INTRODUCTION
Utilizing archives of past environmental information with any accuracy depends upon placing any inferred information into a chronological framework. In the absence of independent chronological controls (e.g. tephras; Lowe et al. Reference Lowe, Shane, Alloway and Newnham2008, Reference Lowe, Blaauw, Hogg and Newnham2013; Vandergoes et al. Reference Vandergoes, Hogg, Lowe, Newnham, Denton, Southon, Barrell, Wilson, McGlone, Allan, Almond, Petchey, Dabell, Dieffenbacher-Krall and Blaauw2013), radiocarbon (14C) dating continues to be the preeminent method used to date organic-rich sedimentary environments. Whereas bulk 14C age determinations for any specific depth in a sediment produce an average age based upon the various organic components (Wust et al. Reference Wust, Jacobsen, Van Der Gaast and Smith2008), it is tempting but erroneous to imagine that the increased analytical precision and the analysis of smaller sample mass via accelerator mass spectrometry (AMS) 14C dating also contributes to an increased accuracy in estimating the true age of any sediment sample.
In Australia there is a narrow range of geomorphic and climatic settings capable of supporting the accumulation of organic sediments (Whinam and Hope Reference Whinam and Hope2005). This means that terrestrial organic sediments are relatively rare, but they often occur in locations that can be sensitive to subtle climate shifts and so they are ideal for targeted palaeoenvironmental research. In eastern Australia organic sediments are often contained in topogenous mires (Whinam et al. Reference Whinam, Hope, Clarkson, Buxton, Alspach and Adam2003; Hope and Whinam Reference Hope and Whinam2005; Hope et al. Reference Hope, Nanson and Jones2011), and these environments can experience higher energy surface flows with corresponding erosivity (Nanson Reference Nanson2009) and regular fire events (e.g. Black et al. Reference Black, Mooney and Attenbrow2008) compared to most sites used for palaeoenvironmental research elsewhere. This means that although these mires contain a variety of organic materials potentially available for 14C dating, their utility for constructing an accurate chronology needs careful consideration.
It is well known that some organic components of sediments, including wood and charcoal, may have inbuilt ages as they might come from old wood or persist in a landscape for centuries or longer and hence return older dates than the unit from which it is sampled (Blong and Gillespie Reference Blong and Gillespie1978; Kershaw et al. Reference Kershaw, Mckenzie, Porch, Roberts, Brown, Heijnis and Orr2007). Such inbuilt age characteristics are not unique to eastern Australia, for example Oswald et al. (Reference Oswald, Anderson, Brown, Brubaker, Hu, Lozhkin, Tinner and Kaltenrieder2005) described wood and charcoal from Arctic and Boreal depositional environments with consistently older ages than mosses and short-lived plant macrofossils sampled from the same depth. In New Zealand Howarth et al. (Reference Howarth, Fitzsimons, Jacobsen, Vandergoes and Norris2013) found that pollen and organic fractions gave radiocarbon ages 350–750 years older than dates obtained with a variety of other methods, including tephra identification. They suggested that such chronological imprecision might be particularly relevant to larger catchments and where higher erosive energy may have the capacity to mobilize old organic material.
Only a few comprehensive Australian studies have been undertaken to investigate different radiocarbon age determinations of various organic materials and these studies have tended to focus on marine reservoir effects (e.g. Hogg and Higham Reference Hogg and Higham1998; Petchey et al. Reference Petchey, Ulm, David, McNiven, Asmussen, Tomkins, Richards, Rowe, Leavesley, Mandui and Stanisic2012) or arid environments (e.g. Bowler et al. Reference Bowler, Qi, Kezoa, Head and Baoyin1986; Gillespie et al. Reference Gillespie, Magee, Luly, Dlugokencky, Sparks and Wallace1991). Kershaw et al. (Reference Kershaw, Mckenzie, Porch, Roberts, Brown, Heijnis and Orr2007) compared dates from plant macrofossils and charcoal from two depths of Caledonia Fen in the Victorian highlands of southeast Australia but both returned ages over 40,000 calibrated years before present (cal yr BP) and so the results were at the very extreme range of 14C dating. Nonetheless, they found that samples from the same depth returned ages 1000–2000 years apart, with the relative ages of each fraction being inconsistent. Dodson and Zhou (Reference Dodson and Zhou2000) also compared the ages of charcoal and pollen fractions across five paired depth intervals within a larger project that also investigated other dating materials in a shallow lake in southwestern Australia. They found that their pollen concentrates returned younger ages for two depths and older ages for three other depths, both relative to charcoal.
Here we determine the 14C ages of three organic components isolated from the same depths across two peat-forming environments located in eastern Australia. There are relatively few opportunities to apply independent dating methods to Holocene environments in these landscapes and so a refined sampling method for 14C analysis has the potential to increase the accuracy of any derived chronology in future palaeoenvironmental research.
TECHNIQUES USED
Two sites in the Blue Mountains area of New South Wales (Figure 1) were sampled using a Russian D-section corer (Jowsey Reference Jowsey1966). Queens Swamp (also known as Long Swamp, located at 33°41'40.77''S, 150°26'38.36''E, elevation: 665 m) is a Temperate Highland Peat Swamp on Sandstone (THPSS). It is abundant in sedges and shrubs, is approximately 1 km long and between 50 and 100 m wide with a catchment of 65 ha. A 3.77 m core was taken from the lower section, 250 m upstream from a nick-point that forms the downstream terminus of the swamp (Figure 1). The second site, Goochs Crater (150°16'1''E, 33°27'17.4''S, elevation: 960 m) was cored to a depth of 9.85 m. This site is atypical of the THPSS classification assigned to it because it only has a small catchment of 2.2 ha of which half is the swamp surface itself, covered mostly by Sphagnum moss and rushes while being mostly devoid of shrubs except for the perimeter transitional zone. While the swamp surface vegetation differs between sites, the surrounding eucalypt forest/woodland is similar.

Figure 1 Location of the sampling sites in the Blue Mountains area, west of Sydney, Australia. The lower panels show Goochs Crater (left) and Queens Swamp (right) with the coring locations (X), the extent of the mires (black lines) and the water catchment (blue lines).
Contiguous 1 cm loss-on-ignition (LOI) analysis of both cores was used to identify sections with high organic content, which were selected for detailed 14C analyses. Here we report a total of 17 AMS 14C dates resulting from macroscopic charcoal, short-lived plant macrofossils and pollen-rich palynological residues isolated from 4 depths at Goochs Crater and two depths at Queens Swamp. The well-humified sediment of Goochs Crater required 2-cm depth intervals in most cases to obtain enough of the target fraction, whereas the fractions from the Queens Swamp core were all isolated from 1 cm increments. The materials and depths are described in Table 1.
Table 1 The materials used, the 14C dates and the calibrated age ranges for the sediment fractions analyzed from two eastern Australian mires. The conventional radiocarbon data are reported with 1-sigma error and the calibrated radiocarbon data are reported with 2-sigma error in calibrated years Before Present (cal yr BP).

Plant macrofossil subsamples were taken from the center of the core to avoid the possible contamination of fibrous surface material along the exterior of the corer (de Vleeschouwer et al. Reference de Vleeschouwer, Chambers and Swindles2010). Plant macrofossils that could be positively identified as short-lived were selected for dating by dispersing the samples in demineralized water and careful selection under magnification. Selecting short-lived components of the vegetation enhanced the likelihood that they had only persisted in the environment for a few years before being incorporated into the sediment sequence. In most cases these plant macrofossil samples were a mix of fern fronds, twigs and leaves, however the sample from core G3 at 275–277 cm was uncharred Eleocharis sp. seeds and the sample from 400–422 cm in the same core was a Myrtaceae operculum.
Charcoal subsamples were isolated from the same depth by immersing sediment subsamples in 4% sodium hypochlorite (bleach) overnight to remove extraneous organic material. We chose larger charcoal fragments on the basis of the assumption that they are less likely to persist through time and hence the bleached material was wet-sieved (using tap water) at 250 µm and isolated macro-charcoal fragments were selected by hand under magnification.
Pollen was concentrated using standard techniques (Faegri and Iverson Reference Faegri and Iversen1975): an alkali treatment (10% NaOH boiled on a hotplate for 10 min) was used to remove organic acids, the material was then sieved using a 130 μm mesh, followed by acetolysis (repeated washes in glacial acetic acid, a 9:1 solution of acetic anhydride (minimum 99.7% w/w) to concentrated sulfuric acid (95–98 % w/w), repeated washes in glacial acetic acid) and then repeated (n=10) washes in reverse osmosis water was used to minimize any residual old C present in the reagents used (Brown et al. Reference Brown, Nelson, Mathewes, Vogel and Southon1989).
Isolated fractions (short-lived plant macrofossils, macro-charcoal and pollen concentrates) were sent for measurement at the ANTARES AMS facility at the Australian Nuclear Science and Technology Organisation (Fink et al. Reference Fink, Hotchkis, Hua, Jacobsen, Smith, Zoppi, Child, Mifsud, van der Gaast, Williams and Williams2004). As a precaution, given that the pollen and charcoal preparations used an alkaline treatment (NaOH for pollen and hypochlorite solution for charcoal), which had the potential to absorb carbon dioxide from the atmosphere, all these samples were washed with dilute HCl and then rinsed repeatedly with de-ionized water. The plant macrofossil samples underwent acid-alkaline-acid (AAA) treatment with washes in 2M HCl, 0.5%NaOH and 2M HCl.
Radiocarbon dates were calibrated in OxCal 4.3 (Bronk Ramsey Reference Bronk Ramsey2009a) using the SHCal13 calibration curve (Hogg et al. Reference Hogg, Hua, Blackwell, Niu, Buck, Guilderson, Heaton, Palmer, Reimer, Reimer, Turney and Zimmerman2013). We also constructed age-depth relationships using P_sequence deposition models (Bronk Ramsey Reference Bronk Ramsey2008) for each fraction. These models were based on 1000 iterations, a k parameter of 20 meaning we expect events to affect the deposition in ~20 years (Bronk Ramsey Reference Bronk Ramsey2009b) and surface (depth 160 cm and zero) and year of sampling (2013, 2014, for Goochs Crater and Queens Swamp, respectively, –63 and –64 yr BP) as the uppermost chronological control point. Our cores from Goochs Crater included a substantial surficial root mat and hence we set the modern surface at the base of these roots (i.e. at 160 cm): this (versus using depth zero) did not affect our depth-age model or the results reported herein. We used the mid-point of the P_sequence depth-age models to estimate the age of a fraction at any depth.
RESULTS
Table 1 shows the dating results for the various organic fractions across the two sites. Samples from Goochs Crater returned ages between 3545 and 12,920 14C years Before Present (yr BP), at ~276 cm and ~702 cm respectively (Table 1). The Goochs Crater dates on core G3 were consistent with preliminary bulk 14C dates obtained from other cores at the site suggesting a relatively consistent accumulation rate, of ~5 cm per century, across the central portion of Goochs Crater (Martin Reference Martin2017). The Queens Swamp samples returned late Holocene ages, with sample ages between about 775 and 3570 14C yr BP. The sediment in the section of Queens Swamp sampled for this work was accumulating at rates of 14 cm per century above 116 cm and 5 cm per century between 116 and 268 cm depth. Only two samples from the same depth (Goochs Crater 420–422 cm, short-lived plant macrofossil and charcoal, OZR838 and OZR837) had overlapping 14C dates.
Figure 2 presents plots of the calibrated 14C ages for each of the sediment fractions analyzed and Figure 3 shows the depth-age data and model for both sites using the calibrated ages in Table 1. Calibration of the radiocarbon ages indicates that the analyzed Goochs Crater sediments extend back to 15,710 cal yr BP and Queens Swamp covers about 3900 cal yr BP. Calibrating the radiocarbon ages from both sites generally resulted in normally distributed probability curves, with the exception of OZS813 and OZS817, which showed young-skewed tails. Figures 2 and 3 also reveal that while the calibrated dates from the same depth often had some degree of overlap at the 95% confidence interval, the dates based on pollen concentrates for Goochs G3 420–422 cm (OZR839), 702–703 cm (OZR372) and for Queens Swamp QS4 116–119 cm (OZT157) did not overlap with the dates resulting from the other organic fractions from the same depths. The Bayesian P_sequence mid-points in the age-depth models also further increased the separation between the three different fractions (Table 1). The difference in (the mid-point of the P_sequence modeled) age of the youngest versus the oldest dated fraction generally increased with depth and was ~500 years in the early Holocene and ~2400 years for the oldest sample.

Figure 2 Plots of calibrated 14C ages for the sediment fractions analyzed from the two sites in eastern Australia.

Figure 3 Age-depth relationships for (a) Goochs Crater and (b) Queens Swamp, constructed using P_sequence deposition models (Bronk Ramsey Reference Bronk Ramsey2008) in OxCal 4.3 using the SHCAL13 calibration curve (Hogg et al. Reference Hogg, Hua, Blackwell, Niu, Buck, Guilderson, Heaton, Palmer, Reimer, Reimer, Turney and Zimmerman2013) and a K-parameter of 20 to approximate the gradual nature of organic sediment accumulation. In these figures plant macro-fossil samples are red, charcoal samples are blue and green represents pollen-rich concentrates.
The ages of the organic fractions were, in most cases, in a consistent chronological order, with short-lived plant macrofossils the youngest, the macro-charcoal giving intermediate ages and the pollen-rich concentrates producing the oldest dated fraction. The only group of samples that did not follow this generalization was from 702 cm at Goochs Crater, which were the oldest, being late Pleistocene in age. For that group of samples, the macro-charcoal sample was the youngest, the short-lived plant macrofossils in-between and the pollen-rich extract the oldest.
DISCUSSION AND CONCLUSIONS
We found that the three organic fractions: short-lived plant macrofossils, macro-charcoal and pollen-rich residues, sampled from the same depths in two sediment profiles returned different 14C ages and that this difference increased with age. These differences were almost universally consistent with short-lived plant macrofossils being the youngest organic component, the macro-charcoal fraction of an intermediate age and pollen-rich residues older than the other samples (Figure 2; Table 2). As we have no independent control we cannot ascertain which organic fraction results in the most accurate estimate of the true age of the deposition of the sediments, however, in these mire environments the most likely source of younger carbon is from root penetration from above, and our careful sample selection should have excluded this. Based on this premise, we suspect that the youngest age is closest to the true age of the sediment deposition, and as short-lived plant macrofossils are the youngest organic component they are likely to provide the best chronology for palaeoenvironmental research in the mires of eastern Australia.
Table 2 The age of the oldest and youngest organic fraction from the sediment samples considered in this research. Here age is determined using the mid-point of the P_sequence depth-age model.
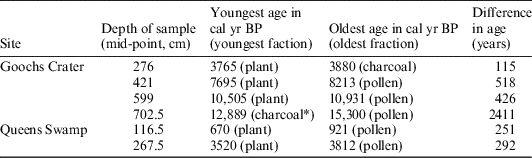
* It is possible that this “charcoal” sample was contaminated with oxidized but not burnt plant roots.
Although pollen concentrates have been widely used as 14C dating targets in Australasia (Vandergoes and Prior Reference Vandergoes and Prior2003; Newnham et al. Reference Newnham, Vandergoes, Garnett, Lowe, Prior and Almond2007; Porch and Kershaw Reference Porch and Kershaw2007; Blois et al. Reference Blois, Williams, Grimm, Jackson and Graham2011; Moss et al. Reference Moss, Tibby, Petherick, McGowan and Barr2013) here they seem to provide dates that are inconsistent with the other fractions. As acknowledged by palynologists, simple preparation techniques for concentrating pollen do not remove charcoal and can leave traces of other (oxidation resistant) organic matter, and hence the “pollen” samples are likely to be a mix of pollen and spore grains, charcoal and some other organic matter (and hence are referred to as “pollen-rich” herein). Although we used a relatively simple preparation for concentrating pollen, this is a standard method used by many labs. The alkali treatment that is standard for many pollen preparations may also allow atmospheric CO2 to precipitate, and although this is probably re-liberated during acetolysis or during pre-treatment, it should be considered. This possibility is however not consistent with our results, as our pollen-rich residues were always older than the other samples from the same depth.
As is typical for humid landscapes in eastern Australia, both Goochs Crater and Queens Swamp are fire-prone landscapes and the pollen-rich residues had a small but not insignificant charcoal component. It is hence possible that charcoal and other oxidation-resistant organic material in these pollen-rich residues is contributing to older apparent ages. The observation that these pollen-rich samples are (on average) older than even the charcoal samples perhaps relates to the size fraction of the latter. Charcoal sizes were <130 μm in our pollen preparations but >250 μm in the macro-charcoal samples, and it is notable that Blong and Gillespie (Reference Blong and Gillespie1978) reported smaller inbuilt ages with larger charcoal fractions.
The larger inbuilt age of the pollen-rich samples also raises the possibility that some pollen might persist in the environment longer than is commonly assumed. This might be especially true for some of the more robust pollen grains, such as some Myrtaceae, members of which comprise a significant component of the vegetation surrounding our sites (e.g. Eucalyptus, Leptospermum) during the Holocene (Black et al. Reference Black, Mooney and Attenbrow2008). Interestingly, Clymo and MacKay (Reference Clymo and MacKay1987) showed that pollen sized material can migrate through a sediment profile, and this potential is conceivably enhanced under the variable hydrological regimes observed in the mires of eastern Australia. However, given that our pollen-rich samples were older than other components we can discount the possibility that this inbuilt age is related to the downward vertical migration of pollen in the relatively unconsolidated upper (acrotelm) sediments.
Our results are consistent with Mensing and Southon (Reference Mensing and Southron1999) who, based on comparison with tephrochronology, found that dates from pollen samples were consistently older than the surrounding sediment. Kilian et al. (Reference Kilian, van der Plicht, van Geel and Goslar2002) also found that 14C dating using palynological preparations were about 660 years too old (based on annual lamination counts) and sensibly recognized that these samples were admixtures of not only pollen but also other organic remains. Vandergoes and Prior (Reference Vandergoes and Prior2003) also found that pollen concentrates were consistently older than other organic fractions, and they attributed this difference to contamination by plant material or humic acids. However, in our situation this explanation seems unlikely.
We conclude that pollen preparations for 14C-dating should be used with some caution, especially when sediments include a significant charcoal component. This conclusion contrasts somewhat with Brown et al. (Reference Brown, Nelson, Mathewes, Vogel and Southon1989), although their advocacy for the radiocarbon dating of pollen was really in comparison with bulk sediment dating. Our conclusion is also somewhat different from that of Dodson and Zhou (Reference Dodson and Zhou2000) who found indistinguishable ages between paired charcoal and pollen dates. This difference might relate to site characteristics (catchment size, topography, climate): nonetheless we suggest that caution is necessary.
We also found a consistent age difference between charcoal and short-lived plant macrofossils with most of the charcoal samples having an inbuilt age between 45 and 245 years, and this difference often increased with age. The only anomaly to this generalization is discussed below. Further refining the charcoal sample by isolating a larger size fraction could potentially reduce this difference (Blong and Gillespie Reference Blong and Gillespie1978), however, larger pieces of macro-charcoal are not particularly common in these sediments. Smaller charcoal fragments can however be a significant portion of the sediment obtained from these sites and the inbuilt age that we observed is likely to result in less accuracy for dates based on bulk sediment samples.
Notably, the oldest group of samples (Goochs Crater Core G3 701–702 cm) was the only anomaly from the otherwise consistent order of the ages of the fractions (usually short-lived plant macrofossils were youngest in age, macro-charcoal charcoal in the middle and the pollen-rich fraction the oldest). In this case the macro-charcoal sample (OZR370) was anomalously young (Figures 2 and 3). We have re-examined the fraction dated for OZR370 and it is more consistent with oxidized (but not pyrolysized) plant material and hence the anomalous date may reflect contamination by roots. These samples have a late Pleistocene age, arguably within, or just preceding, the Antarctic Cold Reversal (Bostok et al. Reference Bostock, Barrows, Carter, Chase, Cortese, Dunbar, Ellwood, Hayward, Howard and Neil2013), and they occur within a period of very low charcoal accumulation (Mooney unpublished data; Black et al. Reference Black, Mooney and Attenbrow2008). This period of low charcoal accumulation might reflect the climate and vegetation of the time, when productivity and fuel loads were unlikely to sustain fire.
The samples from Queens Swamp, which has a much larger catchment than Goochs Crater (Figure 1), exhibited similar age differences between macro-charcoal and short-lived plant macrofossils, suggesting that catchment size does not contribute to the variation, although this generalization needs further testing. The relatively close age of the macro-charcoal to the other dated fractions at Queens Swamp discounts the possibility of reworking of previously deposited material at the core site.
Although isolating short-lived plant macrofossils from organic sediments can be relatively simple they are not always present, and their identification can be difficult, especially in well-humified or older sediments. Mistaking roots for plant macrofossils could result in significant errors of up to as much as 4000 years (too young) since root penetration can exceed 2 m in these environments (using the average rates of deposition reported herein).
Our results demonstrate the importance of selecting an appropriate target material when dating accumulating organic sediments in eastern Australia. We found that radiocarbon dating of short-lived plant macro-fossils results in younger ages, which are potentially closer to the age of the deposition of the sediment. If they are available, we advocate their use as a dating target in palaeoenvironmental research. When they are not available the largest charcoal fraction is likely to produce a reasonably accurate chronology, especially in sediments of Late Pleistocene to Holocene age.
ACKNOWLEDGMENTS
The 14C dates were funded by AINSE Facilities Grants ALNGRA14019 and ALNGRA15019 awarded to SM. The work was also supported by the ANU/Temperate Highland Peat Swamps on Sandstone Research Program Joint Venture project The environmental history of temperate highland sandstone swamps with a focus on climatic variability and fire. LM was supported by an Australian Postgraduate Award. Geoff Hope, Xianglin Zheng, Natalie Brennan, and Karina Judd assisted with field sampling. This paper benefited from the thoughtful comments of Dr Susan Zimmerman on an earlier version and two anonymous reviewers.