Introduction
Recently, biotypes of Sumatran fleabane [Conyza sumatrensis (Retz.) E. Walker] exhibiting an unusual contact-type herbicide symptom after application of 2,4-D in mixture with glyphosate were detected in Paraná State, Brazil, after burndown application before soybean [Glycine max (L.) Merr.] sowing. In those plants, the classical epinasty symptoms in response to 2,4-D were not observed, and the plants exhibited, within a few hours, rapid leaf-cell death, visually similar to necrosis. After approximately 10 d, regrowth occurred, resulting in plant survival and seed production.
Herbicide resistance in the genus Conyza has been documented to the following modes of action: inhibitors of photosystem I and II, enolpyruvylshikimate phosphate synthase (EPSPS), and acetolactate synthase (ALS) (Fuerst et al. Reference Fuerst, Nakatani, Dodge and Arntzen1985; Kruger et al. Reference Kruger, Davis, Weller, Stachler, Loux and Johnson2009; Lehoczki et al. Reference Lehoczki, Laskay, Polos and Mikulás1984; VanGessel Reference VanGessel2001). Multiple resistance has been reported to glyphosate (EPSPS inhibitor) and chlorimuron-ethyl (ALS inhibitor) (Santos et al. Reference Santos, Oliveira, Constantin, Francischini and Osipe2014) and to glyphosate, chlorimuron-ethyl, and paraquat (Heap Reference Heap2019). Chemical alternatives in use involve inhibitors of glutamine synthetase, as glufosinate-ammonium, and mainly the synthetic auxins dicamba and 2,4-D (Flessner et al. Reference Flessner, McElroy, Mccurdy, Toombs, Wehtje, Burmester, Price and Ducar2015; Soares et al. Reference Soares, Oliveira, López-Ovejero and Christoffoleti2012). The evolution of resistance to glyphosate and ALS inhibitors possibly resulted in the increase of the selection pressure of 2,4-D in C. sumatrensis in southern Brazil.
Auxinic herbicides have been used as effective weed control agents since the introduction of 2,4-D herbicides in 1945 (Smith Reference Smith1989). Despite resistance to 2,4-D being reported as early as 1957 in wild carrot (Daucus carota L.) and spreading dayflower (Commelina diffusa Burm. f.) (Hilton Reference Hilton1957; Switzer Reference Switzer1957), the number of auxin-resistant weed species has been considered low based on long worldwide use of 2,4-D (Heap Reference Heap2019; Peterson et al. Reference Peterson, McMaster, Riechers, Skelton and Stahlman2016). Resistance to auxinic herbicides has been studied in several weeds such as prickly lettuce (Lactuca serriola L.), wild mustard (Sinapis arvensis L.), wild radish (Raphanus raphanistrum L.), and yellow starthistle (Centaurea solstitialis L.) (Burke et al. Reference Burke, Yenish, Pittmann and Gallagher2009; Hall et al. Reference Hall, Alam and Murr1993; Jugulam et al. Reference Jugulam, Dimeo, Veldhuis, Walsh and Hall2013; Valenzuela-Valenzuela et al. Reference Valenzuela-Valenzuela, Lownds and Sterling2001; Wang et al. Reference Wang, Deshpande and Hall2001). Different mechanisms have been implicated in resistance or tolerance to 2,4-D, such as decreased absorption in ground ivy (Glechoma hederacea L.) (Kohler et al. Reference Kohler, Throssell and Reicher2004), reduced translocation in R. raphanistrum and common hempnettle (Galeopsis tetrahit L.) (Goggin et al. Reference Goggin, Cawthray and Powles2016; Weinberg et al. Reference Weinberg, Stephenson, McLean and Hall2006), increased metabolism of 2,4-D in waterhemp [Amaranthus tuberculatus (Moq.) J. D. Sauer] (Figueiredo et al. Reference Figueiredo, Leibhart, Reicher, Tranel, Nissen, Westra, Bernards, Kruger, Gaines and Jugulam2018), and differential binding to auxin-binding proteins (Mockaitis and Estelle Reference Mockaitis and Estelle2008). To date, resistance to 2,4-D has been reported in 26 weed species, as well as seven cases of dicamba resistance, four cases of fluroxypyr resistance, and three cases of picloram resistance (Heap Reference Heap2019). The specific mechanisms of resistance are unknown for the majority of cases.
Two main types of auxin receptors are known, ABP1 and the complex SCFTIR1/AFB (Grones and Friml Reference Grones and Friml2015). ABP1 is believed to be involved in fast responses to auxin (Dahlke et al. Reference Dahlke, Luethen and Steffens2010), and the complex SCFTIR1/AFB acts in combination with the auxin regulators Aux/IAAs in the transcriptional response to auxins (Mockaitis and Estelle Reference Mockaitis and Estelle2008). The herbicide 2,4-D is thought to bind to the auxin receptor TIR1 or some analogue, causing higher expression of auxin-responsive genes, leading to increased synthesis of ethylene and abscisic acid (ABA) (Grossmann Reference Grossmann2010; Song Reference Song2014). Inability of plants to modulate the concentration of 2,4-D results in continuous auxin signaling, leading to the classical symptoms of epinasty, followed by growth inhibition and death (Grossmann Reference Grossmann2010). The resistance to auxinic herbicides related to lower translocation (Mithila et al. Reference Mithila, Hall, Johnson, Kelley and Riechers2011; Riar et al. Reference Riar, Burke, Yenish, Bell and Gill2011; Weinberg et al. Reference Weinberg, Stephenson, McLean and Hall2006) might involve changes in influx auxin transporters AUX1/LAX (AUXIN RESISTANT 1/LIKE AUX1), efflux-transporters PIN (PIN-FORMED), and ABC transporters (Cho and Cho Reference Cho and Cho2013; Titapiwatanakun and Murphy Reference Titapiwatanakun and Murphy2009). In Arabidopsis thaliana, mutations in genes coding for auxin receptors and transporters can generate plants resistant to auxinic herbicides (Kubes et al. Reference Kubes, Yang, Richter, Cheng, Młodzińska, Wang, Blakeslee, Carraro, Petrášek, Zažímalová, Hoyerová, Peer and Murphy2012; Roux and Reboud Reference Roux and Reboud2005; Walsh et al. Reference Walsh, Neal, Merlo, Honma, Hicks, Wolff, Matsumura and Davies2006; Yu and Wen Reference Yu and Wen2013). However, the role of these proteins in causing resistance to auxinic herbicides in weeds has not yet been characterized.
About 33 dicotyledonous weed species have been reported as having evolved resistance to auxinic herbicides (Heap Reference Heap2019). In general, resistant individuals are able to produce asymptomatic new growth within a week of herbicide application. Variation of 2,4-D effects in the genus Conyza has been described by studies developed by Kruger et al. (Reference Kruger, Davis, Weller and Johnson2008, Reference Kruger, Davis, Weller and Johnson2010), in which an accession was able to survive and produce seeds after treatment with 560 g ae ha−1 of 2,4-D. In these studies, the resistance mechanism was not described, but abnormal symptomology has not been reported.
The symptoms described here in biotypes of C. sumatrensis are similar to those defined as rapid response or hypersensitive response, identified in giant ragweed (Ambrosia trifida L.) resistant to glyphosate (Brabham et al. Reference Brabham, Gerber and Johnson2011; Jeffery Reference Jeffery2014; Lespérance Reference Lespérance2015; Van Horn et al. Reference Van Horn, Moretti, Robertson, Segobye, Weller, Young, Johnson, Schulz, Green, Jeffery, Lespérance, Tardif, Sikkema, Hall and McLean2017). Rapid cell death and H2O2 accumulation were found in leaves of this accession of A. trifida after glyphosate application, symptoms similar to those seen with photosystem I inhibitors (Jeffery Reference Jeffery2014). Alterations in chloroplast volume and starch accumulation in photosynthetic tissues were also found (Lespérance Reference Lespérance2015). These symptoms were inhibited in the absence of light (Jeffery Reference Jeffery2014) and appeared to be related to programmed cell death (Lespérance Reference Lespérance2015). Recently, Moretti et al. (Reference Moretti, Van Horn, Robertson, Segobye, Weller, Young, Johnson, Douglas Sammons, Wang, Ge, d’Avignon, Gaines, Westra, Green and Jeffery2017) suggested that a source of carbon might be required to induce rapid necrosis symptoms. Higher concentrations of [14C]glyphosate in mature leaves and lower concentrations in apical meristems of plants showing rapid necrosis symptoms compared with susceptible plants at 24 h after spraying suggest reduced translocation is a possible resistance mechanism (Lespérance Reference Lespérance2015). The nomenclature of this new mechanism of resistance is not a consensus. The denomination of “apoptosis” is described as inappropriate in plants (Doorn et al. Reference Doorn, Beers, Dangl, Franklin-Tong, Gallois, Hara-Nishimura, Jones, Kawai-Yamada, Lam, Mundy, Mur, Petersen, Smertenko, Taliansky and Van Breusegem2011), and “hypersensitivity” is more adequate for response to pathogens (Roden and Ingle Reference Roden and Ingle2009). “Rapid response” is also used, but the response could be related to several other plant reactions not associated with cell and tissue death. The term “rapid necrosis” is associated with the acute cell death response that develops rapidly in plants (Proskuryakov et al. Reference Proskuryakov, Konoplyannikov and Gabai2003), which is more closely related to the first observed symptom in the 2,4-D–resistant plants. It will be useful to rename this process once the actual cause of this mechanism of resistance is identified.
In glyphosate-resistant A. trifida with rapid necrosis symptoms, it was observed that the rapid increase of H2O2 (a reactive oxygen species, or ROS) predates leaf necrosis (Moretti et al. Reference Moretti, Van Horn, Robertson, Segobye, Weller, Young, Johnson, Douglas Sammons, Wang, Ge, d’Avignon, Gaines, Westra, Green and Jeffery2017). The rise of ROS in response to auxins has also been demonstrated (Morré et al. Reference Morré, Brightman, Hidalgo and Navas1995; Pazmiño et al. Reference Pazmiño, Romero-Puertas and Sandalio2012; Peer et al. Reference Peer, Cheng and Murphy2013). Auxin-induced ROS production seems to be mediated, at least in part, by the NAD(P)H oxidase RbohD (Mangano et al. Reference Mangano, Denita-Juarez, Choi, Marzol, Hwang, Ranocha, Velasquez, Borassi, Barberini, Aptekmann, Muschietti, Nadra, Dunand, Cho and Estevez2017; Peer et al. Reference Peer, Cheng and Murphy2013), and it is a downstream signal for auxin (Schopfer et al. Reference Schopfer, Liszkay, Bechtold, Frahry and Wagner2002). However, ROS are also involved in auxin oxidation, an irreversible mechanism of auxin elimination (Peer et al. Reference Peer, Cheng and Murphy2013). Therefore, resistance to 2,4-D might be obtained by changing redox status. Consequences of ROS include lipid peroxidation followed by leaf necrosis. The rapid and deregulated production of ROS could be the mechanism causing the occurrence of rapid necrosis in plants that have resistance to herbicides.
The objectives of this study were to evaluate the occurrence of resistance to 2,4-D herbicide and to characterize the rapid necrosis as the mechanism of resistance in C. sumatrensis.
Materials and Methods
Plant Material, Screening and Symptom Characterization
Seeds of 10 C. sumatrensis plants were individually collected in the spring of 2016 in a crop field located in the city of Maripá, PR, Brazil (24.55°S, 53.72°W), where the herbicides 2,4-D and glyphosate were used in the burndown application. Plants in that area showed an atypical symptomatology of 2,4-D herbicide associated with the occurrence of rapid necrosis and resumption of plant growth similar to the effect of contact herbicides (Figure 1A). The susceptible control populations used in this study consisted of the accessions Londs4-s (23.33°S, 51.21°W) and SM (29.71°S, 53.73°W) collected in Londrina, PR, and Santa Maria, RS, Brazil, respectively. The procedure for seed germination started by soaking the seeds in water at 4 ± 2 C for 4 d. After that, the seeds were transferred to plastic trays (15 cm by 10 cm) containing substrate based on charcoal rice husks and vermiculite in a ratio of 1:1. Pots were maintained in a growth chamber (Percival, Boone, IA, USA) at a temperature of 23 ± 3 C and a photoperiod of 13 h (300 μmol m−2 s−1). Plants with one developed leaf were transplanted into 200-ml plastic pots with drainage holes containing a mixture (3:1) of the substrate described earlier and soil (classified as Hapludult, sandy clay loam, with 34% clay). The fertilizer NPK (5-20-10) was added at 5 g kg−1 of substrate and soil mixture. Six individual plants derived from each of the field-sampled plants were used for a total of 60 plants. The plants were maintained in a greenhouse at 25 ± 5 C and irrigated daily.

Figure 1. Conyza sumatrensis plants (A) under field conditions showing the rapid necrosis symptoms and (B) in the greenhouse after 2 h. The susceptible Londs4-s (left) and Marpr9-rn (right) accessions at 1 (C), 2 (D), and 21 (E) d after treatment of 806 g ha−1 of 2,4-D.
The initial screening was performed with the herbicide 2,4-D (DMA® 806 BR SL, Dow AgroSciences Industrial, São Paulo, SP, Brazil) at a dose of 804 g ae ha−1. The herbicide application was performed when the plants were between 7- and 12-cm tall (7 to 10 leaves) in a spray chamber (Generation III Research Sprayer, DeVries Manufacturing, Hollandale, MN, USA) calibrated at 262 kPa delivered by an 80.02E nozzle, resulting in an output volume equivalent to 200 L ha−1. The symptoms of rapid necrosis were monitored at 15, 30, 60, 120, 240, and 420 min and 1, 2, 4, 8, 12, 15, 21, and 28 d after treatment (DAT). After the last evaluation (28 DAT), the surviving plants with rapid necrosis were selected and transplanted to 5-kg pots. Before flowering, the plants were protected with a soft and fine tulle fabric to avoid pollen contamination and seed movement. The following studies were conducted using seeds from a single rapid necrosis surviving plant named Marpr9-rn.
Additional characterization of the rapid necrosis symptoms was performed regarding the effect of glyphosate and of the compounds present in the commercial 2,4-D formulation. The evaluation of the effect of glyphosate (Glizmax Prime® SL, Dow AgroSciences Industrial) was performed by application of the herbicide 2,4-D at doses of 40.2 and 402 g ae ha−1, glyphosate at 864 g ae ha−1, and 2,4-D at 402 g ae ha−1 in a tank mixture with glyphosate at 864 g ae ha−1. The evaluation of the effect of the 2,4-D formulation consisted of commercial 2,4-D herbicide (DMA® 806 BR), 2,4-D analytical product (ACS standard) with 98% purity (Sigma Aldrich, St Louis, MO, USA) diluted in a solution of water and ethanol 50/50%, and an untreated check. Both 2,4-D treatments were applied at a dose of 804 g ae ha−1. The experiment was conducted once, and the number of replicates was five. Plant growth and herbicide spraying conditions were similar to those described earlier. The plant injury evaluation was performed at 2, 4, and 8 h and 1, 3, 7, 14, and 21 DAT. Data analyses were performed using ANOVA followed by a Tukey’s test at 5%.
Dose–Response Curve to 2,4-D
The experiments were carried out with completely randomized design, in a bifactorial arrangement, with four replicates. The experiments were repeated, and results were also confirmed in plants from the second generation (seeds from the second autocross derived from single-seed descendants of the plants identified in the field). Factor A consisted of two 2,4-D–susceptible (Londs4-s and SM) and Marpr9-rn accessions. Factor B was the 2,4-D dosage: 0, 50.25, 100.5, 201, 402, 804, and 1,608 g ae ha−1. Herbicide spraying was performed as described earlier. The occurrence of symptoms of rapid necrosis was evaluated at 2 and 4 h and 1, 7, 14, 21, 28, 35, 42, and 49 DAT by scoring the visual injury at percentage scale, where 0% corresponded to absence of symptoms and 100% to total plant necrosis. The injury was converted to percent tolerance (green leaves) by calculating 100 − injury. Fresh and dry aboveground weight were evaluated at 49 DAT. Data were analyzed by ANOVA followed by a three-parameter nonlinear logistic model:

where y is the response expressed as percentage of the untreated control, a is the asymptotic value of y at the upper limit, b is the slope of the curve around the point of inflection, and x 0 is the dose required to reduce the y value by 50% (LD50) when a = 100. The software SigmaPlot v. 11 was used to perform the regressions. The resistance factor (RF) was obtained by the ratio of the LD50 values of the resistant and susceptible accessions.
Analyses of Cross-Resistance and Multiple Resistance
The experiments were carried out in a completely randomized design in a factorial arrangement with four independent biological replicates and were repeated twice. The doses used are described in Table 1. In all experiments, Factor A consisted of the known susceptible Londs4-s and the putative resistant Marpr9-rn. The cross-resistance experiment consisted of the herbicides 2,4-D, fluroxypyr (Prestige® EC, Dow AgroSciences Industrial), picloram (Padron® SL, Dow AgroSciences Industrial), florpyrauxifen-benzyl (Loyant™SL, Dow AgroSciences, Indianapolis, IN, USA), and halauxifen-methyl (Arylex™ SC, Dow AgroSciences).
Table 1. Herbicide and doses used in cross-resistance, dose–response, and multiple-resistance experiments.

a Dow AgroSciences Industrial Ltda., São Paulo, SP, Brazil; https://www.corteva.br.
b Dow AgroSciences LCC, Indianapolis, IN, USA; https://www.corteva.us.
c BASF Corporation, Durham, NC, USA; www.basf.com/usa.
d Bayer AG, Belford Roxo, RJ, Brazil; https://www.bayer.com.br.
e UPL do Brasil, Ituverava, SP, Brazil; https://br.uplonline.com.
f Syngenta, Paulínia, SP, Brazil; https://www.syngenta.com.br.
An additional cross-resistance dose−response experiment was carried out with the herbicides 2,4-D, dicamba (Clarity® SL, BASF, Durham, NC, USA), and triclopyr (Garlon 480 BR® EC, Dow AgroSciences Industrial). The visual injury was evaluated at 2, 14, and 49 DAT. Data were analyzed as described earlier for the dose−response curve for 2,4-D.
For the multiple-resistance experiment, Factor B was the herbicides 2,4-D, saflufenacil (Heat® WG, BASF, Guaratinguetá, SP, Brazil), chlorimuron-ethyl (Clorim WG, DVA Agro do Brasil, Barueri, SP, Brazil), paraquat (Gramoxone® SL, Syngenta, Paulínia, SP, Brazil), ammonium glufosinate (Finale® SL, Bayer, Belford Roxo, RJ, Brazil), and glyphosate (Glizmax Prime® SL, Dow AgroSciences Industrial). Factor C was the doses as described in Table 1. The herbicides were applied when the plants presented 10 to 15 leaves (10 to 14 cm). The spraying conditions and plant injury evaluations were the same as described earlier. The visual injury was evaluated at 2, 14, and 49 DAT. Fresh and dry aboveground weights were evaluated at 49 DAT. Data analyses for the cross- and multiple resistance were evaluated using ANOVA followed by a Tukey test at 5%.
Evaluation of Presence of H2O2 in Function of Light
The evaluation of the presence of H2O2 was performed using the 3,3′-diaminobenzidine-hydrochloride (DAB) staining method (Jeffery Reference Jeffery2014; Moretti et al. Reference Moretti, Van Horn, Robertson, Segobye, Weller, Young, Johnson, Douglas Sammons, Wang, Ge, d’Avignon, Gaines, Westra, Green and Jeffery2017). In the first experiment, the treatments consisted of the susceptible Londs4-s and Marpr9-rn accessions at the 8- to 10-leaf stage treated with 804 g ae ha−1 of 2,4-D, with paraquat at 600 g ai ha−1 as the positive control and a negative untreated control. The application of the herbicides was performed as already described. Mature leaves were sampled by cutting the petiole base with a scalpel at 7 h after treatment (HAT).
In the second experiment, two 12-mm-diameter leaf disks were collected from the fifth and sixth leaves using a metal hole puncher after 804 g ae ha−1 of 2,4-D spraying at time intervals of 0, 15, 30, 60, 120 240, 420, and 1440 min after treatment. Plants were continuously exposed to high (848 μmol m−2 s−1) and low light (29 μmol m−2 s−1) in a greenhouse with a temperature of 25 ± 5 C. The low-light treatment was obtained with two layers of black mesh with 80% shading. The radiation measure was evaluated at 1200 hours on a sunny day, and the light sensor was positioned at plant level. Each experiment was conducted once, with four replicates.
Leaf and leaf disks were infiltrated with DAB (1 mg ml−1, pH 3.8) and incubated in DAB solution at room temperature overnight. After incubation, samples were decolorized by being boiled in 90% ethanol for 10 min to remove chlorophyll before being photographed. The presence of H2O2 was visualized by color change (brown) where DAB polymerized with this compound. The analysis of the amount of area with DAB was carried out using the color deconvolution tool in Image J (Ruifrok and Johnston Reference Ruifrok and Johnston2001).
Results and Discussion
Screening and Symptom Characterization
Different mechanisms have been associated with resistance to the herbicide 2,4-D; however, to date, none have been connected with the symptoms of rapid necrosis. Therefore, the characterization of this new phenotype’s response to 2,4-D, as determined here, is the first report of the resistance mechanism. The symptoms of rapid necrosis were observed in all six replicates of nine out of the 10 field-sampled plants evaluated after application at 804 g ha−1 of 2,4-D in the Marpr9-rn population. The seeds were collected from plants under field conditions approximately 2 mo after spraying, and because of that, seeds from a susceptible plant may also have been sampled, resulting in absence of the rapid necrosis symptoms in one individual. The atypical symptoms of the rapid necrosis in the population Marpr9-rn were noticeable at approximately 2 h after 2,4-D application, with partial leaf wilt and light necrotic spots (Figure 1B), and after 4 h, leaf necrosis appeared mainly in the borders and tips (Figure 1C). At 1 DAT, the leaves presented total necrosis in the blade region (Figure 1D). The progression of the rapid necrosis symptoms was continuously observed, and at 21 DAT, plant growth resumed via development of axillary buds (Figure 1E). The symptoms were observed in the mature leaves and the meristems; rapid necrosis symptoms were absent from young leaves. The symptoms were more evident in the upper leaves, and the lower leaves presented only necrotic spots, probably due to lower herbicide interception. In plants of the susceptible accession, only the usual epinasty symptoms were observed. The symptoms observed in the Marpr9-rn plants are consistent to those of hypersensitivity to pathogens (Bestwick et al. Reference Bestwick, Brown and Mansfield1998) as well as those described by Brabham et al. (Reference Brabham, Gerber and Johnson2011) and Van Horn et al. (Reference Van Horn, Moretti, Robertson, Segobye, Weller, Young, Johnson, Schulz, Green, Jeffery, Lespérance, Tardif, Sikkema, Hall and McLean2017) in glyphosate-resistant A. trifida. The time required in the present study for the necrosis symptoms to appear in C. sumatrensis treated with the herbicide 2,4-D differed slightly from the time reported for A. trifida in response to glyphosate. The onset of necrosis occurred before 4 h after 2,4-D treatment. Van Horn et al. (Reference Van Horn, Moretti, Robertson, Segobye, Weller, Young, Johnson, Schulz, Green, Jeffery, Lespérance, Tardif, Sikkema, Hall and McLean2017) described necrosis occurring within 6 h after glyphosate application in A. trifida. However, a significant delay in the occurrence of the rapid necrosis occurs for plants growing at low radiation (Jeffery Reference Jeffery2014). In addition, the rapid necrosis symptoms caused by glyphosate in A. trifida is more pronounced in the older leaves, similar to what was observed in the present study. The apical meristem of the treated plants was not affected with rapid necrosis symptom.
The occurrence of rapid necrosis associated with 2,4-D has not been previously described. Therefore, to confirm 2,4-D as the causal agent of this symptomatology, additional evaluations were performed. Considering the similarities between rapid necrosis caused by 2,4-D and the effects of glyphosate in A. trifida, response to glyphosate (864 g ae ha−1) was evaluated; rapid necrosis was not observed (Figure 2), despite the occurrence of glyphosate resistance in C. sumatrensis in the region where seeds were sampled. Glyphosate has been extensively used at burndown and for POST application for Conyza spp. control. However, the symptoms of rapid necrosis identified in C. sumatrensis were caused only by 2,4-D, and this symptom also occurred when this herbicide was sprayed in mixture with glyphosate (Figure 2). The intensity and the time required for the onset of the rapid necrosis symptoms depended on the 2,4-D dose.
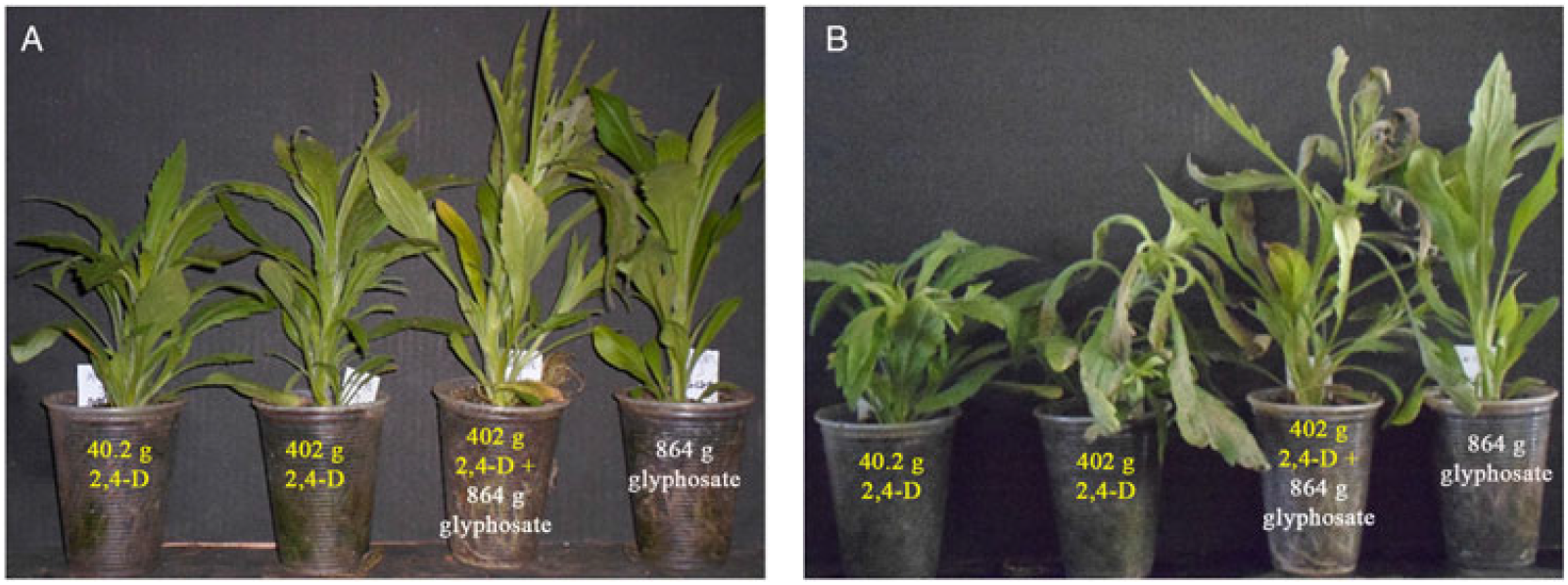
Figure 2. Plants of the Conyza sumatrensis Marpr9-rn accession at 4 h (A) and 1 d (B) after treatment of 2,4-D and glyphosate herbicides alone and in combination.
To confirm that rapid necrosis was a response to 2,4-D and not to any other component of the commercial herbicide formulation, a solution equivalent to 804 g ae ha−1 of the analytical product 2,4-D (ACS grade) diluted in ethanol 50% v/v was prepared. The rapid necrosis symptoms occurred similarly for applications of both the commercial and laboratory 2,4-D products (Figure 3B; Table 2). That indicates that other compounds used in the formulation of the herbicide 2,4-D herbicide (DMA™ 806 BR) are not associated with the rapid necrosis and that the 2,4-D molecule is the cause of this symptom.

Figure 3. Plants of the Conyza sumatrensis Londs4-s (susceptible) (A) and Marpr9-rn (B) accessions at 8 h after treatment of 804 g ha−1 of 2,4-D analytical product (reagent) diluted in ethanol 50% and of the commercial DMA formulation.
Table 2. Plant injury (%) in susceptible (Londs4-s) and resistant to 2,4-D with rapid necrosis (Marpr9-rn) accessions of Conyza sumatrensis in response to the application of the 2,4-D analytical product and commercial DMA formulation at 2, 14, and 21 d after treatment (DAT).

a Lowercase letters compare accessions in the same time (rows), and uppercase letters compare herbicide treatments (columns); P > 0.05 using Tukey’s test.
Dose–Response Curve to 2,4-D
The role of 2,4-D in the rapid necrosis symptoms having been confirmed, the increased tolerance obtained from rapid necrosis was determined. In the evaluation at 2 HAT, only drooping and initial slight necrotic spots symptoms were observed in the Marpr9-rn accession, even for the highest dose of 2,4-D (1,608 g ha−1). No visual symptoms were present in the susceptible Londs4-s accession (Figure 4A and B). At 4 HAT, rapid necrosis began to occur at doses equal to or higher than 100.5 g ha−1 of 2,4-D in the Marpr9-rn accession, and mild epinasty appeared in the susceptible Londs4-s (Figure 4C and D). In the evaluation performed at 1 DAT, the typical 2,4-D symptom of epinasty was clearly observed in the susceptible Londs4-s accession, but the more pronounced symptoms of rapid necrosis occurred in the Marpr9-rn accession (Figure 4E and F). Even in the lower leaves, where rapid necrosis was not present, epinasty symptoms were not observed, an indication that the 2,4-D herbicide may not have been translocated from the treated leaves. In the following evaluations, the resumption of growth of the Marpr9-rn plants was observed in contrast to the mortality of the susceptible accession Londs4-s (Figure 4G and H). The dose–response curves of the evaluations at 1, 14, and 49 DAT are shown in Supplementary Figure S1. The result of the rapid necrosis symptoms is the resumption of plant growth in the Marpr9-rn accession, indicated by the increasing RF. In the evaluation at 7 DAT, the RF was 1.16, and this value was 18.62 in the evaluation at 49 DAT (Table 3). In this evaluation, the LD50 was 1,133 g ae ha−1 of 2,4-D for the Marpr9-rn accession and 60.8 and 56.2 g ha−1 for the susceptible Londs4-s and SM accessions, respectively (Table 3).

Figure 4. Plants of the Conyza sumatrensis Londs4-s (susceptible) and Marpr9-rn accessions treated with 0, 50.25, 100.5, 201, 402, 804, and 1,608 g ae ha−1 of 2,4-D at 2 (A, B) and 4 h (C, D) and 1 (E, F) and 28 d (G, H) after treatment.
Table 3. Parameters of the logistic equation,a concentrations of the herbicide 2,4-D required for 50% reduction of 100 − visual injury (LD50) of Conyza sumatrensis, and resistance factor (RF) in the whole-plant dose–response studies.
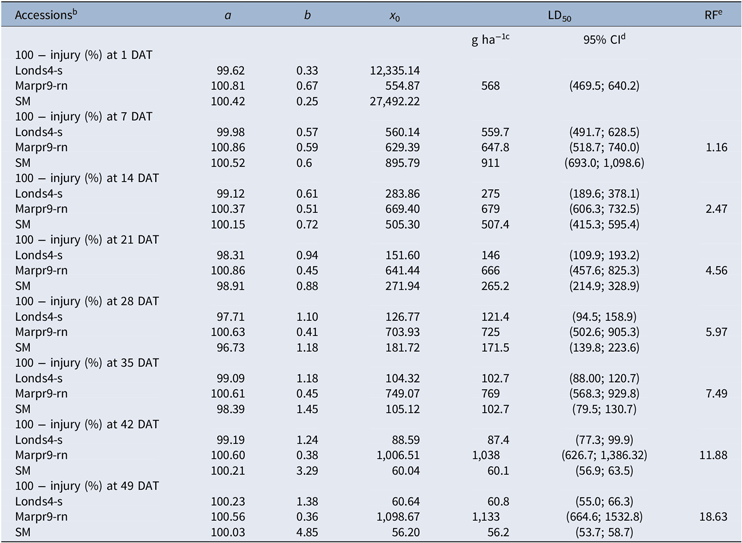
a y = a/1 + (x/x 0)b, where y is the response expressed as percentage of the untreated control, a is the asymptotic value of y at the upper limit, b is the slope of the curve around the point of inflection, x 0 is the dose required to reduce the y values by 50% (LD50) when a equals 100. P < 0.05 for all log-logistic equations.
b DAT, days after treatment.
c Concentrations required for 50% reduction of 100 − injury (%) (LD50); P < 0.05.
d Values in this column are 95% confidence intervals.
e RF: LD50 ratio between the corresponding biotype and the susceptible Londs4-s accession.
There have been no scientific reports of resistance to 2,4-D herbicide in the Conyza genus. However, in a study carried out with horseweed (Erigeron canadensis L.), tolerance differences between populations were evidenced, with an LD50 amplitude of 12.3 to 19.7 g ae ha−1 and LD90 of 42 g to 107 g ae ha−1 of 2,4-D among the most susceptible and tolerant populations, respectively (Kruger et al. Reference Kruger, Davis, Weller and Johnson2008). The LD values obtained in that study are low and possibly do not characterize herbicide resistance. In addition, the occurrence of rapid necrosis in response to 2,4-D was not observed. In 2,4-D–resistant A. tuberculatus plants, the dose required to reduce growth by 50% (GR50) was 176 g ha−1, and the resistance factor was 8-fold in relation to the susceptible accession (Figueiredo et al. Reference Figueiredo, Leibhart, Reicher, Tranel, Nissen, Westra, Bernards, Kruger, Gaines and Jugulam2018). In the same study, previous application of cytochrome P450 inhibitor reduced the GR50 of the resistant accession 7-fold, indicating that 2,4-D detoxification was likely to be the mechanism responsible for resistance. However, the occurrence of rapid necrosis has not been reported in 2,4-D–resistant A. tuberculatus accessions. The occurrence of rapid necrosis has been reported only in A. trifida in response to glyphosate, but the mechanism of resistance is unknown (Moretti et al. Reference Moretti, Van Horn, Robertson, Segobye, Weller, Young, Johnson, Douglas Sammons, Wang, Ge, d’Avignon, Gaines, Westra, Green and Jeffery2017).
Cross-Resistance to Auxinic Herbicides
Mechanisms related to defects in the general auxin response pathway are likely to cause resistance to other auxinic herbicides (LeClere et al. Reference LeClere, Wu, Westra and Sammons2018). Therefore, studies were conducted to characterize the response of this accession to several additional auxinic herbicides. At 1 d after treatment, the rapid necrosis symptoms were observed in response to 2,4-D but not the other four auxinic herbicides tested (Table 4). The symptom caused by these other auxin herbicides was consistent with the typical auxin-induced epinasty (Figure 5A). At 21 DAT, regrowth was evident only for 2,4-D treatment (Figure 5B). The plants of the Marpr9-rn accession were also susceptible to the herbicides dicamba and triclopyr, as indicated in the dose–response study, in which the RF values at 49 DAT were 1.2 and 0.8, respectively (Table 5; Supplementary Figure S2). Therefore, Marpr9-rn is resistant to 2,4-D, but cross-resistance to the other auxinic herbicides—fluroxypyr, triclopyr and picloram (pyridine carboxylic acid), dicamba (benzoic acid), and florpyrauxifen-benzyl and halauxifen-methyl (arykpicolin acid)—was not identified.
Table 4. Plant injury (%) in susceptible (Londs4-s) and resistant to 2,4-D with rapid necrosis (Marpr9-rn) accessions of Conyza sumatrensis in response to the application of five auxinic herbicides at 1, 14, and 49 d after treatment (DAT) and fresh weight at 49 DAT.

a Lowercase letters compare means between accessions in the same time (rows), and uppercase letters compare means within accessions (columns); P > 0.05 using Tukey’s test.

Figure 5. Plants of the Conyza sumatrensis Marpr9-rn accession treated with the auxinic herbicides 2,4-D, fluroxypyr, picloram, halauxifen-methyl, and florpyrauxifen benzyl ester at 1 (A) and 21 d (B) after spraying.
Table 5. Parameters of the logistic equation,a concentrations of the herbicides 2,4-D, dicamba, and triclopyr required for 50% reduction of 100 − visual injury (LD50) and shoot fresh weight (GR50) of Conyza sumatrensis, and resistance factor (RF) in the whole-plant dose–response studies.

a y = a/1 + (x/x 0)b, where y is the response expressed as percentage of the untreated control, a is the asymptotic value of y at the upper limit, b is the slope of the curve around the point of inflection, x 0 is the dose required to reduce the y values by 50% (LD50) when a equals 100. P < 0.05 for all log-logistic equations.
b DAT, days after treatment.
c Concentrations required for 50% reduction of fresh weight (GR50) or 100 − injury (%) (LD50); P < 0.05.
d Values in this column are 95% confidence intervals.
e RF: LD50 ratio between the correspondent biotype and the susceptible Londs4-s accession.
To date, resistance to 2,4-D has been reported in 21 weed species, in some cases coupled with resistance to other auxinic herbicides (Heap Reference Heap2019). Cross-resistance to 2,4-D, MCPA, and dicamba herbicides was identified in L. serriola (Burke et al. Reference Burke, Yenish, Pittmann and Gallagher2009) and oriental mustard (Sisymbrium orientale L.) (Preston et al. Reference Preston, Dolman and Boutsalis2013). A complete understanding of the mechanism of resistance to auxinic herbicides has yet to be determined for most resistant species. However, there are several reports about the process possibly involved in the resistance. One possibility is related to defects in the downstream response from auxins, such as the induction of ethylene synthesis. In S. arvensis, the resistance to picloram was associated with the activity of the acetyl-CoA synthase enzyme that is a precursor of ethylene (Hall et al. Reference Hall, Alam and Murr1993). Similarly, 20-fold lower ethylene levels were identified in a biotype of C. solstitialis resistant to picloram (Fuerst et al. Reference Fuerst, Sterling, Norman, Prather, Irzyk, Wu, Lownds and Callihan1996). Reduction of ethylene might be associated with the induction of de novo synthesis of a 19-kDa protein found in those biotypes. Reduced translocation was also reported as the mechanism of resistance to 2,4-D in S. orientale (Dang et al. Reference Dang, Malone, Boutsalis, Krishnan, Gill and Preston2018) and L. serriola (Riar et al. Reference Riar, Burke, Yenish, Bell and Gill2011). It was recently observed in plants of R. raphanistrum susceptible to 2,4-D that the application of inhibitors of auxin efflux or ABCB transporters mimicked the reduced translocation of the resistant biotype (Goggin et al. Reference Goggin, Cawthray and Powles2016).
Changes in the ability to sense the auxin may also cause resistance. Variation of the modulation of calcium transport in the cell was associated with S. arvensis resistance to 2,4-D, dicamba, and picloram (Wang et al. Reference Wang, Deshpande and Hall2001). A comparative study with tobacco (Nicotiana tabacum L.) cells with antisense ABP1 suggested that resistance to auxinic herbicides would occur due to the lower concentration of ABP (Mithila and Hall Reference Mithila and Hall2005). In kochia [Bassia scoparia (L.) A. J. Scott], cross-resistance was endowed by mutation of two base pairs (GGTs – AATR) in the gene IAA16 (LeClere et al. Reference LeClere, Wu, Westra and Sammons2018). IAA16 is a member of AUX/IAA family that inhibits the action of auxin-responsive factors in the absence of auxin and is labeled to degradation by 26S proteasome upon auxin interaction with the CFTIR1/AFB complex (Song Reference Song2014). The complete cross-resistance pattern to herbicides from the different chemical families was not evaluated in these studies. In addition, the phenotype of rapid necrosis was not reported in any of the studies cited.
Multiple-Resistance Analysis
Resistance to other herbicides caused by the same mechanism involved in rapid necrosis in response to 2,4-D was not expected, considering that cross-resistance was not found. However, taking into account that C. sumatrensis has been reported as resistant to other herbicides, resistance to several other herbicide modes of action applied at recommended doses was assessed in addition to 2,4-D. The evaluation of the herbicide plant injury effects at 14 and 49 DAT indicated that the herbicides paraquat, ammonium glufosinate, and saflufenacil were efficient for controlling the 2,4-D–resistant Marpr9-rn accession (Table 6; Figure 6). However, low herbicide efficacy was observed for glyphosate at 1,440 and 2,880 g ha−1 and chlorimuron-ethyl at 20 and 40 g ha−1, indicating that resistance to these herbicides could also occur in the 2,4-D–resistant Marpr9-rn accession. Resistance of Conyza spp. to glyphosate and ALS inhibitors is one the main problems related to weed control in several regions of the world (Byker et al. Reference Byker, Soltani, Robinson, Tardif, Lawton and Sikkema2013; Urbano et al. Reference Urbano, Borrego, Torres, Leon, Jimenez, Dinelli and Barnes2007; VanGessel Reference VanGessel2001). The occurrence of multiple resistance to glyphosate and chlorimuron-ethyl herbicides in C. sumatrensis was previously identified in the same region where Marpr9-rn was collected by Santos et al. (Reference Santos, Oliveira, Constantin, Francischini and Osipe2014). In this study, it was observed the occurrence of accessions with LD50 of 449.60 g ae ha−1 for glyphosate and 26.12 g ai ha−1 for chlorimuron-ethyl, resulting in a RF of 6.74 and 26.09, respectively. More recently, C. sumatrensis accessions with multiple resistance to the herbicides glyphosate, chlorimuron-ethyl, and paraquat have been reported (Heap Reference Heap2019). However, in the present study, the survival of plants treated with the herbicide paraquat at the doses of 400 and 800 g ai ha−1 was not evidenced.
Table 6. Plant injury (%) at 2, 14, and 49 d after treatment (DAT) and fresh weight at 49 DAT in susceptible (Londs4-s) and resistant to 2,4-D with rapid necrosis (Marpr9-rn) accessions of Conyza sumatrensis in response to the application of six herbicides with different mechanisms of action.

a Lowercase letters compare means between accessions in the same time (rows), and uppercase letters compare means within accessions (columns); P > 0.05 using Tukey’s test.

Figure 6. Plants of the Conyza sumatrensis Marpr9-rn accession treated with 1X (dose 1) (A) and 2X (dose 2) (B) of 2,4-D, paraquat, saflufenacil, ammonium glufosinate, glyphosate, and chlorimuron-ethyl at 14 d after treatment.
The high frequency of resistance to glyphosate and ALS inhibitors and the absence of rapid necrosis symptoms in the resistant accessions of Conyza identified previously (Santos et al. Reference Santos, Oliveira, Constantin, Francischini and Osipe2014; Vargas et al. Reference Vargas, Bianchi, Rizzardi, Agostinetto and Dal Magro2007) indicate that multiple resistance of the Marpr9-rn occurred independently to 2,4-D resistance. Therefore, the evolution of resistance to 2,4-D associated with rapid necrosis in this accession likely occurred in plants that previously had developed resistance to glyphosate and ALS inhibitors. The broad expansion of no-till systems was initially based on the use of glyphosate alone in burndown applications, despite recommendations for use of multiple herbicides. The problem of glyphosate resistance was not evident before the utilization of transgenic soybean resistant to glyphosate. Consequently, Conyza resistance to this herbicide evolved rapidly, and this problem was solved with ALS inhibitors, more specifically chlorimuron-ethyl. Once again, the continuous use of this herbicide in mixture with glyphosate in burndown and PRE applications, and sometimes POST, resulted in multiple resistance to both products.
The herbicide 2,4-D has been used in burndown applications in no-till for several decades. However, the use of this herbicide was always in combination with glyphosate or followed by other contact herbicides, mainly paraquat. Due to the already present resistance of Conyza spp. to glyphosate and ALS inhibitors, the selection pressure for 2,4-D increased, and these species were initially controlled by 2,4-D alone. The consequence of that was the selection of 2,4-D resistance, probably caused by mechanisms independent from those of resistance to glyphosate and ALS inhibitors. Rapid necrosis was also identified in A. trifida in response to glyphosate (Brabham et al. Reference Brabham, Gerber and Johnson2011; Moretti et al. Reference Moretti, Van Horn, Robertson, Segobye, Weller, Young, Johnson, Douglas Sammons, Wang, Ge, d’Avignon, Gaines, Westra, Green and Jeffery2017; Van Horn et al. Reference Van Horn, Moretti, Robertson, Segobye, Weller, Young, Johnson, Schulz, Green, Jeffery, Lespérance, Tardif, Sikkema, Hall and McLean2017). However, the effect of glyphosate on the Marpr9-rn accession observed in the present study was not associated with rapid necrosis (Figures 2 and 6). Therefore, this new mechanism of resistance appears to be elicited by two different herbicides, glyphosate and 2,4-D, in two different species, A. trifida and C. sumatrensis, respectively. The origin and regulation of the rapid necrosis mechanism of resistance and the similarities between these two cases of herbicide resistance remain to be discovered.
Evaluation of Presence of H2O2 in Function of Light
ROS production is essential for the development of the epinasty symptom in plants treated with 2,4-D (Pazmiño et al. Reference Pazmiño, Rodríguez-Serrano, Sanz, Romero-Puertas and Sandalio2014). In these studies, H2O2 production was identified after the occurrence of epinasty symptoms within 3 to 4 DAT of the herbicide to sensitive plants. It is possible that enzymes involved in oxidative stress relief may act on sensitive plants within the first hours after 2,4-D application. Preliminary studies with susceptible and resistant C. sumatrensis accessions indicated formation of H2O2 in the leaves of the resistant Marpr9-rn accession caused by 2,4-D at 7 HAT (Supplementary Figure S3). The herbicide paraquat caused similar symptoms in the Marpr9-rn and susceptible Londs4-s accessions, indicating that the method was effective for identification of H2O2 formation (Supplementary Figure S3). A subsequent study based on earlier evaluations indicated that H2O2 had already increased at 15 min after the application of 2,4-D in both accessions (Figure 7; Supplementary Figure S4). However, in the Marpr9-rn accession, H2O2 production increased substantially at 30 min after application and remained high until 1 DAT. For the susceptible Londs4-s accession, H2O2 remained constant until 420 min (7 h) after application (Figure 7). The presence of peroxide was equal in both accessions 1 d after the 2,4-D herbicide application. Several studies report the effect of 2,4-D on the production of H2O2 and other ROS in the plant death process (McCarthy-Suárez Reference McCarthy-Suárez2017; Pazmiño et al. Reference Pazmiño, Romero-Puertas and Sandalio2012; Rodríguez-Serrano et al. Reference Rodríguez-Serrano, Pazmiño, Sparkes, Rochetti, Hawes, Romero-Puertas and Sandalio2014; Romero-Puertas et al. Reference Romero-Puertas, Mccarthy, Gómez, Sandalio, Corpas, Del Río and Palma2004).
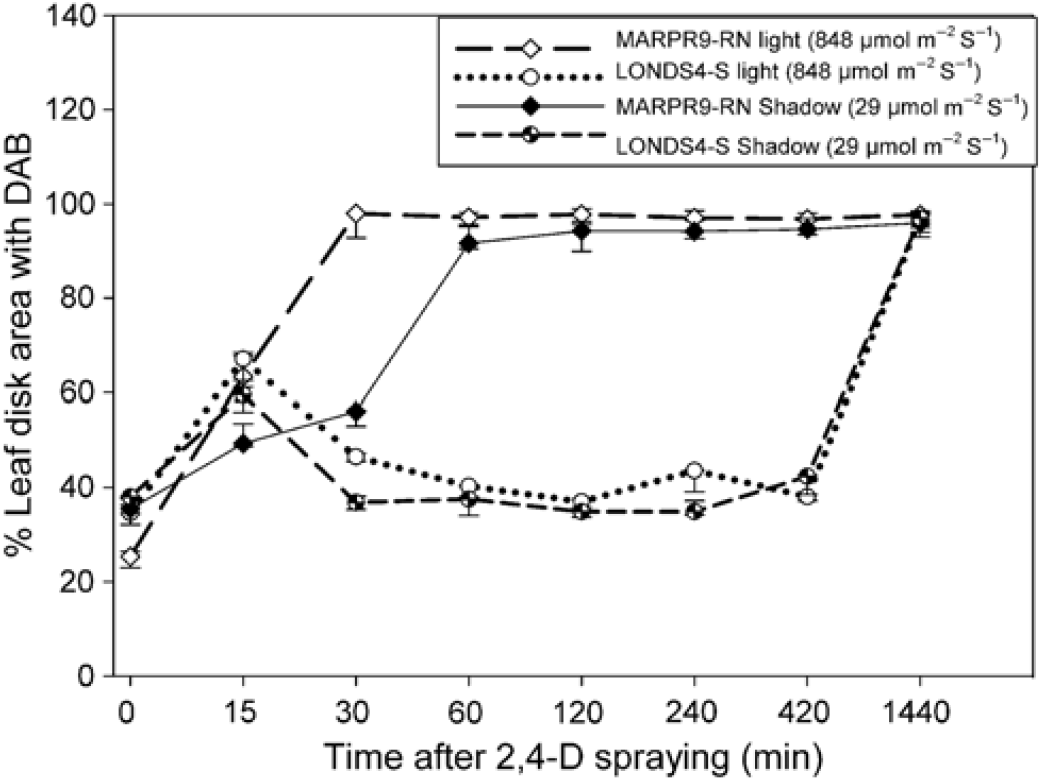
Figure 7. Percentage of leaf disk area with 3,3′-diaminobenzidine-hydrochloride (DAB) dye in Conyza sumatrensis susceptible (Londs4-s) and resistant to 2,4-D (Marpr9-rn) accessions in two light conditions. Average of three replicates ± SE.
The results obtained in the present study indicated the production of H2O2 at 15 min after application in both accessions, but only in the resistant Marpr9-rn did the production increase greatly after that (Figure 7; Supplementary Figure S4). Production of H2O2 is associated with the first appearance of the rapid necrosis visual symptoms, which occurred at 2 h after herbicide spraying, as described earlier (Figure 1). The increase of H2O2 in the susceptible Londs4-s at 1 d after 2,4-D application was associated with the beginning of the epinastic response.
Light intensity influenced the H2O2 production in the Marpr9-rn accession. In the low-light condition (29 μmol m−2 s−1), the amount of leaf area labeled by DAB was lower in relation to the high-light condition (848 μmol m−2 s−1) (Figure 7; Supplementary Figure S4). Atypical dehydration symptoms also showed late onset in the low-light condition, 4 HAT, in contrast to 1 HAT in high-light condition. A similar delay was also observed in A. trifida resistant to glyphosate (Moretti et al. Reference Moretti, Van Horn, Robertson, Segobye, Weller, Young, Johnson, Douglas Sammons, Wang, Ge, d’Avignon, Gaines, Westra, Green and Jeffery2017) and in the hypersensitivity response to plant pathogens (Roden and Ingle Reference Roden and Ingle2009). The light effect appears to modulate the velocity of the beginning of the rapid necrosis and certainly is related to the variability of the occurrence of this symptom in experimental and field conditions.
The causes of the rapid increase in H2O2 (ROS) production in herbicide hypersensitivity in weeds have not yet been elucidated (Ghanizadeh and Harrington Reference Ghanizadeh and Harrington2017). Also, it is not yet known whether the reduced herbicide translocation occurs due to the rapid foliar necrosis or if this necrosis occurs due to the absence of translocation of the herbicide. In the studies examining rapid necrosis of A. trifida in response to glyphosate, it was verified that the joint supply of the amino acids phenylalanine and tryptophan inhibited the occurrence of the rapid necrosis phenotype (Moretti et al. Reference Moretti, Van Horn, Robertson, Segobye, Weller, Young, Johnson, Douglas Sammons, Wang, Ge, d’Avignon, Gaines, Westra, Green and Jeffery2017). The biosynthesis of these amino acids is inhibited by the action of glyphosate. Based on these results, the authors concluded that the phenotype of rapid necrosis was not caused by the herbicide molecule but by the effects of the herbicide mechanism. To date, rapid necrosis in C. sumatrensis has been found to be specific for 2,4-D herbicide and does not occur in response to glyphosate, which indicates that in this species, this symptom is not possibly associated with amino acid biosynthesis as described for glyphosate effect in A. trifida.
In conclusion, the biotype Marpr9-rn is resistant to 2,4-D and the rapid necrosis symptoms begin at approximately 2 h after herbicide application with partial leaf wilt and light necrotic spots. A build-up of ROS precedes the visual occurrence of the rapid necrosis in the resistant biotype. This symptom occurs faster under light conditions, which are conditions that favor the herbicidal effects of 2,4-D. Cross-resistance to other auxinic herbicides was not identified in the Marpr9-rn biotype, but multiple resistance to ALS and EPSPS inhibitors occurs, apparently caused by independent mechanisms. This is the first report of resistance to the herbicide 2,4-D caused by rapid necrosis. This rapid response phenotype had been previously reported only for glyphosate in A. trifida (Brabham et al. Reference Brabham, Gerber and Johnson2011). Knowledge about the origin and regulation of rapid necrosis resulting in resistance to these herbicides is not available. Rapid necrosis is a new mechanism of herbicide resistance and characterizes the increasing complexity of weed control based only on herbicide strategies.
Acknowledgments
The authors thank Dow AgroSciences Industrial LTDA, especially the Integrated Field Scientist Team, for assistance in seed collection and shipment. The authors are grateful to the National Council for Scientific and Technological Development (CNPq) for a scholarship granted to ARSdQ and fellowships awarded to CAD and the sixth author. Funding was provided by CNPq and Dow AgroSciences Industrial LTDA. The authors declare that there is no conflict of interest.
Supplementary Material
To view supplementary material for this article, please visit https://doi.org/10.1017/wsc.2019.65