Introduction
Species from the genus Habranthus Herb., actually including the genus Zephyranthes Herb. (Amaryllidaceae) (García et al., Reference García, Meerow, Arroyo-Leuenberger, de Oliveira, Dutilh, Soltis and Judd2019), are native to the American continent. Within South America, they are distributed in Brazil, Uruguay, Paraguay, Chile and Argentina, the latter being the country with the highest concentration (Hurrell et al., Reference Hurrell, Bazzano, Delucci and Hurell2005). They are mostly perennial herbs, geophytes, with small bulbs, fibrous roots and attractive colourful flowers. They develop mainly in natural environments with little water availability, although their flowering occurs after the rainy season. Among the species described in Argentina, some show great interest in their qualities, both ornamental (Hurrell et al., Reference Hurrell, Bazzano, Delucci and Hurell2005; Rosselló, et al., Reference Rosselló, Marinangeli, Rodrigo and Curvetto2006; Echeverria and Alonso, Reference Echeverría and Alonso2010; Fernández et al., Reference Fernández, Marinangeli, Curvetto and Facciuto2013) and pharmacological (Hurrell et al., Reference Hurrell, Bazzano, Delucci and Hurell2005; Cavallaro et al., Reference Cavallaro, Alza, Murray and Murray2014). Particularly, Zephyranthes tubispatha (L'Hér.) Herb. (formerly Habranthus tubispathus, ‘copper lily’) is a small, erect, perennial, with a high aesthetic ornamental value for sustainable landscaping. Like other bulbous species, it can be multiplied agamically through bulbs or scales, or after sexual reproduction, through the germination of their seeds. In addition to being usually simpler and cheaper, this last process is essential for the establishment of individuals in a given environment and allows the possibility of conserving and selecting for variability within populations. Although progress has been made in understanding the requirements for flowering and germination of some species within this genus (e.g. Echeverría and Alonso, Reference Echeverría and Alonso2010; Santa Cruz et al., Reference Santa Cruz, Tapia, Romero and Quiroga2011; Fernández et al., Reference Fernández, Marinangeli, Curvetto and Facciuto2013; Wesley da Silva et al., Reference Wesley da Silva, Guerra Barbosa, Barboza da Silva, Silva Guirra, da Silva Gama, Moreira de Oliveira and França Dantas2014; Afroz et al., Reference Afroz, Rahman and Hassan2018), the factors that regulate germination have not been exhaustively analyzed.
It is widely known that temperature is one of the main abiotic regulators of seed germination. According to the thermal time model, at temperatures above the optimal range, germination decreases due to different processes that can occur simultaneously (e.g. Bewley et al., Reference Bewley, Bradford, Hilhorst and Nonogaki2013; Batlla and Benech-Arnold, Reference Batlla and Benech-Arnold2014). In particular, the exposure of seeds to high temperatures (HTs) may result in specific physiological responses called thermodormancy or thermoinhibition. While the former is characterized by prolonged inhibition of germination after exposing the seeds to optimal conditions (one type of secondary dormancy acquired at supraoptimal temperatures), the second is understood as the inhibition of germination of hydrated seeds induced by exposure to HTs, which is quickly alleviated when the seeds are transferred to suitable temperatures (Kendall and Penfield, Reference Kendall and Penfield2012; Huo et al., Reference Huo, Wei and Bradford2016; Derakhshan et al., Reference Derakhshan, Bakhshandeh, Siadat, Moradi-Telavat and Andarzian2018; Geshnizjani et al., Reference Geshnizjani, Ghaderi-Far, Willems, Hilhorst and Ligterink2018). This type of response has been reported in celery (Bouzo et al., Reference Bouzo, Favaro and Pilatti2007), parsley (da Silva et al., Reference da Silva, Baldini, Ferreira, Nakagawa and da Silva2017), tomato (Geshnizjani et al., Reference Geshnizjani, Ghaderi-Far, Willems, Hilhorst and Ligterink2018) and rapeseed (Derakhshan et al., Reference Derakhshan, Bakhshandeh, Siadat, Moradi-Telavat and Andarzian2018), and it has been deeply studied in lettuce (e.g. Argyris et al., Reference Argyris, Dahal, Hayashi, Still and Bradford2008; Deng and Song, Reference Deng and Song2012; Yoong et al., Reference Yoong, O'Brien, Truco, Huo, Sideman, Hayes and Bradford2016) and Arabidopsis thaliana (Tamura et al., Reference Tamura, Yoshida, Tanaka, Sasaki, Bando, Toh and Kawakami2006; Toh et al., Reference Toh, Kamiya, Kawakami, Nambara, McCourt and Tsuchiya2012; Huo et al., Reference Huo, Wei and Bradford2016; Yan and Chen, Reference Yan and Chen2020). Recent studies on the germination requirements of Z. tubispatha seeds indicate that the germination percentage markedly decreases at temperatures above 25°C, probably due to thermoinhibition (Manfreda et al., Reference Manfreda, Acosta and Alcaráz2019), although the underlying physiological mechanisms are unknown.
Many reports have shown that thermoinhibition is under the control of phytohormones, among which abscisic acid (ABA), gibberellins (GAs) and ethylene play a key role (Nambara et al., Reference Nambara, Okamoto, Tatematsu, Yano, Seo and Kamiya2010; Linkies and Leubner-Metzger, Reference Linkies and Leubner-Metzger2012; Huo and Bradford, Reference Huo, Bradford and Anderson2015). Particularly, the increase in endogenous ABA levels together with the inhibition of GA biosynthesis would be among the main causes of germination inhibition at HT, and GA supply has been shown to release thermoinibition in some species by stimulating the expression of genes involved in ABA degradation (e.g. Gonai et al., Reference Gonai, Kawahara, Tougou, Satoh, Hashiba, Hirai, Kawaide, Kamiya and Yoshioka2004; Toh et al., Reference Toh, Imamura, Watanabe, Nakabayashi, Okamoto, Jikumaru, Hanada, Aso, Ishiyama, Tamura, Iuchi, Kobayashi, Yamaguchi, Kamiya, Nambara and Kawakami2008; Leymarie et al., Reference Leymarie, Benech-Arnold R, Farrant J and Corbineau2009; Vishal and Kumar, Reference Vishal and Kumar2018). Even though the antagonism between ABA and GA is considered to play a key role in controlling seed germination, the mechanism of their interaction is still not fully understood, and many other factors apart from these phytohormones are implicated in the regulation of the thermal response during this process (e.g. Saini et al., Reference Saini, Consolacion, Bassi and Spencer1989; Hossain et al., Reference Hossain, Itoh, Yoshimura, Tokuda, Oku, Cohen and Yamasaki2010; Toh et al., Reference Toh, Kamiya, Kawakami, Nambara, McCourt and Tsuchiya2012; Ye et al., Reference Ye, Zhu, Liu, Zhang, Li, Liu, Shi, Jia and Zhang2012; Huo and Bradford, Reference Huo, Bradford and Anderson2015; Ma et al., Reference Ma, Guan, Li, Pan, Wang, Liu, Ma, Zhu, Hu, Ruan, Chen and Zhang2019; Wei et al., Reference Wei, Yang, Huo, Ge, Liu, Luo, Hu, Huang and Long2020). Particularly, ethylene production as well as genes implicated in ethylene biosynthesis are down-regulated in seeds exposed to thermoinhibitory conditions (e.g. Gallardo et al., Reference Gallardo, Delgado Mdel, Sánchez-Calle and Matilla1991; Argyris et al., Reference Argyris, Dahal, Hayashi, Still and Bradford2008), and the supply of ethylene-releasing compounds was shown to promote germination at supraoptimal temperatures in different species (e.g. Keys et al., Reference Keys, Orrin, Kumamoto and Lyon1975; Rao et al., Reference Rao, Sankhla and Khan1975; Gallardo et al., Reference Gallardo, Delgado Mdel, Sánchez-Calle and Matilla1991; Corbineau et al., Reference Corbineau, Xia, Bailly and El-Maarouf-Bouteau2014).
HTs are usually associated with heat stress. Like other types of stress, this causes an increase in the production of free radicals and reactive oxygen species (ROS), with consequent damage to macromolecules and cell integrity (Candan and Tarhan, Reference Candan and Tarhan2003; Demidchik, Reference Demidchik2015). Lipid peroxidation and protein carbonylation are among the first physiological indicators of oxidative stress. To maintain cell redox homeostasis, plants have several enzymatic and non-enzymatic mechanisms, some of which are specifically stimulated in response to altered levels of ROS (Demidchik, Reference Demidchik2015). In particular, among the former, the activities of antioxidant enzymes such as superoxide dismutase (SOD, EC 1.15.1.1), ascorbate peroxidase (APX, EC 1.11.1.11), glutathione peroxidase (GPX, EC 1.11.1.9), glutathione S-transferase (GST, EC 2.5.1.18), guaiacol peroxidase (GPOX, EC 1.11.1.7) and catalase (CAT, EC 1.11.1.6) have been shown to play an essential role in the prevention of oxidative damage under numerous types of stress (reviewed in Blokhina et al., Reference Blokhina, Virolainen-Arne and Fagerstedt2003; Orabi and Abou-Hussein, Reference Orabi and Abou-Hussein2019). Although ROS accumulation under stress conditions is considered to cause cell damage and alterations in the growth of seedlings, it is widely accepted that ROS action is necessary for the breakdown of dormancy and seed germination (Oracz et al., Reference Oracz, Bouteau, Farrant, Cooper, Belghazi, Job, Job, Corbineau and Bailly2007; El-Maarouf-Bouteau and Bailly, Reference El-Maarouf-Bouteau and Bailly2008; Leymarie et al., Reference Leymarie, Vitkauskaité, Hoang, Gendreau, Chazoule, Meimoun and Bailly2012; El-Maarouf-Bouteau et al., Reference El-Maarouf-Bouteau, Meimoun, Job, Job and Bailly2013; Pedrosa Gomes and Souza García, Reference Pedrosa Gomes and Souza Garcia2013; Bailly, Reference Bailly2019). In this sense, ${\rm O}_2^{{\cdot}{-}} $ generation by plasma membrane NADPH oxidases was shown to play a key role in the regulation of different processes required for seed germination, including ABA and/or GA metabolism and dormancy release, in different species such as A. thaliana (Leymarie et al., Reference Leymarie, Vitkauskaité, Hoang, Gendreau, Chazoule, Meimoun and Bailly2012; Li et al., Reference Li, Zhi and Fu2019), barley (Ishibashi et al., Reference Ishibashi, Kasa, Sakamoto, Aoki, Kai, Yuasa, Hanada, Yamaguchi and Iwaya-Inoue2015) and Vigna radiata (Singh et al., Reference Singh, Chaudhuri and Kar2014). Similarly, H2O2 supply can promote germination in seeds exposed to different stressors, including HT (e.g. Çavusoglu and Kabar, Reference Çavusoglu and Kabar2010; Gomes et al., Reference Gomes, Bicalho and Garcia2022).
While progress has been made on the possible links between ROS generation, antioxidant metabolism activity and hormonal regulation pathways during dormancy release and germination under different environmental conditions (El-Maarouf-Bouteau and Bailly, Reference El-Maarouf-Bouteau and Bailly2008; Ye et al., Reference Ye, Zhu, Liu, Zhang, Li, Liu, Shi, Jia and Zhang2012; Bailly, Reference Bailly2019), the information available on the role of the antioxidant metabolism in the thermoinhibitory process is relatively scarce. Furthermore, most of the research work on this topic has been carried out in dicotyledonous species, so it is interesting to analyze whether similar mechanisms occur in monocotyledonous plants such as the species studied here.
The main purposes of the present work are to characterize the germination responses of Z. tubispatha seeds to different thermal conditions and to analyze the role of key components of the antioxidant metabolism and phytohormones (ABA, GA and ethylene) in their control, particularly during and after exposure of the seeds to supraoptimal temperatures.
Materials and methods
Seed collection and germination assays
Z. tubispatha seeds were obtained from natural populations located in the district of Azul in Buenos Aires, Argentina. Given that flowering in these populations takes place from December to mid-April, fruits collected in a similar period (December 2018 and December 2021) were assayed to minimize putative effects due to the thermal conditions of the maternal environment during seed formation. Soon after harvesting, the capsules were distributed on wooden paper to complete their drying, and once opened, they were threshed and the seeds were allowed to air dry for a week at room temperature. Seeds were then stored at 10°C into sealed tubes placed inside two sealed polyethylene bags.
Culture chambers set at 7, 10, 14, 20, 28 and 33°C (±1.5°C) were used to characterize the germination response at different temperatures under darkness. The germination tests were performed in plastic Petri dishes containing a layer of filter paper over four layers of towel paper, moistened with 4 ml of distilled water. Fifty seeds were sown on each Petri dish without previous disinfection. To preserve humidity and help minimize temperature fluctuations, each Petri dish was placed inside a transparent plastic box on a layer of foam rubber impregnated with water. The boxes were, in turn, placed inside trays containing a water layer, which were wrapped with transparent polyethylene bags prior to the application of the temperature treatments.
To study the effect of a HT pretreatment on the germination at an optimum temperature, seeds were hydrated at 33 ± 2°C as described above for different periods (0, 3, 5, 7, 9, 15, 25, 47 and 60 d) and then transferred to 20°C to monitor germination in the dark (treatments HTi + 20C, where i = pretreatment period). Four replicates of 50 seeds each were used per condition assayed. Seeds were considered germinated when at least 2 mm length of the non-haustorial segment of the embryo had emerged (Tillich, Reference Tillich, Rudall, Cribb, Cutler and Humphries1995; see also Supplementary Fig. S1). The number of germinated seeds was counted daily until the beginning of germination (considered as the time point where 8–10% of the seeds had germinated), and then every 12–30 h (depending on the germination response) until no new germination events occurred for at least 60 h. At the end of each assay, the total number of germinated (maximum germination) and non-germinated seeds were recorded. Non-germinated seeds were classified in the following seminal states, according to the general criteria of ISTA (2006): empty (E), dead (D) and firm under finger pressure (F), as an estimator of the proportion of live seeds whose germination might be inhibited. Maximum germination (Gm) and each seminal state were expressed as percentages of the total number of seeds minus empty seeds.
To test whether the seed hydration profile differed between the control (20°C) and supraoptimal (33°C) temperatures, four replicates of 40 mg of seeds each were placed in germinating Petri dishes as described above and weighed at different times after imbibition until the control began to germinate.
Study of the effect of temperature on different components of the oxidative metabolism
The evaluation of oxidative damage and changes in the antioxidant metabolism was carried out by measuring lipid peroxidation, total antioxidant capacity and the activity of antioxidant enzymes (CAT, APX, GPOX, GST and SOD), in seeds exposed to the following experimental conditions: hydration at 20°C without HT pretreatment (control treatment, 20C), hydration at HT (33°C) for 3, 5 and 25 d (treatments HT3, HT5 and HT25, respectively) and hydration at HT for 3 and 25 d and then transferred to 20°C (treatments HT3 + 20C and HT25 + 20C, respectively). Dry seeds were also tested in order to determine the initial level of each trait. Samplings were carried out in quadruplicate at the end of each hydration period at 33°C, and at the beginning of germination at 20°C, in order to compare seed populations with a similar germination stage. The material was stored at −75°C until further processing.
Effect of pharmacological treatments on the germination responses
A pharmacological approach was adopted to investigate whether changes in the endogenous levels of key regulators of seed germination such as the phytohormones such as ABA, GA and ethylene, as well as ascorbic acid (AA) or hydrogen peroxide (H2O2), were involved in the control of the thermoinhibition process. When indicated, seed germination (three plates with 30–35 seeds each) was performed at HT (33°C), in the darkness, in the presence of different concentrations of the ABA biosynthesis inhibitor fluridone, ethephon (acid 2-chlorethylphosphate), which is a source of ethylene in plant tissues (Kolářová et al., Reference Kolářová, Bezděčková and Procházková2010 and references therein), GA4+7, GA3 (supplied either alone or in combination with fluridone or ethephon), AA or H2O2. When indicated, the GA biosynthesis inhibitor paclobutrazol (Hedden and Graebe, Reference Hedden and Graebe1985) was included in ethephon treatments (see Table 2 for a description of all the conditions tested). In order to test whether seed sensitivity to selected treatments varied with the length of the HT incubation period reagents was supplied either from the beginning, or at 9 or 25 d after starting imbibition at HT (treatments HT0, HT9 and HT25, respectively). Germination was then monitored over 2 weeks unless it plateaued within a shorter time. When necessary, treatments were renewed in order to minimize changes in solute concentration, as well as to reduce the risk of contamination. Germination at either 20 or 33°C was also monitored in the absence of externally added metabolites as controls. When indicated, the ethylene biosynthesis inhibitor CoCl2 (Schaller and Binder, Reference Schaller and Binder2017) was used in some of the germination trials (see Results). At the end of the assays, non-germinated seeds were examined, and the percentage of firm, empty and dead seeds was estimated as previously described.
Biochemical analyses
Lipid peroxidation was estimated by measuring malondialdehyde (MDA) content in the seeds according to Vavilin et al. (Reference Vavilin, Ducruet, Matorin, Venediktov and Rubin1998), with some modifications. Briefly, 0.05 g of fresh weight was homogenized with 0.70 ml of 20% trichloroacetic acid (TCA) and centrifuged 20 min at 17,500 × g. The reaction media contained 500 μl of supernatant, 500 μl of a mixture of 20% TCA + 0.5% thiobarbituric acid and 50 μl of 2% (w/v) butylatehydroxytoluene (BHT) in absolute ethanol. After heating for 30 min at 95°C, samples were cooled to room temperature and centrifuged 5 min at maximum speed to obtain a clear supernatant. MDA equivalents per ml extract were determined after reading the samples at 440, 532 and 600 nm in a Shimadzu UV 1800 spectrophotometer according to Hodges et al. (Reference Hodges, DeLong, Forney and Prange1999).
Total antioxidant capacity in aqueous extracts (0.05 g FW/0.40 ml) was estimated through the formation of a phosphomolybdenum complex as described in Prieto et al. (Reference Prieto, Pineda and Aguilar1999). Ascorbic acid was used as standard.
For the determination of CAT-, APX-, GPOX-, GST- and SOD-specific activity, whole seeds (about 0.1 g of fresh weight) were extracted in a mortar, on ice, with 0.70 ml extraction buffer consisting of 50 mM Tris–HCl buffer (pH 7.6) plus 1.0 mM ethylenediaminetetraaceticacid (EDTA), 1.0 mM ascorbic acid, 2.0 mM MgSO4, 1.0 mM phenylmethanesulfonyl fluoride (PMSF) and 0.5% Triton X-100, in the presence of 2.0 mg of polyvinylpolypyrrolidone (PVPP). The extracts were centrifuged at 17,500 × g (two cycles of 30 and 20 min, respectively) in a refrigerated centrifuge, kept in ice and immediately used for the enzyme assays. The activity of the above-mentioned enzymes was determined following the procedures described in Causin et al. (Reference Causin, Bordón and Burrieza2020), using the following reaction mixtures (in all cases the final volume was 1 ml): 80 μl extract + 50 μl of 5 mM ascorbic acid + 20 μl of 0.1 M H2O2 + reaction buffer (for APX); 35 μl of enzyme extract + 120 μl of guaiacol solution + 25 μl of 0.1 M H2O2 + reaction buffer (for GPOX); 25 μl of enzyme extract + 25 μl of 0.7 M H2O2 + reaction buffer (for CAT); 60 μl of extract + 20 μl of CDNB solution + 50 μl of GSH solution + reaction buffer (for GST). For the measurement of SOD activity in 96-well clear microplates, aliquots of 6–12 μl of enzyme extract were added to the incubation medium, which contained 270 μl of ${\rm O}_2^{{\cdot}{-}} $-generating mixture plus reaction buffer up to a final volume of 0.3 ml.
Soluble protein concentration in the extracts was determined according to Bradford (Reference Bradford1976), using bovine serum albumin as a standard.
To measure the concentration of ABA, freeze-dried seeds of selected treatments (three to four replicates of 280 ± 20 mg, per condition tested) were extracted using a modification of the protocol of Durgbanshi et al. (Reference Durgbanshi, Arbona, Pozo, Miersch, Sancho and Gómez-Cádenas2005). Seeds were homogenized in an Ultraturrax T25 basic homogenizer (IKA, Staufen, Germany) with 5 ml of deionized water. Fifty nanograms of [2H6]ABA (OlChemIm s.r.o, Olomouc, Czech Republic) were added as an internal standard. Extracts were transferred to 50-ml tubes and after centrifugation (15 min, 1500 × g), the supernatant was collected, adjusted to pH 2.8 with 15% (v/v) acetic acid and extracted twice with an equal volume of diethyl ether. The aqueous phase was discarded and the organic fraction was evaporated by vacuum. Dried extracts were dissolved in 1 ml of methanol. Samples were filtered through a syringe filter tip on a vacuum manifold at a flow rate of less than 1 ml min-1, and the eluate was evaporated at 35°C under vacuum in a SpeedVac SC110 (Savant Instruments, Inc., New York, NY, USA). Identification and quantification of ABA was performed with a quadrupole tandem mass spectrometer (MS/MS) (Quattro pt Ultima, Micromass, Manchester, UK) fitted with an electrospray ion (ESI−) source in a multiple reaction monitoring mode (MRM). The analysis included precursor ions and their transitions (m/z): ABA (m/z 263/153) and D6-ABA (m/z 269/159). The cone energy used was 35 V and the spectrometry software for data analysis was MassLynx version 4.1.
Histochemical detection of superoxide anion and hydrogen peroxide generation
To detect superoxide anion (${\rm O}_2^{{\cdot}{-}} $) production, embryos from 12 to 15 seeds randomly sampled from selected treatments, were carefully separated with the aid of small tweezers and immediately stained for 12 min with a solution of 0.1% (w/v) nitroblue tetrazolium (NBT) in 50 mM potassium phosphate buffer (pH 7.5) in the dark (Jabs et al., Reference Jabs, Dietrich and Dangl1996). To visualize the presence of H2O2, embryos were stained for 3.5 h with a solution of 0.1% 3,3-diaminobenzidine (DAB) in 50 mM potassium phosphate (pH 5.5) (modified from Thordal-Christensen et al., Reference Thordal-Christensen, Zhang, Wei and Collinge1997). In both cases, after staining, the embryos were rinsed in distilled water to stop the reaction and kept in 70% ethanol at 4°C until being analyzed. Embryos were observed under a Zeiss Stemi 2000-C stereomicroscope and photographed with a Sony DSC-S70 camera, and adapted to the equipment.
Data analysis and statistical tests
Cumulative germination per sampling unit was characterized through non-linear regression by fitting the following equation: % G = A*(1 − 1/(1 + (t/B)^k)) (Geshnizjani et al., Reference Geshnizjani, Ghaderi-Far, Willems, Hilhorst and Ligterink2018; Manfreda et al., Reference Manfreda, Alcaraz and Scaramuzzino2020). Coefficient A is an asymptotic value equivalent to the Gm percent (i.e. % Gm); B equals the time required to reach 50% of Gm (i.e. t 50) and k is a dimensionless shape coefficient around a given B (i.e. a measure of the slope of the curve at t 50). The germination rate at each temperature was estimated as the inverse of B value. In a few cases, when the estimated value for coefficient A exceeded 100%, it was fixed to 100 and the remaining parameters were recalculated.
Non-parametric Kruskal–Wallis ANOVA and multiple comparisons of mean ranks were performed to test for significant differences in germination traits and in each of the parameters estimated after equation fitting. One-way ANOVAs were performed to test for significant differences in MDA concentration, total antioxidant capacity, enzyme activities and ABA concentration. Tukey's HSD post hoc tests were used to identify homogeneous groups. In those cases where ANOVA assumptions could not be met, non-parametric Kruskal–Wallis ANOVA was performed as previously described. Unless otherwise stated, treatment differences were considered significant at P ≤ 0.05. All statistical analyses were performed with Infostat 2017 version (Di Rienzo et al., Reference Di Rienzo, Casanoves, Balzarini, Gonzalez, Tablada and Robledo2017).
Results
Germination responses to temperature treatments
Gm was close to or greater than 90% within the temperature range from 7 to 20°C (Supplementary Table S1), and preliminary tests at 20°C showed that seeds harvested both in 2018 and 2021 had a similar germinative behaviour (data not shown). Average seed mortality did not exceed 1% within this temperature range, so that non-germinated seeds were mostly firm. At elevated temperatures (28 and 33°C), the % Gm markedly decreased, although the percentages of non-viable seeds did not significantly differ from the other treatments (Supplementary Table S1). Instead, at these supraoptimal temperatures, the low % Gm values coincided with the presence of high percentages of firm seeds, suggesting the existence of an inhibitory effect on the germination process. This phenomenon was not associated with an alteration of the seed hydration profile (Supplementary Fig. S2). When those seeds were transferred to 20°C, about 60–90% germinated within the first week, supporting the notion that thermoinhibition rather than secondary dormancy was the most likely underlying process (see also below).
The temperature effect was not only evident in the final seminal stages, but also in the dynamic of the germination response and the time to germination onset. For example, while at extreme temperatures (7 and 28°C) germination started after about 420 h from sowing, the time was reduced to 70–80 h when the tests were performed between 14 and 20°C (Fig. 1A), suggesting that the temperature range for optimal germination in this species is close to these values.

Fig. 1. Representative data of cumulative germination of Z. tubispatha seeds exposed (A) at different temperatures or (B) at 20°C after 3-, 5-, 7-, 9-, 25- or 60-d incubation at HT (33°C).
Given the low percentages of Gm recorded at 28 and 33°C, equation fitting was performed on cumulative germination data within the thermal range of 7–20°C. As shown in Table 1, the estimated values for coefficient A were close to or higher than 90%, which is in agreement with the % Gm data mentioned above. The time required to reach 50% germination (coefficient B) markedly increased at temperatures below 14°C, indicating that they can be considered suboptimal for seed germination in this species. On the contrary, k values did not follow a consistent pattern among the temperatures analyzed (Table 1).
Table 1. Mean values (and standard deviation) for coefficients A, B, k in Z. tubispatha seeds exposed to the indicated temperatures. Statistically homogeneous groups within each row are indicated with the same letter

The thermoinhibitory effect of HT on the germination of Z. tubispatha seeds was confirmed when seeds exposed to 33°C for different periods were able to germinate successfully after being transferred to 20°C (e.g. Fig. 1B). Interestingly, no significant HT pretreatment effect was observed for Gm, which ranged between 94 and 99% in all cases. This is in agreement with the values of coefficient A obtained after equation fitting (Supplementary Table S2). On the contrary, significant differences for coefficient B were observed depending on the length of the HT pretreatment. In fact, B values were higher than the control in seeds incubated during 3–9 d at HT, while they significantly decreased when the pretreatments were extended for 25 d or more (Fig. 2). Taken together, these data suggest that Z. tubispatha seeds developed two types of responses: one after a short-term exposure and other after a long-term exposure to supraoptimal temperatures, with the first leading to a delayed, while the second to accelerated germination once the seeds are exposed to 20°C. Regarding the effect of HT treatments on coefficient k, while a great intra- and inter-treatment variability was observed for pretreatment periods lower than 15 d, k values tended to increase when the incubation at HT exceeded that period (data not shown).

Fig. 2. Mean values and standard deviation (bars) for coefficient B (an estimator of T 50), calculated after equation fitting to cumulative germination at 20°C, in Z. tubispatha seeds incubated for different periods at HT (33°C). Statistically homogeneous groups are indicated with the same letter.
Effect of temperature treatments on the antioxidant metabolism of whole seeds and ROS generation in the embryo
The total antioxidant capacity of Z. tubispatha seeds was about threefold higher prior to imbibition in the germination plates, irrespective of the temperature treatment (Fig. 3A). Even though most of the lower values for this trait were recorded in seeds sampled at the beginning of germination, no significant differences were observed due to the HT pretreatments (Fig. 3A).

Fig. 3. Mean values and standard deviation (bars) of (A) total antioxidant capacity and (B) MDA concentration in Z. tubispatha seeds, before (dry seeds) or after exposure to the following temperature treatments: 20C, germination at 20°C (optimum temperature control); HT3, 5 or 25, seeds incubated for 3, 5 or 25 d at HT (33°C); HT3 + 20C or HT25 + 20C, seeds incubated during 3 or 25 d at HT and then transferred to 20°C (data recorded at the start of germination). Statistically homogeneous groups are indicated with the same letter.
As for total antioxidant capacity, the content of MDA significantly decreased after seed soaking (Fig. 3B). Despite the degree of lipid peroxidation remained at low levels in all the conditions tested, when HT-incubated seeds were transferred to 20°C and sampled at the beginning of germination (i.e. treatments HT3 + 20C and HT25 + 20C), the MDA concentration was significantly higher in seeds that had been pretreated for 3 d at 33°C compared to those pretreated for 25 d, which also showed lower MDA levels than the 20C control (Fig. 3B).
The specific activity of both peroxidases (APX and GPOX) was very low in dry seeds and increased about ten times when measured either at the start of germination at 20°C, or immediately after a short-term exposure (3 d) to 33°C (Fig. 4A, B), which was close to the average time required to initiate germination at an optimal temperature. When the HT pretreatment was extended up to 5 d, a significant down-regulation of the activity of both peroxidases was observed. Nevertheless, this negative effect was less pronounced (for APX) or nil (for GPOX) after 25 d of HT pretreatment (HT25). Interestingly, while only a partial recovery of APX activity was observed at the start of germination when the seeds were transferred to an optimum temperature (Fig. 4A, treatments HT3 + 20C and HT25 + 20C), GPOX activity showed a consistent up-regulation, irrespective of the duration of the HT pretreatment (Fig. 4B, treatments HT3 + 20C and HT25 + 20C).

Fig. 4. Mean values and standard deviation (bars) of (A) APX- and (B) GPOX-specific activity in Z. tubispatha seeds, before (dry seeds) or after the exposure to the following temperature treatments: 20C, start of germination at 20°C (optimum temperature control); HT3, 5 or 25, seeds incubated for 3, 5 or 25 d at HT (33°C); HT3 + 20C or HT25 + 20C, seeds incubated during 3 or 25 d at HT and then transferred to 20°C (data recorded at the start of germination). Statistically homogeneous groups are indicated with the same letter.
Contrary to peroxidases, dry seeds had relatively high constitutive CAT and GST activities, although the responses of either enzyme to the temperature treatments markedly differed (Fig. 5A, B). As for peroxidases, the specific activity of CAT was stimulated at the onset of germination in control seeds, or immediately after 3 d of pretreatment at HT, but then decreased as the incubation period at 33°C increased (Fig. 5A). When the enzyme activity was measured after the transfer to 20°C, a different behaviour was found depending on the length of the HT pretreatment: while it partially recovered in the seeds that had been exposed for a long period (25 d), it was consistently down-regulated in those incubated for the shortest period (Fig. 5A). As opposed to CAT, GST-specific activity decreased after seed hydration in most of the conditions tested, with the exception of the HT25 and the HT25 + 20C treatments, where a significant up-regulation was observed (Fig. 5B).

Fig. 5. Mean values and standard deviation (bars) of (A) CAT, (B) GST and (C) SOD-specific activity in Z. tubispatha seeds, before (dry seeds) or after the exposure to the following temperature treatments: 20C, start of germination at 20°C (optimum temperature control); HT3, 5 or 25, seeds incubated for 3, 5 or 25 d at HT (33°C); HT3 + 20C or HT25 + 20C, seeds incubated during 3 or 25 d at HT and then transferred to 20°C (data recorded at the start of germination). Statistically homogeneous groups are indicated with the same letter.
Finally, SOD-specific activity in Z. tubispatha seeds increased both with the soaking time and with the period of incubation at 33°C, without being significantly altered after the transfer to 20°C (Fig. 5C).
NBT staining of isolated embryos showed an intense colouring of the radicle poles when the seeds were assayed at the start of germination at 20°C, either in the control as well as in seeds previously exposed to HT (Fig. 6). However, when NBT staining was performed immediately after the incubation at HT, a marked inhibition of colour formation occurred as the incubation period increased (Fig. 6). Interestingly, the haustorial end of the embryo was also stained, although changes due to the temperature conditions were comparatively minor. Similar trends were found for H2O2 formation, although both the rate of H2O2 generation and the magnitude of the thermal effects were less marked than those observed for superoxide anions (Supplementary Fig. S3).

Fig. 6. NBT staining of representative embryos isolated from seeds of the following temperature treatments: 20C, start of germination at 20°C (optimum temperature control); HT3 or 25, seeds incubated for 3 or 25 d at HT (33°C); HT3 + 20C or HT25 + 20C, seeds incubated during 3 or 25 d at HT and then transferred to 20°C (seeds were collected at the start of germination). Note the intense colouring of the radicle pole (black arrowheads) in embryos sampled at the beginning of germination. c, cotyledon; cs, cotyledonary sheath; hp, haustorial pole; pl, primary leaf; rp, radicle pole. Bar = 1 mm.
Effect of pharmacological treatments on germination responses
A marked difference in the responses to some of the pharmacological treatments was observed depending on the length of incubation at HT. Sensitivity to fluridone increased along with HT exposure time in a dose-dependent manner (Fig. 7), although concentrations higher than 60 μM inhibited germination probably due to toxic effects (Table 2). While a high germination percentage was attained with 20 μM fluridone when supplied at the start of the HT incubation period (Table 2), the stimulatory effect was only evident after about 185 h (Fig. 7). In contrast, when fluridone was given at 25 d HT exposure, it was able to restore germination at a rate similar or even higher than the 20C control (Fig. 7, treatments HT25 + 20F and HT25 + 60F, respectively). The response to fluridone was not significantly modified by the simultaneous addition of either 40 μM or 1 mM GA3 (data not shown). Ethephon also had a stimulatory effect of germination at HT. Among the concentrations tested, 75 mg/l was the one that showed the most consistent results (higher concentrations increased the contamination of plates and seed mortality). As for fluridone, the sensitivity to ethephon tended to increase together with the extent of the HT pretreatment, although in this case only two conditions (HT0 and HT9) could be assayed (Fig. 8A). The addition of 40 μM GA3 to the ethephon solution increased the percentage of germinated seeds when supplied after 9 d (but not at 0 d) HT pretreatment (Fig. 8A). On the other hand, the effect of ethephon was strongly inhibited in the presence of increasing concentrations of paclobutrazol, suggesting the need for gibberellin synthesis to properly exert its action (Table 2). The addition of 40 μM GA4+7 or different concentrations of GA3 alone had almost nil effects on germination at HT (Table 2), although about 27% of the HT25-pretreated seeds germinated in the presence of 1 mM GA3 during the period assayed (data not shown).

Fig. 7. Cumulative germination of Z. tubispatha seeds supplied with 20 or 60 μM fluridone (F) at 0, 9 or 25 d from sowing at HT (33°C). Germination at optimum temperature (20°C) was included as control. Germination at HT in the presence of water alone was null irrespective of the time period assayed. Data are means ± SD.

Fig. 8. (A) Cumulative germination of Z. tubispatha seeds supplied with 75 mg/l etephon (ET) alone, or in combination with 40 μM GA3 (GA), at 0 or 9 d from sowing at HT (33°C). Germination at optimum temperature (20°C) was included as control. Germination at HT in the presence of water alone was null irrespective of the time period assayed. (B) Cumulative germination of Z. tubispatha seeds at 20°C, in the absence (control) or in the presence of 10 mM CoCl2, supplied alone (Co2+) or plus 75 mg/l etephon (Co2+ + ET). Data are means ± SD.
Table 2. Mean values (and standard deviation) of Gm percent in Z. tubispatha seeds supplied with the indicated concentrations of fluridone (F), etephon (ET), GA3, paclobutrazol (Pbz), H2O2 or ascorbic acid (AA) at 0 d from sowing at HT (33°C)

To further evaluate whether ethylene was required for proper germination at optimum temperature, seeds were placed in germination plates containing either water alone (control) or 10 mM CoCl2 (lower concentrations had only minor effects on the germination rate in our experimental model) (data not shown). After 24 h imbibition, half of the seeds exposed to Co2+ ions were left in the same plates and the rest were transferred to plates containing a solution of 10 mM CoCl2 plus 75 mg/l ethephon. In all cases, germination was monitored at 20°C in the dark. As shown in Fig. 8B, the germination rate was significantly down-regulated in the presence of Co2+ when compared to the control, and this negative effect was reversed when ethephon was supplied together with CoCl2. Nevertheless, it is worth mentioning that when the incubation period in CoCl2 extended beyond 120 h, germination was markedly inhibited irrespective of the presence or absence of ethephon, probably reflecting toxic side effects exerted by prolonged exposure to the Co2+ ion (e.g. Burhan et al., Reference Burhan, Shaukat and Tahira2001).
The effect of 2 mM H2O2 or 3 mM AA was almost nil when supplied either at the beginning or after 9 d incubation at HT (Fig. 9). Nevertheless, both treatments exerted a marked release of thermoinhibition on seeds pretreated for 25 d at HT, which reached more than 70% germination at the end of the experimental period.
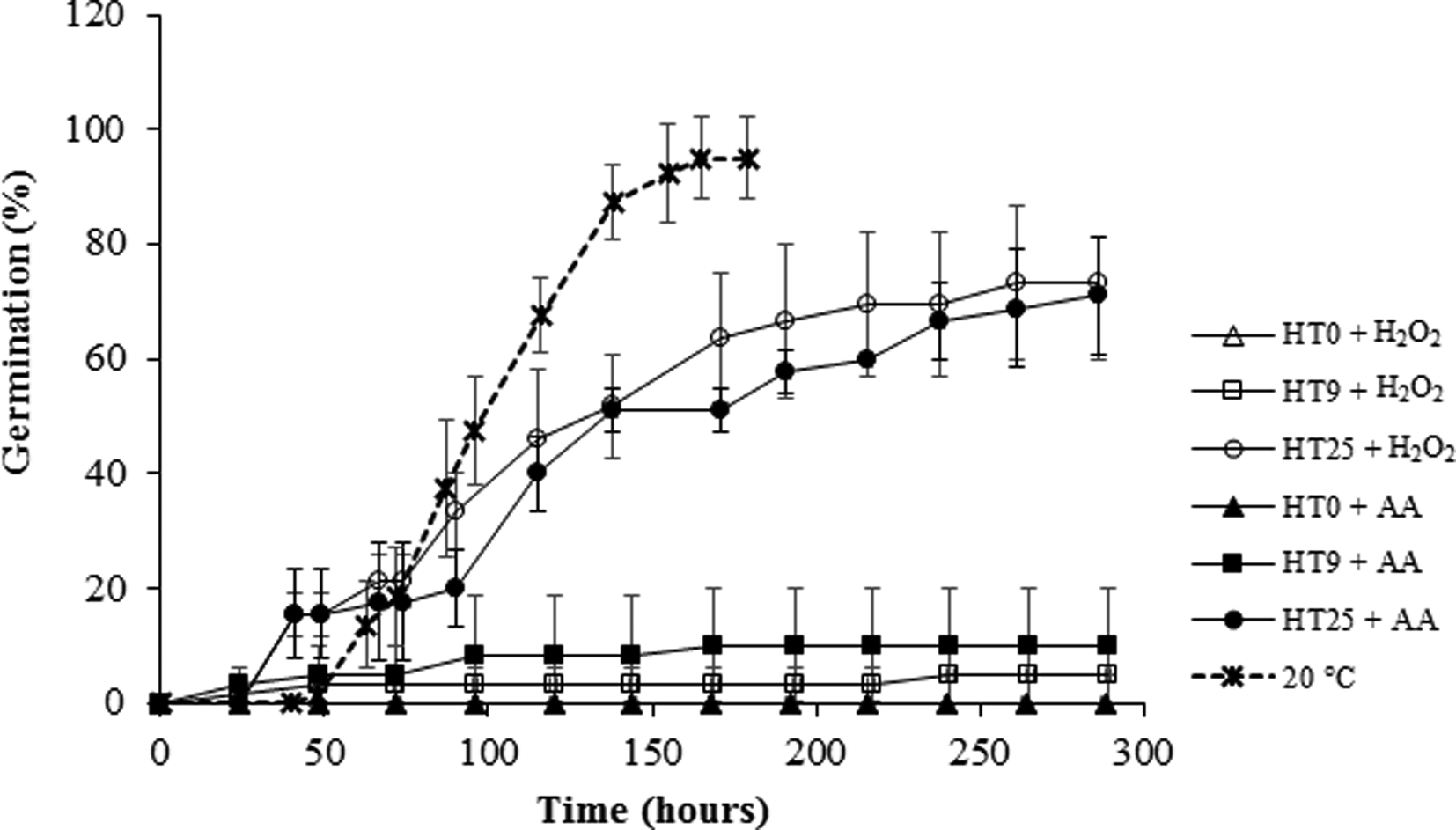
Fig. 9. Cumulative germination of Z. tubispatha seeds supplied with 2 mM H2O2 or 3 mM ascorbic acid (AA) at 0, 9 or 25 d from sowing at HT (33°C). Germination at optimum temperature (20°C) was included as control. Germination at HT in the presence of water alone was null irrespective of the time period assayed. Data are means ± SD.
Finally, the analysis of ABA content in whole seeds showed that ABA levels were highest in non-soaked seeds and steadily decreased as the HT incubation period increased (Fig. 10). A reduction in ABA content was observed at the start of germination at 20°C in most cases; however, seeds from the control treatment maintained significantly higher levels of this phytohormone than those pretreated for either 5 or 25 d at HT (Fig. 10).

Fig. 10. Mean values and standard deviation (bars) of ABA concentration in Z. tubispatha seeds, before (dry seeds) or after the exposure to the following temperature treatments: 20C, start of germination at 20°C (optimum temperature control); HT5 or 25, seeds incubated for 5 or 25 d at HT (33°C); HT5 + 20C or HT25 + 20C, seeds incubated during 5 or 25 d at HT and then transferred to 20°C (data recorded at the start of germination). Statistically homogeneous groups are indicated with the same letter.
Discussion
Germination responses of Z. tubispatha seeds to temperature
Z. tubispatha is a species native of South America, which shows great ornamental interest. Knowing its thermal requirements for germination is essential for its conservation and reproduction. Our results show that this species has the potential to attain its Gm percentage in a temperature range between 7 and 20°C, without evidencing significant changes in seed mortality. In this sense, preliminary tests showed that seed viability was not affected when seeds were stored for at least 10 months at ambient compared to low (10°C) temperature (data not shown). This is a desirable trait in terms of commercial production and is in line with data reported for the same species by Maza et al. (Reference Maza, Uría and Roitman2004).
Fitting the three-parameter non-linear function to cumulative germination data satisfactorily described the germination dynamics of this native species, as already shown for other native species like Baccharis dracunculifolia subsp. tandilensis (Manfreda et al., Reference Manfreda, Alcaraz and Scaramuzzino2020) and Rhodophiala bifida, a closely related species to Z. tubispatha (Acosta et al., Reference Acosta, Alcaraz, Scaramuzzino and Manfreda2021). Despite the fact that total germination percent at 7°C did not significantly differ from the 20°C (control) treatment, an important delay in both germination onset and rate (coefficient B) was observed, leading us to the conclusion that the minimum temperature for optimum germination in Z. tubispatha seeds was clearly above 7°C.
A marked reduction of both germination rate and % Gm was observed at temperatures around 28°C or higher. The recovery of germination after transferring the seeds to an optimum temperature (20°C) supports the notion that thermoinhibition rather than thermodormancy was the underlying phenomenon, as reported for several cultivated species (e.g. Bouzo et al., Reference Bouzo, Favaro and Pilatti2007; Deng and Song, Reference Deng and Song2012; Yoong et al., Reference Yoong, O'Brien, Truco, Huo, Sideman, Hayes and Bradford2016; da Silva et al., Reference da Silva, Baldini, Ferreira, Nakagawa and da Silva2017; Derakhshan et al., Reference Derakhshan, Bakhshandeh, Siadat, Moradi-Telavat and Andarzian2018; Geshnizjani et al., Reference Geshnizjani, Ghaderi-Far, Willems, Hilhorst and Ligterink2018). To our knowledge, this is the first report demonstrating the existence of thermoinhibition in this species. In an experimental trial with Habranthus gracilifolius and Rodophiala bifida (recently reclassified into the genus Zephyrantes), Echeverría and Alonso (Reference Echeverría and Alonso2010) showed that both species attained germination values higher than 92% when incubated either at 20°C (constant) or at alternating temperatures of 10/20°C, but that germination was significantly delayed (particularly in H. gracilifolius) at alternating temperatures of 20/30°C. In view of our results, it is possible that the use of the latter regime partially masked the inhibitory effect of HT (30°C) on the total germination of H. gracilifolius. It would be interesting to carry out tests under similar experimental conditions and with a greater number of species to determine whether the phenomenon of thermoinhibition is common within this genus.
A differential response was observed for germination dynamics at an optimum temperature depending on the duration of HT exposure (Figs 1B and 2), which was reflected in the behaviour of several measured physiological traits, as well as in changes in sensitivity to germination promoters due to the HT treatments will be further discussed. This is an interesting aspect that points to the importance of comparing the germinative response after both short and long periods of exposure to thermoinhibitory conditions in species frequently used as model systems, something scarcely reported in the available literature (Hills and van Staden, Reference Hills and van Staden2003).
Changes in ROS homeostasis and phytohormone metabolism are involved in the control of germination responses by temperature cues in Z. tubispatha
To understand the physiological processes underlying the observed responses, we first studied the changes in different components of the antioxidant metabolism in whole seeds, as well as ROS generation in embryonic tissues, given their importance in regulating not only seed viability but also dormancy breakdown and germination (reviewed in El-Maarouf-Bouteau and Bailly, Reference El-Maarouf-Bouteau and Bailly2008; El-Maarouf-Bouteau et al., Reference El-Maarouf-Bouteau, Meimoun, Job, Job and Bailly2013; Pedrosa Gomes and Souza Garcia, Reference Pedrosa Gomes and Souza Garcia2013; Kokila et al., Reference Kokila, Bhaskaran, Sathish and Lakshmi Prasanna2014; Bailly, Reference Bailly2019).
In agreement with the low percentages of seed mortality, the HT treatments did not appear to cause major increases in oxidative stress symptoms. The lower level of lipid peroxidation detected in soaked – as compared to dry – seeds has been frequently reported and may be associated with both a dilution effect due to tissue imbibition, as well as a down-regulation of the oxidative conditions generated during the desiccation phase of seed maturation, once the metabolic (including the antioxidant) activity is resumed (Bailly, Reference Bailly2019; see also Figs 4 and 5). In a similar way, the slightly (but statistically significant) lower MDA content found in Z. tubispatha seeds from the 25d HT treatment is consistent with the observed up-regulation of several of the antioxidant enzymes tested after long-term (compared to short-term) exposure to supraoptimal temperature.
In several orthodox seeds, the re-activation of both APX activity and ascorbic acid (AA) biosynthesis has been shown to play an important role in seed germinability (De Gara et al., Reference De Gara, Paciolla, Liso, Stefani and Arrigoni1991, Reference De Gara, Paciolla, De Tullio, Motto and Arrigoni2000; De Tullio and Arrigoni, Reference De Tullio and Arrigoni2003). In rice kernels, an adequate supply of both ROS and AA is required to promote many biochemical and physiological processes of seed germination (Ye et al., Reference Ye, Zhu, Liu, Zhang, Li, Liu, Shi, Jia and Zhang2012). Particularly, in that monocotyledonous species, the suppression of germination by exogenous ABA involved the inhibition of AA biosynthesis and ROS generation via NADPH oxidases, which in turn caused a down-regulation of APX and CAT activity, in addition to other factors. Both enzymes were shown to play an important role in the tight control of endogenous H2O2 pools (reviewed in Sofo et al., Reference Sofo, Scopa, Nuzzaci and Vitti2015; Anjum et al., Reference Anjum, Sharma, Gill, Hasanuzzaman, Khan, Kachhap, Mohamed, Thangavel, Devi, Vasudhevan, Sofo, Khan, Misra, Lukatkin, Singh, Pereira and Tuteja2016), which, in turn, may serve as a secondary messenger in phytohormone signalling, as well as in key physiological processes during seed germination (Çavusoglu and Kabar, Reference Çavusoglu and Kabar2010; Bahin et al., Reference Bahin, Bailly, Sotta, Kranner, Corbineau and Leymarie2011; Bailly, Reference Bailly2019). Interestingly, the activity of APX and CAT was also lowered in Z. tubispatha seeds exposed to either 5 or 25 d at HT when compared to the 20C control, which is also in agreement with data reported on the effect of HT on the activity of antioxidant enzymes in rice grains (Liu et al., Reference Liu, Hasanuzzaman, Wen, Zhang, Peng, Sun and Zhao2019). Furthermore, AA as well as H2O2 were able to partially rescue Z. tubispatha seed germination under HT when supplied after a long-term (but not short-term) incubation period, suggesting that the maintenance of adequate levels of these metabolites is necessary (although not sufficient) for the control of germination responses to thermal cues in this species.
The fact that ROS homeostasis was altered at supraoptimal temperatures was also confirmed by the down-regulation of both ${\rm O}_2^{{\cdot}{-}} $ and H2O2 generation in the embryo (but particularly at the root pole) as the period of incubation at HT increased. Several studies indicate that localized ROS production is required for cell expansion during the morphogenesis of organs such as roots and leaves. An active generation of superoxide anions in the apex of germinating embryos has been reported in different species such as maize (Liszkay et al., Reference Liszkay, van der Zalm and Schopfer2004), cucumber (Renew et al., Reference Renew, Heyno, Schopfer and Liszkay2005), A. thaliana (Renew et al., Reference Renew, Heyno, Schopfer and Liszkay2005; Carol and Dolan, Reference Carol and Dolan2006; Dunand et al., Reference Dunand, Crèvecoeur and Penel2007), salix (Causin et al., Reference Causin, Roqueiro, Petrillo, Láinez, Pena, Marchetti, Gallego and Maldonado2012) and barley (Ishibashi et al., Reference Ishibashi, Kasa, Sakamoto, Aoki, Kai, Yuasa, Hanada, Yamaguchi and Iwaya-Inoue2015); and its suppression was sufficient to inhibit germination in some of these or other ones (e.g. Ishibashi et al., Reference Ishibashi, Tawaratsumida, Zheng, Yuasa and Iwaya-Inoue2010, Reference Ishibashi, Kasa, Sakamoto, Aoki, Kai, Yuasa, Hanada, Yamaguchi and Iwaya-Inoue2015; Causin et al., Reference Causin, Roqueiro, Petrillo, Láinez, Pena, Marchetti, Gallego and Maldonado2012; Liu et al., Reference Liu, Zhou and Xing2012). Being a very short-lived ROS, it is believed that the main mechanism of action is through the oxidative modification of molecules that, in turn, would contribute to the relief of dormancy and germination of the seed (Oracz et al., Reference Oracz, Bouteau, Farrant, Cooper, Belghazi, Job, Job, Corbineau and Bailly2007; Bailly, Reference Bailly2019). Moreover, ROS generated by NADPH oxidases were shown to stimulate GA biosynthesis and ABA catabolism in germinating barley seeds, supporting the view that a tight interaction between the metabolism of ROS and phytohormones controls germination under different environmental conditions (Ishibashi et al., Reference Ishibashi, Aoki, Kasa, Sakamoto, Kai, Tomokiyo, Watabe, Yuasa and Iwaya-Inoue2017).
The induction of either thermodormancy or thermoinhibition has often been associated with the maintenance of high endogenous ABA levels (e.g. Gonai et al., Reference Gonai, Kawahara, Tougou, Satoh, Hashiba, Hirai, Kawaide, Kamiya and Yoshioka2004; Argyris et al., Reference Argyris, Dahal, Hayashi, Still and Bradford2008; Leymarie et al., Reference Leymarie, Robayo-Romero, Gendreau, Benech-Arnold and Corbineau2008, Reference Leymarie, Benech-Arnold R, Farrant J and Corbineau2009; Liu et al., Reference Liu, Hasanuzzaman, Wen, Zhang, Peng, Sun and Zhao2019). This would not appear to be the case in Z. tubispatha, since ABA contents in whole seeds declined to lower levels than those in the 20C control as the HT incubation period increased. This may partly explain why the sensitivity to fluridone and most of the germination promoters assayed was highest in seeds exposed during a long-term rather than a short-term period to HT. However, the fact that seeds incubated during 25 d at HT failed to germinate despite having much lower endogenous ABA levels than the 20C control suggests that either increases in ABA sensitivity rather than content, and/or the maintenance of an ABA/germination promoter ratio above a critical level, would be essential for germination inhibition at HT. In celery seeds, thermoinhibition has been associated with the accumulation of a germination inhibitor that did not appear to be ABA since ABA levels in thermoinhibited seeds were lower than those in untreated seeds and did not increase with the duration of HT treatment (Thomas et al., Reference Thomas, Dearman and Biddington1986). In the recent work, Xia et al. (Reference Xia, Ponnaiah, Thanikathansubramanian, Corbineau, Bailly, Nambara, Meimoun and El-Maarouf-Bouteau2019) investigated hormone involvement in temperature-induced germination as compared to that caused by after-ripening in sunflower seeds. Dormant (D) seeds cannot germinate at 10°C but fully germinate at 20°C, while after-ripened seeds become non-dormant (ND), that is, able to germinate at 10°C. Hormone quantification showed that after-ripening is mediated by a decline in both ABA content and sensitivity, while ABA content was increased in D seeds treated either at 10 or 20°C. Both findings support the notion that changes in ABA levels are not a prerequisite for the release (or maintenance) of temperature-induced germination inhibition and highlight the importance of examining the role of other factors in the regulation of germination by temperature.
Several lines of evidence support the idea that in mature seeds of most Angiosperms, ABA, GA and ethylene interact to tightly regulate germination responses to environmental cues (reviewed in Linkies and Leubner-Metzger, Reference Linkies and Leubner-Metzger2012). The comparatively minor effect exerted by GA when supplied either alone or with fluridone suggests that its endogenous levels would not be the main factor limiting germination at HT in our experimental conditions. However, the promotion of germination exerted by ethephon, particularly as the HT-incubation period increased, indicates that HT-induced down-regulation of endogenous ethylene levels may contribute to the maintenance of thermoinibition in Z. thubispatha seeds. HT was shown to decrease ethylene production in different species (Corbineau et al., Reference Corbineau, Xia, Bailly and El-Maarouf-Bouteau2014), and several works demonstrate that stimulation of ethylene biosynthesis or the exogenous supply of ethylene-releasing compounds also helped to overcome germination suppression by HT (e.g. Saini et al., Reference Saini, Consolacion, Bassi and Spencer1989; Corbineau et al., Reference Corbineau, Xia, Bailly and El-Maarouf-Bouteau2014). The requirement of ethylene for proper germination of Z. tubispatha seeds even at optimum temperature is also supported by the decrement of germination rate observed when ethylene biosynthesis was inhibited through Co2+ supply. Among the mechanisms of action proposed, it is worth mentioning that ethylene was shown to counteract ABA synthesis and sensitivity and to stimulate both GA biosynthesis and signalling, as well as ROS production in the embryo (Corbineau et al., Reference Corbineau, Xia, Bailly and El-Maarouf-Bouteau2014). Interestingly, the effect of ethephon was enhanced or suppressed when GA3 or paclobutrazol, respectively, was supplied together with the ethylene-releasing compound during seed exposure to HT. This confirms that both phytohormones interact, and suggests that the action of ethylene would favour either GA perception and/or signalling rather than GA production.
In summary, the present work demonstrates that thermoinhibition is a state of high dynamism, in which the level and/or perception of phytohormones and the sensitivity to multiple germination promoters change over time. Particularly, the efficacy of the antioxidant metabolism in preventing oxidative damage after long-term exposure to supraoptimal temperatures can be highlighted, as well as the relevance of localized alterations in ROS homeostasis and in ABA metabolism to maintain thermoinhibition in Z. tubispatha seeds. Unlike some model species studied, the presence of ethephon was much more effective in suppressing thermoinhibition than GA supply alone, suggesting that temperature-induced changes in the relative balance and/or sensitivity between ethylene and ABA across time play an important role in the control of thermoinhibition as well as the response to germination-promoting compounds. New experimental approaches are required to test this hypothesis and deepen the understanding of the mechanisms involved in the regulation of germination by thermal cues in Z. tubispatha.
Supplementary material
To view supplementary material for this article, please visit: https://doi.org/10.1017/S0960258522000228.
Acknowledgements
The authors thank Mg. Rosa Lourdes Scaramuzzino for her valuable collaboration in the identification, characterization and collection of the plant material used, and Dr. Leandro Martínez Tosar for helping with the language edition of the manuscript draft.
Financial support
The present work was supported by the Universidad de Buenos Aires (Project UBACyT Mod. I 20020170100331BA) and the Universidad Nacional del Centro de la Provincia de Buenos Aires.
Conflicts of interest
The author(s) declare none.