene SeT AnaLysis Toolkit (WebGestalt; Zhang et al., Reference Zhang, Kirov and Snoddy2005; http://bioinfo.vanderbilt.edu/webgestalt). The array-specific gene list was used as a reference set for the hypergeometric statistical test with default adjustment parameters. The Ingenuity Pathway Analysis (IPA) database and ProfCom software (Antonov et al., Reference Antonov, Schmidt, Wang and Mewes2008, http://webclu.bio.wzw.tum.de/profcom/index.php) were also used to identify groups of genes with common biological functions, enriched in the list of differentially expressed genes. The microarray data have been deposited in the NCBI Gene Expression Omnibus with accession number GSE58901.
Validation of microarray results by RT-qPCR
Quantitative real-time polymerase chain reaction (PCR) was used to validate the microarray results. Two parallel reverse transcription reactions were set up from each amplified RNA sample using random hexamers (250 ng) and Superscript II enzyme (Invitrogen). The thermal profile consisted of denaturation (at 72°C for 2 min) and incubation (at 25°C for 10 min) of the sample–primer mix before heating to 42°C and addition of other reaction components (1× RT buffer, 0.01M DTT, 1 mM dNTP, 20 U RNasin (Promega), 200U Superscript II). The 1 h incubation was followed by 30 min at 70°C. Next, 10 ng cDNA was used in a 10 μl qPCR reaction containing 1× SsoFast EvaGreen Supermix (Bio-Rad) and 0.25 μM primers run in a CFX96 Touch Real-time PCR system (Bio-Rad) with the following cycle conditions 95°C, 3 min; 50× (95°C, 10 s; 60°C, 10 s; 72°C, 10 s). Melting curve was registered using 95°C for 10 s ramping from 70°C to 95°C by 0.5°C/step at the end of the reaction. Amplicon-specific thresholds were selected to determine Cq values and to calculate relative expression ratios using the CFX Manager software (Bio-Rad). The gene-specific PCR efficiency was calculated by averaging the individual amplification curve-based values determined by the LinRegPCR software (Ramakers et al., Reference Ramakers, Ruijter, Deprez and Moorman2003). Specific primers were designed using the Primer3 plug-in of Geneious 3.8.5 (www.geneious.com) for each gene selected for validation (ABCC3, ATP5H, COX6C, HDHD1A, NDUFS1) and a set of potential reference genes (18S, GAPDH, H2a, HPRT, YWHAZ). The latter was tested for stability across the treated and control groups using the GeNorm tool (Biogazelle) to select appropriate normalizer reference genes. Primer sequences, product sizes and accession numbers are listed in Table 1. The Pearson correlation coefficient of the array expression ratios and qPCR relative expressions were calculated in Microsoft Excel.
Table 1 List of genes and primer sequences used for qPCR validation
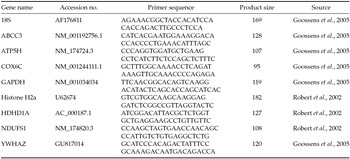
Results
Embryo development rates and sex ratio in the embryos treated with THs vs. control
Here we confirmed that THs significantly increased blastocyst formation rate when IVC was supplemented with THs (P < 0.05) (Fig. 1) and that the embryos used in this study exhibited the developmental profile of TH-treated embryos described previously (Ashkar et al., Reference Ashkar, Semple, Schmidt, St John, Bartlewski and King2010a). Moreover, sex ratio in the treated blastocysts shifted towards a greater number of females than did the control embryos. The difference in sex ratio between the treated and control groups was statistically significant with 95% reliability (Table 2).
Table 2 Blastocysts sex ratios

a Significant increase (P < 0.05) in the percentage of female blastocysts in the TH-treated group.
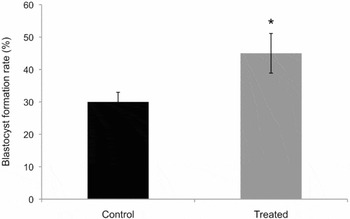
Figure 1 Blastocyst rates in control vs. THs treated. The average per cent of blastocyst formation rate (y-axis) on day 8 post insemination was significantly higher in the TH-treated group vs. control (*P < 0.05).
Global gene expression in TH-treated and control blastocysts
Among the 37,238 array gene-specific probes, 29,028 and 27,867 (control and treated groups respectively) showed signal intensities that were higher than the average intensity of background control spots plus twice the standard deviation. Statistical analysis revealed that, after background correction and subtraction, 1212 genes were differentially expressed in TH-treated embryo cells, 462 genes were down-regulated and 750 were up-regulated. In addition to the 1212 differentially expressed genes in the treated group, 23 were only expressed in controls and eight were only expressed in treated embryos (Tables 3 and 4). The microarray results were validated by RT-qPCR. First, a panel of potential reference genes was tested for stability across the experimental IVP conditions. The GeNorm analysis identified the YWHAZ, GAPDH and HPRT1 genes as most stable suitable reference genes. The geometric mean of their expression was used for normalization of target genes. Relative expression of five selected genes (ABCC3, ATP5H, COX6C, HDHD1A, NDUFS1) showed high correlation (0.926) with the microarray results (Fig. 2).
Table 3 Genes expressed only in TH-treated embryos

Table 4 List of genes expressed in control embryos
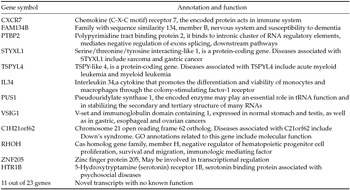
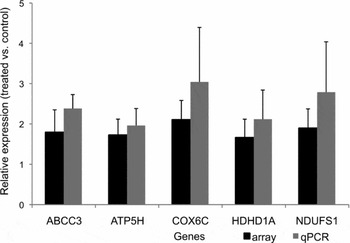
Figure 2 Array validation with differentially expressed genes in treated embryos. The black bars represent the relative expression level in the array and the grey bars represent the average relative expression of those genes in qPCR. The y-axis represents the relative fold expression of the selected genes and the axis are the genes selected.
Differential expression of major functional gene clusters
The resulting list of 1212 differentially expressed genes and 31 uniquely expressed genes were subjected to GO enrichment analysis: a database that categorizes genes according to their common functions in a certain cellular compartment or biological process (http://www.geneontology.org). The major functional clusters among differentially expressed genes that were identified by IPA (Fig. 3) were: Cellular Growth and Proliferation (146 genes), Cellular Development (67 genes), Embryonic Development (37 genes), DNA Replication and Repair (34 genes), and Mitochondria (14 genes). While genes associated with THs encoding TRs and deiodinases (DIO I, II and III) were not differentially expressed, their transcript abundance was very close to the detection limit in both groups. The WebGestalt software found 49 highly significant enrichment of genes localized in the mitochondrion (P < 0.005). IPA also revealed an enrichment of 13 genes associated with mitochondrial activity of ATP synthesis coupled electron transport (P < 0.0005).

Figure 3 Functional analysis of differentially expressed genes in treated vs. control embryos using Ingenuity Pathway Analysis database. The grey bars in the chart represent those differentially expressed genes were clustered according to their function in embryogenesis.
Differential expression of sex chromosome genes
Of the 1629 X-chromosome linked gene probes in the array, 52 were differentially expressed in treated blastocysts only (Table 5). Although Y-chromosomal transcripts have not been fully annotated on the microarray, three transcripts (EIF1AY, TSPY, DDX3Y) were identified among those expressed genes, thus confirming the presence of male embryos in each sample pool.
Table 5 X-chromosome linked genes upregulated in the treated embryos

Discussion
The goal of this study was to characterize the effect of THs on the bovine embryonic transcriptome in blastocyst stage embryos that were derived from IVC media supplemented with THs. This study, which looked at transcriptional consequences of THs in the early embryo, is the first of its kind in mammals. We observed that TH supplementation led to differential expression of about 4% of the transcriptome covered by the array and induced a slight but significant shift in sex ratio toward female (15% increase) compared with untreated controls.
Gene array analysis showed that 1212 genes were differentially expressed in TH-treated embryos; the differentially expressed genes were predominantly associated with growth, proliferation, embryo development, DNA repair and duplication, as well as metabolism and mitochondrial function. Conversely, genes associated with TRs, and DIOs were expressed at similar levels in both TH-treated and untreated groups. The change in total transcriptome following TH supplementation has been demonstrated previously in early embryos of amphibians and fish (Pelayo et al., Reference Pelayo, Oliveira, Thienpont, Babin, Raldua, Andre and Pina2012). Addition of 50 nM T3 to the culture medium on days 2–5 post fertilization resulted in a 2% change in zebrafish transcriptome including genes involved in organogenesis and TR genes. Our study further supports the significant increase in gene expression resulting from TH supplementation.
Several studies have shown sex-related differences in gene expression and transcript levels. For example, De La Fuente et al. (Reference De La Fuente, Hahnel, Basrur and King1999) showed that the X inactive-specific transcript (XIST) expression is unique to female blastocysts, while Hamilton et al. (Reference Hamilton, Combe, Caudle, Ashkar, Macaulay, Blondin and King2012) showed that DDX3Y and EIF1AY expression was unique to male blastocysts. At the transcriptome level, Bermejo-Alvarez et al. (Reference Bermejo-Alvarez, Rizos, Rath, Lonergan and Gutierrez-Adan2010) reported that when male and female blastocysts were compared about one-third of transcripts showed differential expression that was sexually dimorphic. In our study, it might be argued that the differential expression of transcripts was due to the fact that there were potentially 15% more female embryos in the pools of treated embryos. However, as the cut-off for considering genes to be differentially expressed was 1.5-fold and we used multiple pools of mixed sex blastocysts it seems unlikely that a small increase in the number of females would have such an effect. Furthermore, genes known to be expressed uniquely in male (DDX3Y, EIF1AY) or in female (XIST) blastocysts were not differentially expressed in the treated embryo pools compared with control embryo pools. A more likely scenario is that individual treated embryos of both sexes exhibited differentially expressed transcriptomes compared with untreated controls.
In all, 23 genes were exclusively expressed in controls, and eight in TH-treated blastocysts. Those genes, expressed only in control blastocysts, were primarily associated with immunological reaction processes (IL-34) (Masteller & Wong, Reference Masteller and Wong2014; Chang et al., Reference Chang, Lee, Song, Jang, Park, Hong, Ko, Kim, Kim, Lee and Heo2014), pathologies including cancer (STYXL1 and TSPYL4), such as lung carcinoma (You et al., Reference You, Wang, Huang, Jiang, Chen, Wang and Jiang2015) and with negative regulatory effectors and leukemia (RHOH) (Sanchez-Aguilera et al., Reference Sanchez-Aguilera, Rattmann, Drew, Müller, Summey, Lucas, Byrd, Croce, Gu, Cancelas, Johnston, Moritz and Williams2010; Troeger et al., Reference Troeger, Johnson, Wood, Blum, Andritsos, Byrd and Williams2012, Reference Troeger and Williams2013; Troeger & Williams, Reference Troeger and Williams2013). Conversely, genes expressed exclusively in TH-treated blastocysts have been associated with cardiac cell differentiation (IRX5; Gaborit et al., Reference Gaborit, Sakuma, Wylie, Kim, Zhang, Hui and Bruneau2012) spinal cord functional plasticity and regulation of immature heart function and hypertrophy (NCS1, Yip et al., Reference Yip, Wong, Sears, Yáñez-Muñoz and McMahon2010; Nakamura et al., Reference Nakamura, Jeromin, Mikoshiba and Wakabayashi2011) and cell migration and turnover (SPATA13, Evans et al., Reference Evans, Hines, Forsythe, Erdogan, Shi, Hill, Rose, McLean and Webb2014) and those generally associated with either stimulation of protein synthesis (LOC515517) or as transcription mediators (STAT4). In particular, LOC515517, also known as Larp1, has been shown to be required for spermatogenesis, embryogenesis, mitosis and cell cycle progression (Burrows et al., Reference Burrows, Abd Latip, Lam, Carpenter, Sawicka, Tzolovsky, Gabra, Bushell, Glover, Willis and Blagden2010) while IRX5 appears to be involved in embryonic/fetal mouse cardiac development has been indicated for IRX5 (Gaborit et al., Reference Gaborit, Sakuma, Wylie, Kim, Zhang, Hui and Bruneau2012). STAT4 is the only one among these eight genes to have a direct connection with thyroid hormone, as it has been associated with thyroid autoimmune disease (Ben Hamad et al., Reference Ben Hamad, Cornelis, Mbarek, Chabchoub, Marzouk, Bahloul, Rebai, Fakhfakh, Ayadi, Petit-Teixeira and Maalej2011; Yan et al., Reference Yan, Meng, Zhou, Xu, Muhali, Jiang, Shi, Shi and Zhang2014). Overall, those genes exclusively expressed in treated blastocysts were associated with regulatory processes, which was expected given the known biological role of THs. However, the expression of genes in control embryos may have contributed to suboptimal development in those embryos.
Important to note is that the genes chosen for array validation were among those known to be associated with mitochondrial function. These genes exhibited the greatest stability and strongest correlation (92%) with the array. Moreover, four of those mitochondrial genes are known to function in oxidative phosphorylation ATP5H, COX6C, NDUFC2 and NDUFS1. The cytochrome-C oxidase subunit (COX6c) has been reported to be upregulated with THs in tissue culture (Sheehan et al., Reference Sheehan, Kumar and Hood2004). The same trends for mitochondrial regulatory genes are expected by THs genomic action (Cheng et al., Reference Cheng, Leonard and Davis2010).
Bioinformatics analysis showed differential expression of 49 mitochondrial genes following TH administration; 13 of these were associated with electron transfer chain elements and 14 with ATP production. Increased expression of these mitochondrial genes may underlie more efficient energy expenditure in embryonic cells, as evidenced by our previous reports of enhanced tolerance to cryopreservation in TH-treated embryos (Ashkar et al., Reference Ashkar, Semple, Schmidt, St John, Bartlewski and King2010a). The role of mitochondria in mammalian early embryo competency has been well established (Tarazona et al., Reference Tarazona, Rodriguez, Restrepo and Olivera-Angel2006; Van Blerkom, Reference Van Blerkom2011). The positive correlation between oocyte ATP content and successful preimplantation bovine embryo development has been reported. As such, oocyte ATP content may be considered a biomarker of embryo quality and pregnancy outcome (Stojkovic et al., Reference Stojkovic, Machado, Stojkovic, Zakhartchenko, Hutzler, Goncalves and Wolf2001; Nagano et al., Reference Nagano, Katagiri and Takahashi2006). Enhanced embryo development and survival rates, as well as elevated expression of mitochondrial regulator genes, are all consistent with anticipated TH influenced mitochondrial functions.
Pathway analysis also identified differential expression of 34 genes in TH-treated embryos that were associated specifically with DNA replication and repair. Embryo survival requires the maintenance of cellular DNA integrity (Menezo et al., Reference Menezo, Dale and Cohen2010) and about 15–20% of IVF-produced embryos arrest in the first few cleavage stages; this finding has in part been attributed to DNA damage (Favetta et al., Reference Favetta, Robert, St John, Betts and King2004; King et al., Reference King, Coppola, Alexander, Mastromonaco, Perrault, Nino-Soto, Pinton, Joudrey and Betts2006; Betts & Madan Reference Betts and Madan2008; King, Reference King2008). Imbalanced regulation of DNA repair and replication may ultimately induce excessive cell loss through DNA damage and apoptosis (Yoshida & Miki, Reference Yoshida and Miki2004). Previous studies have shown that THs influence DNA repair in amphibian and fish embryos (Atkinson et al., Reference Atkinson, Warkman and Chen1998; Gavriouchkina et al., Reference Gavriouchkina, Fischer, Ivacevic, Stolte, Benes and Dekens2010). It is known that early embryos express a series of genes responsible for DNA repair activities during the oocyte to blastocyst stages (Jaroudi & SenGupta, Reference Jaroudi and SenGupta2007; Jaroudi et al., Reference Jaroudi, Kakourou, Cawood, Doshi, Ranieri, Serhal, Harper and SenGupta2009). As such, enhanced embryo survival may also be supported by TH-induced elevations in DNA repair genes.
Unlike mitochondrial and DNA repair associated genes, TR and DIO gene expression levels were not significantly different between treated and untreated embryos, suggesting that TR expression may be either independent of TH presence/supplementation, or not inducible. This finding suggested the importance of TR expression in the earlier stages of embryogenesis, in which embryos do not possess endogenous THs. TR expression has previously been reported in granulosa cells of oocytes of cattle (Costa et al., Reference Costa, Cordeiro, Silva, Sastre, Santana, Sa, Sampaio, Santos, Adona and Miranda2013) and other mammalian species (Zhang et al., Reference Zhang, Carrillo and Darling1997). At the transcript (mRNA) level, these findings suggest that sufficient TR and deiodinases already exist at the embryo stage to metabolize THs and regulate bioavailability of each analogue if needed.
Another unique finding of the study was that GO analysis of sex chromosomes identified differential expression of 52 X-chromosome linked genes in the treated blastocysts, 46 of which were novel genes with unidentified functions, and six genes (ASMTL, HDHD1A, KAL1, MSL3, PIN4, TKTL1) have known functions involving chromatin remodeling, cell cycle regulation and energy metabolism. The over-expression of these particular genes may have been the result of either two actively transcribed X chromosomes, or developmentally associated X-linked genes that escaped gene inactivation. Indeed, five of these genes are known to be located in the region of the human X-chromosome that is known to escape inactivation (Yen et al., Reference Yen, Ellison, Salido, Mohandas and Shapiro1992).
The enhanced expression of X-linked genes may have also increased survival of female embryos that might have otherwise been destined to arrest prior to blastocyst formation. As such, the skewed sex ratio within treated embryos to favour females may have been the result of greater female embryo survival. Whether TH influenced sex ratio through modulation of energy regulation or X-chromosome linked gene activation, requires further investigation.
In summary, this study is the first to demonstrate that TH treatment induces differential gene expression in mammalian embryos at the blastocyst stage. Wide ranging increases in differentially expressed genes is predictable based on the known physiological effect of THs. Sex ratio inversion and over-expression of X-linked genes were interesting and unanticipated findings that merit further study.
Funding
The work was supported by Discovery Grants from the National Science and Engineering Research (NSERC) Council of Canada, the Canada Research Chair's program, Agriculture and Agrifood Canada, Ontario Gradate Scholarship and EmbryoGene, Canada.
Acknowledgements
This work was supported by a grant from the Natural Sciences and Engineering Research Council of Canada (grant number: NETGP-340825–06) for the Strategic Research network EmbryoGENE (www.embryogene.ca)