Introduction
Panic disorder (PD) is a particularly severe and costly anxiety disorder, affecting approximately 7.8 million people in Europe (Wittchen et al. Reference Wittchen, Jacobi, Rehm, Gustavsson, Svensson, Jonsson, Olesen, Allgulander, Alonso, Faravelli, Fratiglioni, Jennum, Lieb, Maercker, van Os, Preisig, Salvador-Carulla, Simon and Steinhausen2011). Patients experience recurrent and sudden attacks of intense anxiety and concerns about their potential implications, often followed by agoraphobia (AG), the avoidance of situations in which escape or help may not be available (APA, 2000). Ranking among the 10 most burdensome psychiatric and neurological diseases in the reproductive years, PD is one of most disabling anxiety disorders (Wittchen et al. Reference Wittchen, Jacobi, Rehm, Gustavsson, Svensson, Jonsson, Olesen, Allgulander, Alonso, Faravelli, Fratiglioni, Jennum, Lieb, Maercker, van Os, Preisig, Salvador-Carulla, Simon and Steinhausen2011).
Fear conditioning has been proposed as a core process for the development and maintenance of PD/AG (Bouton et al. Reference Bouton, Mineka and Barlow2001). Interoceptive conditioning, where the accompanying physiological symptoms during a panic attack become conditioned stimuli, is linked to interoceptive symptoms in PD whereas exteroceptive conditioning is associated with agoraphobic behaviour (Bouton et al. Reference Bouton, Mineka and Barlow2001). However, the particular nature of the underlying conditioning deficit in PD/AG remains elusive.
During fear conditioning, a neutral stimulus is paired repeatedly with an aversive unconditioned stimulus (US). The neutral stimulus gradually becomes a conditioned stimulus (CS), which elicits a conditioned fear response signalling the anticipation of the US. Different learning processes are indexed by differential conditioning, simple conditioning and safety signal processing. Differential conditioning includes a second CS that is not paired with the US and acquires safety signal properties (CS−). Conditioned responses are indexed by the difference between the two CSs, thus representing the relative difference of the two processes involved (e.g. discriminatory conditioning): fear conditioning towards the CS+ and fear inhibition towards the CS−. By contrast, simple conditioning evaluates conditioning effects of the CS against a preconditioning baseline, assessing mere association of a CS with the US independent of discriminatory capabilities. During safety signal processing, the capacity to inhibit a fear response in the presence of a safety signal (CS−) is assessed. Failure to inhibit the conditioned response could result in pathological overgeneralization of fear (Lissek, Reference Lissek2012). Using exteroceptive conditioning tasks, enhanced simple conditioning (Lissek et al. Reference Lissek, Powers, McClure, Phelps, Woldehawariat, Grillon and Pine2005), deficient safety signal processing (Lissek et al. Reference Lissek, Rabin, McDowell, Dvir, Bradford, Geraci, Pine and Grillon2009) or increased resistance to extinction learning, evidencing more persistent recall of the conditioned response (Michael et al. Reference Michael, Blechert, Vriends, Margraf and Wilhelm2007) has been suggested to account for learning deficits in PD.
The neural network of fear conditioning has been extensively studied in humans using functional magnetic resonance imaging (fMRI; for a review see Sehlmeyer et al. Reference Sehlmeyer, Schoning, Zwitserlood, Pfleiderer, Kircher, Arolt and Konrad2009). Extending animal research focusing on the amygdala as a key region (LeDoux et al. Reference LeDoux, Iwata, Cicchetti and Reis1988), fMRI studies have revealed a cortical and subcortical network encompassing the thalamus, amygdala, hippocampus, insula, anterior cingulate cortex (ACC) and prefrontal/orbitofrontal cortex (PFC/OFC) involved in human fear conditioning (Sehlmeyer et al. Reference Sehlmeyer, Schoning, Zwitserlood, Pfleiderer, Kircher, Arolt and Konrad2009). This network has substantial overlap with fear circuitry structures that show abnormal activation in different anxiety disorders (Etkin & Wager, Reference Etkin and Wager2007; Shin & Liberzon, Reference Shin and Liberzon2010), further supporting the suitability of fear conditioning as a behavioural probe to investigate the neural substrates of anxiety disorders (Gorman et al. Reference Gorman, Kent, Sullivan and Coplan2000). Although fear circuitry dysfunctions in a network that includes the amygdala, hippocampus, thalamus, hypothalamus, periaqueductal grey (PAG) and locus coeruleus (Gorman et al. Reference Gorman, Kent, Sullivan and Coplan2000) have been proposed in the pathogenesis of PD, models so far are mainly based on animal work and thus of primarily heuristic value for human subjects. Furthermore, these models have rarely been tested empirically according to the dysfunctional processes of interest (e.g. fear conditioning). In accordance with behavioural studies on fear conditioning (Lissek et al. Reference Lissek, Rabin, McDowell, Dvir, Bradford, Geraci, Pine and Grillon2009), altered neural processing of safety cues in PD has been suggested, with less activation during instructed threat and increased activity during the safe condition in the subgenual cingulate, ventral striatum and extended amygdala, and in the midbrain PAG (Tuescher et al. Reference Tuescher, Protopopescu, Pan, Cloitre, Butler, Goldstein, Root, Engelien, Furman, Silverman, Yang, Gorman, LeDoux, Silbersweig and Stern2011).
Based on these findings we aimed to elucidate neural substrates of exteroceptive fear conditioning in PD/AG patients. Regarding the still undefined nature of the learning deficit, we tested different hypotheses focusing on a particular conditioning process; that is, enhanced differential conditioning, enhanced simple conditioning and altered safety signal processing. Based upon previous work on the neural substrates of fear conditioning in general (Sehlmeyer et al. Reference Sehlmeyer, Schoning, Zwitserlood, Pfleiderer, Kircher, Arolt and Konrad2009), and altered safety signal processing in PD (Tuescher et al. Reference Tuescher, Protopopescu, Pan, Cloitre, Butler, Goldstein, Root, Engelien, Furman, Silverman, Yang, Gorman, LeDoux, Silbersweig and Stern2011), we expected patients to exhibit enhanced neural activity in fear circuit structures according to the above-described conditioning processes encompassing the amygdala, thalamus, midbrain, insula, ACC and prefrontal cortex (PFC) when compared to controls. A positive association with anxiety sensitivity, which has been suggested as a subclinical trait marker of PD (Schmidt et al. Reference Schmidt, Zvolensky and Maner2006; Bernstein et al. Reference Bernstein, Zvolensky, Marshall and Schmidt2009), was expected.
Method
Subjects
The study was part of the national research network PANIC-NET (Gloster et al. Reference Gloster, Wittchen, Einsle, Hofler, Lang, Helbig-Lang, Fydrich, Fehm, Hamm, Richter, Alpers, Gerlach, Strohle, Kircher, Deckert, Zwanzger and Arolt2009). Four centres (Aachen, Berlin-Charité, Dresden and Münster) participated in the fMRI study. Present data are based on a baseline assessment prior to treatment. Results on neural correlates of treatment-related changes have been reported elsewhere (Kircher et al. Reference Kircher, Arolt, Jansen, Pyka, Reinhardt, Kellermann, Konrad, Lueken, Gloster, Gerlach, Ströhle, Wittmann, Pfleiderer, Wittchen and Straube2013). Quality controlled data from 60 patients and 60 healthy controls were included in the analysis. Patients and controls were matched for age (± 5 years), gender and handedness (Edinburgh Handedness Inventory, EHI; Oldfield, 1971). Patient inclusion criteria encompassed a primary diagnosis of PD/AG according to DSM-IV-TR criteria as assessed by the Composite International Diagnostic Interview (CAPI-WHO-CIDI; DIAX-CIDI version; Wittchen & Pfister, Reference Wittchen and Pfister1997), which was validated by clinical experts, a score ⩾18 on the structured interview guide for the Hamilton Anxiety Rating Scale (SIGH-A; Shear et al. Reference Shear, Vander Bilt, Rucci, Endicott, Lydiard, Otto, Pollack, Chandler, Williams, Ali and Frank2001), a score ⩾4 at the Clinical Global Impressions Scale (CGI; Guy, Reference Guy1976), and age between 18 to 65 years. Clinically significant suicidal intent, any psychotic or bipolar disorder, borderline personality disorder, current drug dependence or a medical disease that could explain the patient's symptoms were followed by exclusion. Other current co-morbid diagnoses, including unipolar depression and other anxiety disorders, were allowed unless they were of primary clinical concern. As such, this sample can be considered as both relatively severe and representative of patients seen in clinical practice. Patients had to discontinue all psychopharmacological medication; patients on psychotropic medication underwent a wash-out period of 4 weeks before inclusion. Control subjects were free of current or past medical, neurological or psychiatric illness, as evidenced by the CAPI-WHO-CIDI; DIAX-CIDI version. Exclusion criteria for both groups were pregnancy, cardiac pacemaker, ferromagnetic metal implants, tattoos or permanent make-up with ferromagnetic colours. After complete description of the study, subjects provided informed written consent. The study was approved by the ethics committees of all participating centres.
fMRI task
The task design has been described in detail elsewhere (Reinhardt et al. Reference Reinhardt, Jansen, Kellermann, Schuppen, Kohn, Gerlach and Kircher2010; Kircher et al. Reference Kircher, Arolt, Jansen, Pyka, Reinhardt, Kellermann, Konrad, Lueken, Gloster, Gerlach, Ströhle, Wittmann, Pfleiderer, Wittchen and Straube2013). In brief, we used a differential fear conditioning task that consisted of three phases: familiarization (F) with 16 trials, acquisition (A) with 32 trials, and extinction (E) with 16 trials of each CS [coloured geometrical forms; presentation time 2000 ms with a variable inter-trial interval (ITI) of 4.785 to 7.250 s] and an aversive tone (white noise; 100 ms) as the US between 70 and 105 dB. Depending on individual judgements of aversiveness, subjects scoring <5 on a 10-point Likert scale were excluded to control for potential non-responders. In the acquisition, the US was paired pseudo-randomly with one of the CSs (counterbalanced between subjects; partial reinforcement rate of 50%), resulting in equal proportions of CS+ paired and CS+ unpaired trials. To avoid confounding effects between the CS+ and US processing, only CS+ unpaired trials were analysed during acquisition. After each phase, subjective valence and arousal ratings using the Self-Assessment Manikin (SAM; Bradley & Lang, Reference Bradley and Lang1994) for both CSs were obtained using a five-point Likert scale (for valence: 1, ‘very unpleasant’ to 5, ‘very pleasant’; and for arousal: 1, ‘not arousing’ to 5, ‘very arousing’). Because of technical problems, the ratings of one patient and two healthy control subjects were missing. Task duration was 16 min 49 s. Stimuli were presented by MR-compatible LCD goggles or back-projection systems and standard headphones using Presentation 11 (Neurobehavioral Systems; www.neurobs.com).
fMRI data acquisition and analysis
A detailed description of measures for quality control in this multicentre study is given in Kircher et al. (Reference Kircher, Arolt, Jansen, Pyka, Reinhardt, Kellermann, Konrad, Lueken, Gloster, Gerlach, Ströhle, Wittmann, Pfleiderer, Wittchen and Straube2013). Images were acquired using 3-T Philips Achieva (Aachen and Münster), 3-T Siemens Trio (Dresden) and 3-T General Electric Healthcare (Berlin) scanners. Five-hundred and five axial functional images [echo-planar imaging (EPI): matrix 64 × 64, 30 slices interleaved, field of view (FOV) = 230, voxel size = 3.6 × 3.6 × 3.8 mm, echo time (TE) = 30 ms, repetition time (TR) = 2 s] covering the whole brain and positioned parallel to the intercomissural line (AC–PC) were recorded, along with a three-dimensional (3D) structural data set [magnetization prepared rapid gradient echo (MPRAGE): matrix 128 × 112, 88 slices, FOV = 256, voxel size = 2 × 2 × 2 mm, TE = 3.93 ms, TR = 1100 ms, flip angle = 9°]. MR images were analysed using Statistical Parametric Mapping (SPM5; www.fil.ion.ucl.ac.uk) implemented in MATLAB 6.5 (Mathworks Inc., USA). The first five volumes were discarded to minimize T1 saturation effects. Data were filtered to 1/128 Hz to remove low-frequency noise. Slice time correction was performed, shifting the signal measured in each slice relative to the acquisition time of the middle slice. Functional images were temporally and spatially aligned and normalized into standard stereotactic space (2 × 2×2 mm). To account for differences in intrinsic smoothness between scanners, an iterative smoothness equalization procedure (Friedman et al. Reference Friedman, Glover, Krenz and Magnotta2006) was performed using 12-mm full-width at half-maximum (FWHM) Gaussian isotropic kernel smoothing. Thus, data from all centres were smoothed iteratively until a smoothness of 12-mm FWHM was reached, independent of scanner-specific intrinsic smoothness of the data. Assuming an intrinsic smoothness of 4–6 mm, a comparable smoothness would be obtained by applying a predefined kernel of 8-mm FWHM in a normal smoothing procedure.
At the first level, realignment parameters were included as regressors of no interest into the model to account for movement artefacts. The blood oxygen level-dependent (BOLD) response for each event type (CS+ paired, CS+ unpaired, CS−, US) and phase (F, A, E) was modelled by the canonical haemodynamic response function within the framework of the general linear model to analyse brain activation differences related to the onset of the different stimuli. Phases were further split into first and second halves (e.g. F1, F2, A1, A2, E1, E2). The second half of the familiarization (F2) was used as a baseline for CS+ and CS− for contrasts on simple conditioning and safety signal processing, assuming that orienting reactions should have decayed during the late phase. Regressors for the acquisition and extinction were again collapsed. Parameter estimates (β) and t statistic images were calculated. At the second level, a group analysis was performed by entering contrast images into a flexible factorial analysis, in which subjects were treated as random variables. fMRI centre variables were introduced as covariates of no interest to account for scanner differences between sites. Further covariates of no interest included education level, where we found group differences, and the Beck Depression Inventory Second Edition (BDI-II; Beck et al. Reference Beck, Steer and Brown1996), which might confound panic symptomatology in the current sample. However, analyses without the BDI-II score yielded comparable patterns of brain activation.
Contrasts of interest
We first tested for general conditioning effects in controls, patients and the combined sample in the acquisition and extinction phases (A: CS+ unpaired > CS−; E: CS+ unpaired > CS−). We then investigated group differences in differential fear conditioning (A: PD/AG > controls: CS+ unpaired > CS−; E: PD/AG > controls: CS+ unpaired > CS−), simple fear conditioning (A: PD/AG > controls: CS+ unpaired > CS+ familiarization; E: PD/AG > controls: CS+ unpaired > CS+ familiarization) and safety signal processing (A: PD/AG > controls: CS– > CS– familiarization; E: PD/AG > controls: CS– > CS– familiarization). Because of the lack of evidence on neural correlates of fear conditioning in PD/AG, a whole-brain analysis was carried out first. Post-hoc region-of-interest (ROI) analyses were performed for the amygdala to ensure that activity of the amygdala was not excluded because of the large cluster threshold. As early habituation of this structure has been reported (Büchel et al. 1998), amygdala activity during the first half of the acquisition phase was investigated separately. Estimated β values from activation clusters were extracted for illustration of box plots. Pearson's correlations were carried out between estimated β values and anxiety sensitivity scores as indicated by the Anxiety Sensitivity Index (ASI; Reiss et al. Reference Reiss, Peterson, Gursky and McNally1986). A Monte Carlo simulation was conducted to establish an appropriate voxel contiguity threshold (Slotnick et al. Reference Slotnick, Moo, Segal and Hart2003). This procedure relies on the fact that, given spurious activity or noise (voxel-wise type I error), the probability of observing increasingly large (spatially contiguous) clusters of activity decreases systematically. A cluster extent threshold can be enforced to ensure an acceptable probability of cluster-wise type I error (Slotnick & Schacter, Reference Slotnick and Schacter2004). This correction has the advantage of higher sensitivity to smaller effect sizes, while still correcting for multiple comparisons across the whole-brain volume. The result of the Monte Carlo simulations was based on the 2 × 2×2 mm interpolated voxels, the 12-mm smoothing kernel, the FOV and the number of slices. Assuming an individual voxel type I error of p < 0.005, a cluster extent of 142 contiguous resampled voxels was indicated as sufficient to correct for multiple voxel comparisons at p < 0.05. Thus, for all analyses, voxels with a significance level of p < 0.005 uncorrected belonging to clusters with at least 142 voxels are reported (see also Kircher et al. Reference Kircher, Arolt, Jansen, Pyka, Reinhardt, Kellermann, Konrad, Lueken, Gloster, Gerlach, Ströhle, Wittmann, Pfleiderer, Wittchen and Straube2013). For conjunction analyses against the null hypothesis (Nichols et al. Reference Nichols, Brett, Andersson, Wager and Poline2005), a minimum cluster size of 30 voxels was used.
Sample characteristics were tested using χ 2 and t tests (two-tailed). Subjective ratings were analysed with three-factorial ANOVAs for repeated measures and two-tailed pairwise comparisons with the two within-subject factors phase (F; A; E) and stimulus (CS+; CS−) and the between-subjects factor group (patients; controls). Post-hoc analyses were also conducted separately for patients and controls. Greenhouse–Geisser corrections were used when appropriate. An α level of p < 0.05 indicated statistical significance; analyses were carried out using IBM SPSS 19.0 (IBM Corp., USA).
Results
Sample characteristics and behavioural data
Sample characteristics are given in Table 1. Although educational level was lower in patients, groups were comparable in neuropsychological performance.
Table 1. Demographic and clinical characteristics of the sample
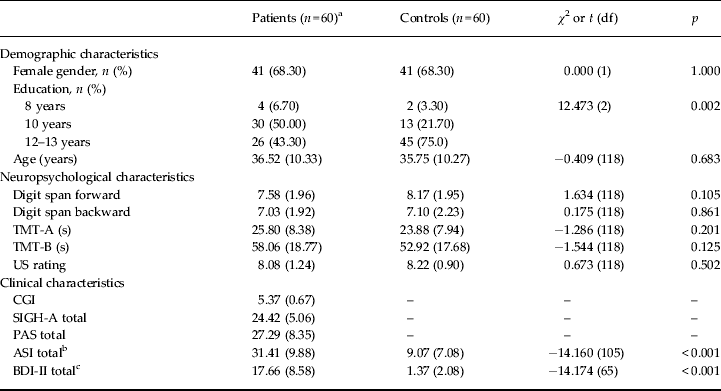
TMT-A, Trail Making Test Part A; TMT-B, Trail Making Test Part B; US, unconditioned stimulus (10-point Likert scale); CGI, Clinical Global Impressions Scale; SIGH-A, structured interview guide for the Hamilton Anxiety Rating Scale; PAS, Panic and Agoraphobia Scale; ASI, Anxiety Sensitivity Index; BDI-II, Beck Depression Inventory Second Edition; df, degrees of freedom.
a One patient participated in the functional magnetic resonance imaging (fMRI) study, but did not consent to the clinical trial.
b Available for n = 119.
c Available for n = 118.
Values given as mean (standard deviation) except where noted.
Regarding valence (higher values equal positive valence), a main effect of group indicated significantly lower ratings for patients throughout the entire experiment (F 1,115 = 17.011, p < 0.001). A significant main effect of phase was observed, with lower ratings after familiarization and acquisition than after extinction (F 2,209 = 7.712, p = 0.001; F, A < E, p < 0.05). The significant interaction effect phase × CS indicated that differential acquisition of contingencies had occurred, with CS+ ratings being significantly lower than CS– ratings after acquisition (F 2,218 = 10.132, p < 0.001; acquisition: CS+ < CS−, p = 0.003). Similar results were obtained for arousal ratings (higher values equal more arousal), with a main effect of group (F 1,115 = 26.747, p < 0.001; patients > controls), phase (F 2,278 = 3.778, p = 0.035; F, A < E, p < 0.05), CS (F 1,115 = 6.290, p = 0.014; CS+ > CS−) and interaction effect phase × CS (F 2,206 = 3.474, p = 0.038; A: CS+ > CS−, p = 0.002). Separate subgroup analyses showed that differences were mainly driven by controls, whereas patients did not discriminate CS+ and CS− by means of subjective ratings. No other significant main or interaction effects were observed (Fig. 1).

Fig. 1. Neural and behavioural markers of differential fear conditioning. (a) Brain activation during differential conditioning [acquisition phase: reinforced conditioned stimulus (CS+ unpaired) > non-reinforced conditioned stimulus (CS−)] in the combined sample. Clusters encompass the superior temporal gyri (extending to the anterior insula; left figure), precentral gyri and midbrain (right figure). (b) Behavioural data (valence and arousal ratings) on fear conditioning. *** p < 0.001.
fMRI results
Differential conditioning effects in the combined sample
In controls, we observed midbrain activation during the acquisition phase in response to the CS+ > CS−. In patients, a widespread network encompassing the bilateral insula, superior temporal gyrus, left precentral gyrus and right supplementary motor area was activated. In the combined sample, a large cortical and subcortical network encompassing the superior temporal gyrus [extending to the inferior frontal gyrus (IFG) and anterior insula], precentral gyrus, supplementary motor area and midbrain was activated during fear acquisition. Expression of conditioned fear during extinction revealed a cluster in the superior medial frontal gyrus extending to the medial orbitofrontal and anterior cingulate gyri (Table 2; Fig. 2).

Fig. 2. Group differences in brain activation during differential conditioning [reinforced conditioned stimulus (CS+ unpaired) > non-reinforced conditioned stimulus (CS−)], simple conditioning (CS+ unpaired > CS+ familiarization) and safety learning (CS– > CS– familiarization). Box plots on estimated β values from the cluster for each regressor are given. F2, the second half of the familiarization phase; A, the acquisition phase.
Table 2. Brain activation (clusters and cluster extensions) of fear conditioning for the combined patient and control sample and for patients > controls
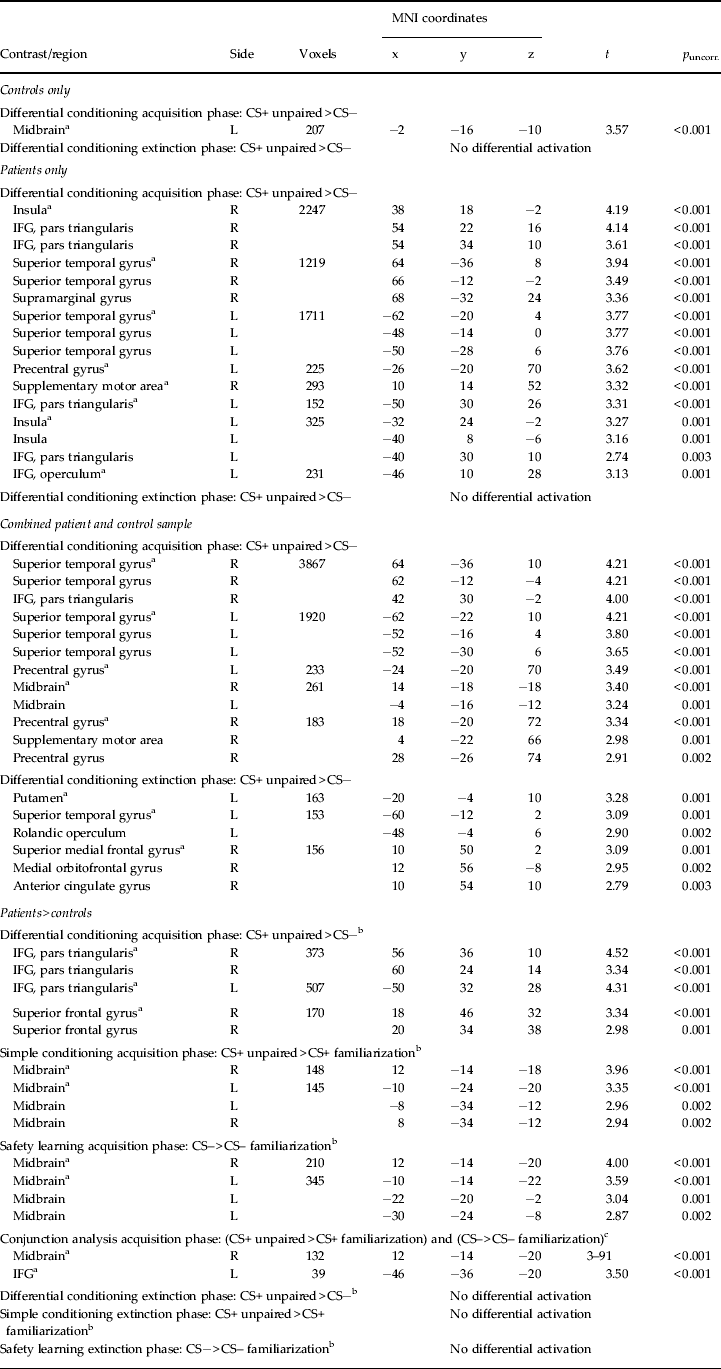
L, Left; R, right; CS+ unpaired, conditioned stimulus associated with the unconditioned stimulus (US) (unpaired trial only); CS−, CS not associated with the US; IFG, inferior temporal gyrus; Voxel, number of voxels per cluster; MNI, Montreal Neurological Institute;
a Cluster peak voxels.
b p < 0.005 (uncorrected) with a minimum cluster size of 142 contiguous voxels, indicating correction for multiple comparisons at p < 0.05.
c p < 0.005 (uncorrected) with a minimum cluster size of 30 contiguous voxels for the conjunction analysis.
Group differences in differential conditioning, simple conditioning and safety signal processing
Compared to controls, patients showed enhanced neural activation for differential conditioning during acquisition (PD/AG > controls: CS+ unpaired > CS−) in the bilateral dorsal IFG and right superior frontal gyrus. Bar graphs indicate that this effect was driven by enhanced activation towards the CS+ in patients compared to controls, who did not differ in the magnitude of brain activation in this cluster for both CSs. Simple conditioning (PD/AG > controls: CS+ unpaired > CS+ familiarization) and safety signal processing (PD/AG > controls: CS− > CS– familiarization) were associated with increased neural activity in the midbrain in patients (Table 2; Fig. 2). Again, this effect was driven by increased responding in patients towards the CS+ unpaired and CS− during acquisition when compared to the CS+ and CS− during late familiarization, whereas a relative deactivation was observed in controls. A conjunction analysis of the simple conditioning and safety signal processing contrasts confirmed that neural activity in the midbrain was enhanced during both CS+ unpaired and CS− processing in patients. No differential activation patterns for these contrasts were observed during extinction. Exploratory ROI analyses did not yield differential activity in the amygdala. We further explored amygdala activation in these contrasts of interest during the first half of the acquisition phase. In patients, increased activation during safety signal processing (A1: CS– > CS– familiarization) was observed in the left amygdala [Montreal Neurological Institute (MNI) coordinates: x = − 24, y = − 2, z = − 26; cluster size = 147, t = 3.34, p uncorr. <0.001] during early acquisition (Fig. 3). Controls showed a small cluster of amygdala activation during simple conditioning (A1: CS+ > CS+ familiarization) in the right amygdala (x = 38, y = 4, z = − 26; cluster size = 2, t = 2.65, p uncorr. = 0.004). Group comparisons did not yield any further amygdala activation.
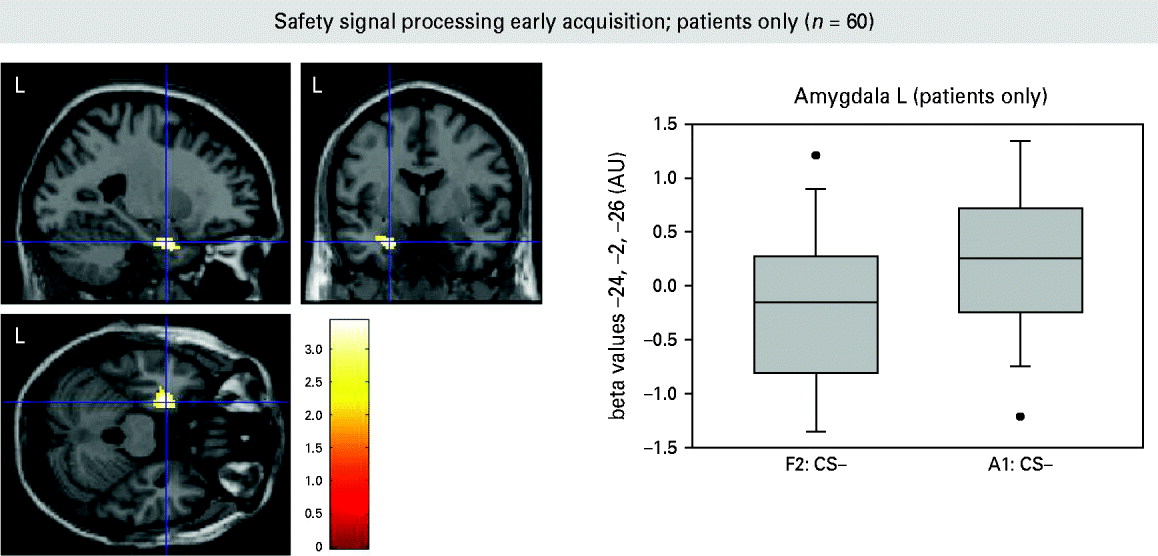
Fig. 3. Amygdala activation during safety signal processing [non-reinforced conditioned stimulus (CS–) > CS– familiarization] in the early acquisition phase (patients only). F2, the second half of the familiarization phase; A1, the first half of the acquisition phase stimulus; L, left.
Anxiety sensitivity was positively correlated with brain activation in the midbrain during simple conditioning, but not with activity in the dorsal IFG during differential conditioning (patients: r R IFG (56, 26, 10) – ASI = − 0.072, p = 0.586; r R midbrain (12, −14, −18) – ASI = 0.288, p = 0.027; r R midbrain (12, −14, −20) – ASI = 0.006, p = 0.964; controls: r R IFG (56, 26, 10) – ASI = − 0.074, p = 0.574; r R midbrain (12, −14, −18) – ASI = 0.297, p = 0.021; r R midbrain (12, −14, −20) – ASI = 0.0166, p = 0.205).
Discussion
Despite an increasing number of studies investigating the neural correlates of PD (for a review see Dresler et al. Reference Dresler, Guhn, Tupak, Ehlis, Herrmann, Fallgatter, Deckert and Domschke2012), relatively little is known about the neural correlates of fear conditioning in these patients. Behavioural studies have indicated deficient safety signal processing as a potential marker of PD (Lissek et al. Reference Lissek, Powers, McClure, Phelps, Woldehawariat, Grillon and Pine2005, Reference Lissek, Rabin, McDowell, Dvir, Bradford, Geraci, Pine and Grillon2009). Investigating different aspects of fear conditioning and their neural correlates in PD/AG, the present study yielded the following key results: patients showed enhanced bilateral dorsal IFG activation during differential conditioning whereas simple conditioning and safety signal processing were associated with enhanced midbrain activity in PD/AG patients. These data suggest altered top-down and bottom-up processing of fear conditioning in PD/AG.
Although subjective ratings indicated that successful acquisition of contingencies in the combined sample was driven mainly by controls, patients activated key structures of the neural network subserving fear conditioning, such as the bilateral insula, superior temporal gyrus and premotor cortex during differential conditioning. In the combined group, we observed a cortical and subcortical network encompassing the superior temporal gyrus (extending to the IFG and anterior insula), precentral gyrus, supplementary motor area and midbrain involved in differential conditioning. Expression of the conditioned response during extinction activated the putamen, superior medial frontal gyrus (extending to the medial OFC and ACC) and superior temporal gyrus, thus overlapping with previous observations on fear conditioning networks in the brain (Sehlmeyer et al. Reference Sehlmeyer, Schoning, Zwitserlood, Pfleiderer, Kircher, Arolt and Konrad2009). No amygdala activation was observed during differential conditioning in the entire group even when applying a less conservative ROI approach. Converging evidence from animal and human lesion studies exists that the amygdala is involved in fear acquisition (LeDoux et al. Reference LeDoux, Iwata, Cicchetti and Reis1988; Wilensky et al. Reference Wilensky, Schafe, Kristensen and LeDoux2006), but fMRI studies have demonstrated amygdala involvement inconclusively, possibly because of its fast habituation profile and different task characteristics (Sehlmeyer et al. Reference Sehlmeyer, Schoning, Zwitserlood, Pfleiderer, Kircher, Arolt and Konrad2009). Patients showed increased amygdala activity during safety signal processing. Regarding PD, other findings do not support the notion of a generally hyperactive amygdala (Ottaviani et al. Reference Ottaviani, Cevolani, Nucifora, Borlimi, Agati, Leonardi, De Plato and Brighetti2012), but the current results could indicate the relevance of altered safety signal processing that seems to activate structures associated with the detection of potential threat. This observation is in accordance with altered safety signal processing in behavioural studies (Lissek et al. Reference Lissek, Powers, McClure, Phelps, Woldehawariat, Grillon and Pine2005, Reference Lissek, Rabin, McDowell, Dvir, Bradford, Geraci, Pine and Grillon2009). It points to the importance of fear inhibition in the presence of safety signals, supplementing conventional conditioning analyses that are restricted to differential conditioning effects.
Comparing patients and controls, the results yielded increased activation along the neuraxis in response to the conditioned stimuli, ranging from prefrontal (IFG) to midbrain structures, as a neural marker of PD/AG. The right, but also the left, IFG has been associated with the inhibition of motor responses during stop-signal tasks (Aron et al. Reference Aron, Fletcher, Bullmore, Sahakian and Robbins2003; Swick et al. Reference Swick, Ashley and Turken2008). A recent study reported activity in the right IFG pars triangularis to stimuli associated with stop signals, suggesting that activity in this brain region can be triggered through associative learning (Lenartowicz et al. Reference Lenartowicz, Verbruggen, Logan and Poldrack2011). Hyperactivation in the bilateral IFG in PD/AG patients could be interpreted in terms of increased behavioural inhibition in the presence of a stimulus signalling potential threat. Whereas the IFG is associated with behavioural inhibition, the midbrain PAG is an integral part of the brain system mediating defensive reactivity under threat (McNaughton & Corr, Reference McNaughton and Corr2004; Brandao et al. Reference Brandao, Zanoveli, Ruiz-Martinez, Oliveira and Landeira-Fernandez2008). Somatic, autonomic and behavioural components of emotional reactions are coordinated within the PAG, which itself is controlled by extensive descending fibres from the medial and orbital PFC, ACC, insula, amygdala and hypothalamus (Amaral et al. Reference Amaral, Price, Pitkänen, Carmichael and Aggleton1992; An et al. 1998). Although conventional anatomical masking usually does not allow for exact labelling of the PAG or its substructures (e.g. dorsal or ventral parts), MNI coordinates (conjunction analysis: x = 12, y = − 14, z = − 20) were similar to those reported by others on midbrain alterations in PD patients (Protopopescu et al. Reference Protopopescu, Pan, Tuescher, Cloitre, Goldstein, Engelien, Yang, Gorman, LeDoux, Stern and Silbersweig2006: x = 6, y = − 15, z = − 18; Uchida et al. Reference Uchida, Del Ben, Busatto, Duran, Guimaraes, Crippa, Araujo, Santos and Graeff2008: x = − 11, y = − 17, z = − 22; Tuescher et al. Reference Tuescher, Protopopescu, Pan, Cloitre, Butler, Goldstein, Root, Engelien, Furman, Silverman, Yang, Gorman, LeDoux, Silbersweig and Stern2011: x = 6, y = − 24, z = − 18), indicating that this area of the midbrain might be involved in the pathophysiology of PD. Based on animal studies, neurophysiological models of PD state that the midbrain PAG is a key area involved in panic behaviour, indicating increased defensive reactivity in these patients (Lovick, Reference Lovick2000; Brandao et al. Reference Brandao, Zanoveli, Ruiz-Martinez, Oliveira and Landeira-Fernandez2008; Graeff & Del Ben, Reference Graeff and Del Ben2008). Human structural and functional imaging studies have further corroborated this notion, showing increased BOLD responses in the midbrain during safety signal processing (Tuescher et al. Reference Tuescher, Protopopescu, Pan, Cloitre, Butler, Goldstein, Root, Engelien, Furman, Silverman, Yang, Gorman, LeDoux, Silbersweig and Stern2011) and increased metabolic activity during anticipatory anxiety (Boshuisen et al. Reference Boshuisen, Ter Horst, Paans, Reinders and Den Boer2002), in addition to enhanced grey matter volume in the midbrain and pons in PD patients (Protopopescu et al. Reference Protopopescu, Pan, Tuescher, Cloitre, Goldstein, Engelien, Yang, Gorman, LeDoux, Stern and Silbersweig2006; Uchida et al. Reference Uchida, Del Ben, Busatto, Duran, Guimaraes, Crippa, Araujo, Santos and Graeff2008; Fujiwara et al. Reference Fujiwara, Yoshida, Otsuka, Hayano, Asami, Narita, Nakamura, Inoue and Hirayasu2011).
Some studies suggest that the neural substrates of defensive reactions are organized along a functional gradient including higher forebrain areas and midbrain structures mediating ‘hard-wired’ imminent threat reactions (McNaughton & Corr, Reference McNaughton and Corr2004; Mobbs et al. Reference Mobbs, Marchant, Hassabis, Seymour, Tan, Gray, Petrovic, Dolan and Frith2009). A shift from prefrontal to midbrain PAG activity has been reported with decreasing predatory distance (Mobbs et al. Reference Mobbs, Petrovic, Marchant, Hassabis, Weiskopf, Seymour, Dolan and Frith2007). When confronted with the potential presence of an exteroceptive threat (US), patients activated defensive system structures associated with imminent threat processing (as indicated by midbrain activity) in response to both CSs, whereas relative differences between these stimuli were related to response inhibition (dorsal IFG), which possibly indicates threat evaluation processes such as risk assessment or behavioural inhibition (McNaughton & Corr, Reference McNaughton and Corr2004). In line with these results, we interpret our current findings in terms of altered top-down (risk assessment or behavioural inhibition) and bottom-up (defensive reactivity) processing during fear conditioning in PD/AG. This interaction may also indicate the interplay between instrumental (behavioural inhibition) and Pavlovian conditioning as reflected by IFG and midbrain activation, by which conditioned fear drives behavioural avoidance, a hypothesis that could be tested with instrumental conditioning designs. In contrast to behavioural studies (Michael et al. Reference Michael, Blechert, Vriends, Margraf and Wilhelm2007), we did not observe altered neural processing of extinction learning because group differences were restricted to the acquisition phase. It should be noted that the present task focused on fear learning; future studies should investigate extinction learning and recall in more detail.
Midbrain activity during simple conditioning correlated with anxiety sensitivity in patients and controls. Findings indicate that high-risk markers of panic behaviour such as anxiety sensitivity are related to midbrain activity during the processing of stimuli signalling potential threat, but studies on high-risk samples are warranted to examine this hypothesis further.
Our findings should be interpreted within the study limitations. First, no autonomic marker or US expectancy ratings indicating successful conditioning were available, and the choice of an auditory US and partial reinforcement rate could have resulted in relatively mild conditioning effects. Sympathetic indicators such as skin conductance (SC) or startle would have been helpful in further interpreting the present findings on defensive reactivity in PD/AG. However, the paradigm itself has been validated in control subjects combining fMRI and SC (Reinhardt et al. Reference Reinhardt, Jansen, Kellermann, Schuppen, Kohn, Gerlach and Kircher2010). Regarding this particular patient population that is very vulnerable to the stress-eliciting properties of the scanner setting itself (Lueken et al. Reference Lueken, Muehlhan, Wittchen, Kellermann, Reinhardt, Konrad, Lang, Wittmann, Strohle, Gerlach, Ewert and Kircher2011), there is a trade-off between the induction of conditioning effects on the one hand and patient compliance on the other. We tried to control for non-responders by excluding subjects below a certain threshold in the aversiveness rating. Ratings were furthermore comparable between patients and controls. Second, no consolidation phases were included in the present task, thus possibly accounting for the missing group differences in the extinction phase. Future studies should consider delayed extinction tasks as a valuable alternative, although the feasibility of fMRI study designs requiring assessments on consecutive days needs to be proven in this patient group. Third, missing a patient control group, the specificity of alterations in fear conditioning for PD/AG has not yet been tested. Studies applying comparative designs on different anxiety disorders or on high-risk samples are warranted. Finally, the relevance of anxiety sensitivity could be examined in more detail using other experimental approaches closely related to the pathophysiology of PD, such as interoceptive conditioning.
In conclusion, patients showed enhanced processing of differential conditioning in the dorsal IFG, while at the same time exhibiting enhanced responses to either the CS+ unpaired or CS− in the midbrain. This activation pattern might indicate altered top-down and bottom-up processing during fear conditioning in PD/AG. Our findings can be interpreted within a neural framework of defensive reactivity being hierarchically organized from prefrontal (potential threat) to midbrain (proximal threat) defensive mechanisms that seems to play a key role in the aetiopathogenesis of PD.
Appendix
Centres
Principal investigators (PIs) with respective areas of responsibility in the Mechanisms of Action in CBT (MAC) study are V. Arolt (Münster: overall MAC programme coordination), H. U. Wittchen (Dresden: PI for the randomized clinical trial and manual development), A. Hamm (Greifswald: PI for psychophysiology), A. L. Gerlach (Münster: PI for psychophysiology and panic subtypes), A. Ströhle (Berlin: PI for experimental pharmacology), T. Kircher (Marburg: PI for functional neuroimaging) and J. Deckert (Würzburg: PI for genetics). Additional site directors in the RCT component of the programme are G. W. Alpers (Würzburg), T. Fydrich and L. Fehm (Berlin-Adlershof) and T. Lang (Bremen).
Staff members by site
Greifswald (coordinating site for psychophysiology): C. Melzig, J. Richter, S. Richter, M. von Rad; Berlin-Charité (coordinating centre for experimental pharmacology): H. Bruhn, A. Siegmund, M. Stoy, A. Wittmann; Berlin-Adlershof: I. Schulz; Münster (overall MAC programme coordination, genetics and functional neuroimaging): A. Behnken, K. Domschke, A. Ewert, C. Konrad, B. Pfleiderer, C. Uhlmann, P. Zwanzger; Münster (coordinating site for psychophysiology and subtyping): J. Eidecker, S. Koller, F. Rist, A. Vossbeck-Elsebusch; Marburg/Aachen (coordinating centre for functional neuroimaging): B. Drüke, S. Eskens, T. Forkmann, S. Gauggel, S. Gruber, A. Jansen, T. Kellermann, I. Reinhardt, N. Vercamer-Fabri; Dresden (coordinating site for data collection, analysis and the RCT): F. Einsle, C. Froehlich, A. T. Gloster, C. Hauke, S. Heinze, M. Hoefler, U. Lueken, P. Neudeck, S. Preiß, D. Westphal; Würzburg Psychiatry Department (coordinating centre for genetics): A. Reif, C. Gagel; Würzburg Psychology Department: J. Duerner, H. Eisenbarth, A. B. M. Gerdes, H. Krebs, P. Pauli, S. Schad, N. Steinhäuser; Bremen: V. Bamann, S. Helbig-Lang, A. Kordt, P. Ley, F. Petermann, E.-M. Schroeder. Additional support was provided by the coordinating centre for clinical studies in Dresden (KKS Dresden): X. Graehlert and M. Käppler.
Acknowledgements
This work is part of the German multicentre MAC study, which is funded by the German Federal Ministry of Education and Research (BMBF; project no. 01GV0615; neuroimaging study: project no. 01GV0611) as part of the BMBF Psychotherapy Research Funding Initiative. (The study was registered with the ISRCTN: ISRCTN80046034.) The RTC project was approved by the Ethics Committee of the Medical Faculty of the Technische Universität Dresden (EK 164082006). The neuroimaging components were approved by the Ethics Committee of the Medical Faculty of the Rheinisch-Westfaehlische Hochschule University Aachen (EK 073/07) and at all local sites. The experimental pharmacology study was approved by the Ethics Committee of the state of Berlin (EudraCT: 2006-00-4860-29).
Declaration of Interest
V. Arolt is member of advisory boards and/or gave presentations for the following companies: AstraZeneca, Janssen-Organon, Lilly, Lundbeck, Servier, Pfizer and Wyeth. He also received research grants from AstraZeneca, Lundbeck and Servier. He chaired the committee for the ‘Wyeth Research Award Depression and Anxiety’. J. Deckert received in the past three years honoraria by Janssen, Bristol Myers-Squibb, Wyeth, Lundbeck, AstraZeneca and Pfizer and grant support by Medice, Lundbeck and AstraZeneca. T. Kircher received fees for educational programmes from Janssen-Cilag, Eli Lilly, Servier, Lundbeck, Bristol Myers Squibb, Pfizer and AstraZeneca; travel support/sponsorship for congresses from Servier; speaker's honoraria from Janssen-Cilag; and research grants from Pfizer and Lundbeck. C. Konrad received fees for an educational programme from esparma GmbH/Aristo Pharma GmbH, Lilly Deutschland GmbH, Servier Deutschland GmbH, and MagVenture GmbH. A. Reif has received a research grant from AstraZeneca. A. Ströhle received research funding from the German Federal Ministry of Education and Research, the European Commission (FP6) and Lundbeck, and speaker honoraria from AstraZeneca, Boehringer Ingelheim, Eli Lilly & Co, Lundbeck, Pfizer, Wyeth and UCB. Educational grants were given by the Stifterverband für die Deutsche Wissenschaft, the Berlin Brandenburgische Akademie der Wissenschaften, the Boehringer Ingelheim Fonds and the Eli Lilly International Foundation. H.-U. Wittchen has served as a general consultant (non-product related) for Pfizer, Organon, Servier and Essex Pharma and has received grant funding for his institution from Sanofi Aventis, Pfizer, Lundbeck, Novartis, Essex Pharma, Servier and Wyeth.