INTRODUCTION
Marine mollusk shells can be a very useful source of palaeoarchives in coastal and continental shelf waters, particularly with regard to their stable isotopes (δ13C and δ18O) (e.g. Simstich et al. Reference Simstich, Erlenkeuser, Harms, Spielhagen and Stanovoy2005a, Reference Simstich, Harms, Karcher, Erlenkeuser, Stanovoy, Kodina, Bauch and Spielhagen2005b; McConnaughey and Gillikin Reference McConnaughey and Gillikin2008; Mettam et al. Reference Mettam, Johnson, Nunn and Schöne2014; Reich et al. Reference Reich, Warter, Wesselingh, Zwaan, Lourens and Renema2015) and trace metal composition (Mg/Ca, Sr/Ca) (e.g. Batenburg et al. Reference Batenburg, Reichart, Jilbert, Janse, Wesselingh and Renema2011; Warter et al. Reference Warter, Muller, Wesselingh, Todd and Renema2015). The shell composition of many mollusk species is likely to represent ambient seawater conditions and can be used to trace the physical and chemical properties of the surrounding waters. Furthermore, mollusk carbonate can provide a direct chronology of environmental change through radiocarbon (14C) dating.
The disequilibrium between marine and atmospheric 14C concentrations requires that the resulting marine reservoir age must be taken into account when using marine sourced samples to construct a chronology. Marine reservoir ages have been generated for a number of sites (Cage et al. Reference Cage, Heinemeier and Austin2006; Rick et al. Reference Rick, Henkes, Lowery, Colman and Culleton2012; Hadden and Cherkinsky Reference Hadden and Cherkinsky2015, Reference Hadden and Cherkinsky2017a, Reference Hadden and Cherkinsky2017b) but further uncertainty is introduced as mollusks inhabit a range of ecological niches. The usual assumption is that shell carbonate is precipitated from dissolved inorganic carbon in seawater; however, shell formation can be influenced by secondary factors such as metabolic processes (vital effect) and feeding strategies (DeNiro and Epstein Reference DeNiro and Epstein1978; Tanaka et al. Reference Tanaka, Monaghan and Rye1986; Gillikin et al. Reference Gillikin, Lorrain, Bouillon, Willenz and Dehairs2006; McConnaughey and Gillikin Reference McConnaughey and Gillikin2008). This may be of significance in the case of deep burrowing and deposit feeding mollusks, as they can incorporate in their shells carbon from old calcareous sediments resulting in apparently older ages than the true age of seawater dissolved inorganic carbon (e.g. Cage et al. Reference Cage, Heinemeier and Austin2006; Mangerud et al. Reference Mangerud, Bondevik, Gulliksen, Karin Hufthammer and Høisæter2006). Additionally, a recent study reported that old, but bioavailable organic matter found in arctic aquatic environments due to thawing of permafrost (yedoma) can be a source of old carbon in the diet of aquatic organisms, causing an overall “aging” effect (Guillemette et al. Reference Guillemette, Bianchi and Spencer2017). Several studies purport to find apparent 14C age differences between contemporaneous mollusk specimens (e.g. Forman and Polyak Reference Forman and Polyak1997; Hogg et al. Reference Hogg, Higham and Dahm1998; Yoneda et al. Reference Yoneda, Kitagawa, van der Plicht, Uchida, Tanaka, Uehiro, Shibata, Morita and Ohno2000; Rick et al. Reference Rick, Henkes, Lowery, Colman and Culleton2012; England et al. Reference England, Dyke, Coulthard, Mcneely and Aitken2013; Jull et al. Reference Jull, Burr and Hodgins2013; Hadden and Cherkinsky Reference Hadden and Cherkinsky2015, Reference Hadden and Cherkinsky2017a, Reference Hadden and Cherkinsky2017b); whereas others claim that no significant age difference is observed between species from different ecological settings (Harkness Reference Harkness, Mook and Waterbolk1983; Berkman and Forman Reference Berkman and Forman1996; Eiríksson et al. Reference Eiríksson, Larsen, Knudsen, Heinemeier and Símonarson2004; Ascough et al. Reference Ascough, Cook, Dugmore, Scott and Freeman2005; Tisnérat-Laborde et al. Reference Tisnérat-Laborde, Paterne, Métivier, Arnold, Yiou, Blamart and Raynaud2010).
Similarly, the impact of vital effects and feeding strategies on stable isotopes is still under debate. Some studies report high internal variability in marine mollusk stable isotopes that would prevent their use as robust environmental proxies (e.g. Gillikin et al. Reference Gillikin, Lorrain, Bouillon, Willenz and Dehairs2006); whereas others used stable isotopes to identify palaeoenvironments and ecological processes within these environments (e.g. Mettam et al. Reference Mettam, Johnson, Nunn and Schöne2014; Reich et al. Reference Reich, Warter, Wesselingh, Zwaan, Lourens and Renema2015).
To assess the reliability of 14C ages and stable isotopes, we analyzed 9 species of known-age marine bivalves collected in 6 localities around the NE Atlantic coast (Figure 1). By comparing live-collected pre-bomb marine bivalve 14C ages and stable isotopes from (i) replicate samples of a single species from the same locality (ii) the same species at different localities and (iii) different species at the same locality, we examined in situ, intra-species and inter-species variability in a wider context than has been previously undertaken.
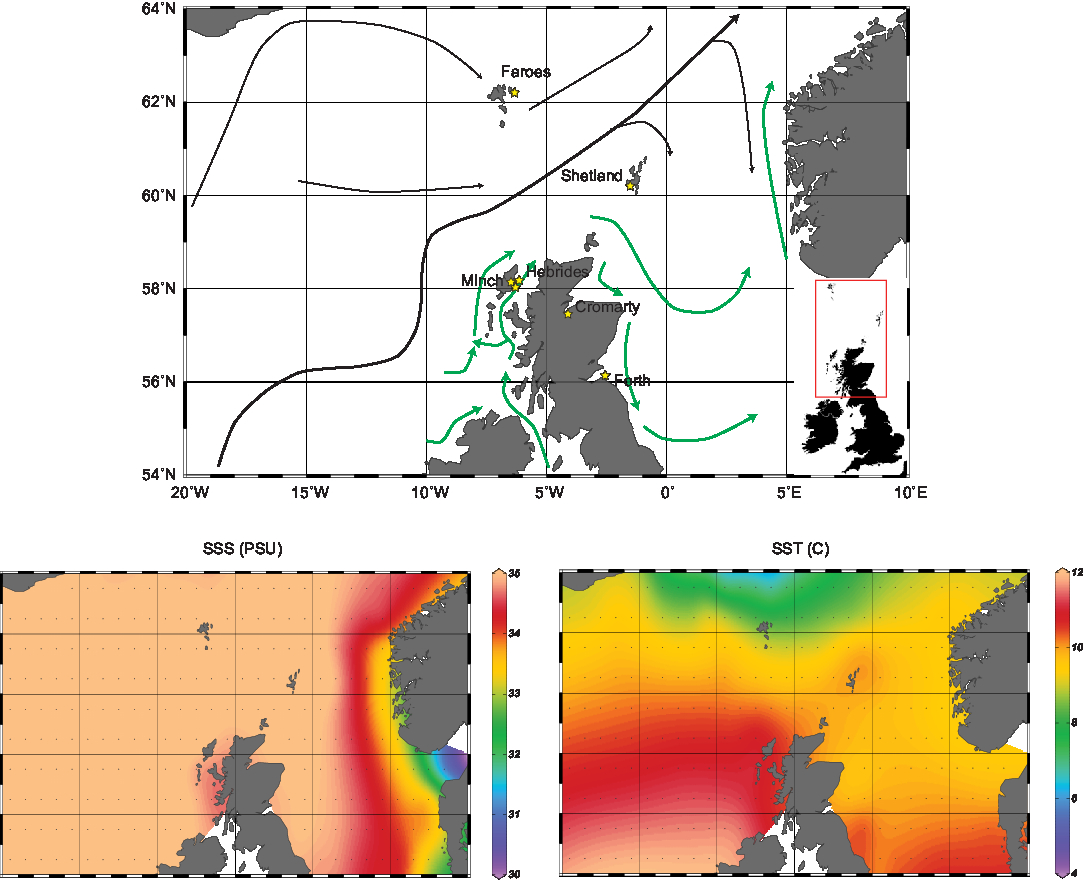
Figure 1 Location map of samples analyzed in this study (stars), and circulation map representing major current pathways after Austin et al. (Reference Austin, Hibbert, Rasmussen, Peters, Abbott and Bryant2012) and Baxter et al. (Reference Baxter, Boyd, Cox, Donald, Malcolm, Miles, Miller and Moffat2011). Black arrows indicate North Atlantic current, green arrows indicate coastal currents. Sea surface salinity and temperature data are from GIN sea regional climatologies (Seidov et al. Reference Seidov, Baranova, Boyer, Cross, Mishonov, Parsons and Reagan2018). (Please see electronic version for color figures.)
MATERIAL AND METHODS
Area of Study
The Fishery Board of Scotland conducted a survey of bottom fauna in the northern part of the North Sea and to the north and west of Scotland during the years 1922–1925 (Stephen Reference Stephen1934). The current study focuses on six of these sites: Shetland (Gruting Voe), Cromarty Bay, Forth (St Andrews Bay), Minch (Loch Erisort), Hebrides (Loch Leurbost) and Faroes.
Grunting Voe is located in the southwest of Shetland (Figure 1). Two main types of soil are present in this catchment: peat and organic soils, both poorly draining. A few rivers and streams discharge in Grunting Voe, however freshwater influence in the voe is generally very low. This, together with an overall small tidal range, leads to wind-driven currents locally, with wind typically blowing from the south (Cefas 2010a).
Cromarty Bay is a shallow, enclosed basin adjacent to the Moray Firth on the east coast of Scotland (Figure 1). Two types of soils are characteristic of the area: peaty soils and most predominantly humus iron podzols, the latter being freely draining and potentially reducing runoff. The catchment area includes one large river (River Conon) and smaller watercourses that seasonally supply freshwater to the Cromarty Bay. Predominant winds are from the north east and, in combination with density (freshwater) flows, can drive local currents. Tidal energy is strong in the Cromarty Firth, but tidal currents seems to slow down in proximity of the Bay where a sand bar is deposited (Cefas 2010b).
The Forth is situated on the eastern coast of Scotland bathing the city of St Andrews after which the Bay takes its name (Figure 1). Nowadays, this is an urbanized area and most of the land is arable, improved grassland. In proximity of the Forth Estuary, prevailing currents are tidal and freshwater inputs have a very marginal influence on local hydrography. Local currents are overall relatively weak and the Forth is more of a coastal/marine environment than an estuary (Cefas 2013).
The Minch bathes the eastern coast of the Isle of Lewis in the Outer Hebrides (Figure 1). The catchment area of Loch Erisort is dominated by peaty soils with the sparse presence of organic and brown forest soils. Currents can be highly impacted by seasonal freshwater inputs from local streams resulting in density flows, but are generally quite weak. The rough topography of the seafloor may enhance local currents close to constrictions, and wind-driven currents usually prevail over tidal energy (Cefas 2010c).
The Hebrides site, Loch Leurbost, is also situated on the eastern coast of the Isle of Lewis, just north of the Minch site (Figure 1). Two types of soils characterize this loch: brown forest soils (freely draining) on the northern coast and peaty soils (poorly draining) inland to the north of the loch and along the southern coastline. Currents are generally weak but can be channeled by changes in local bathymetry owing the presence of the island of Tannaraidh and other smaller islands at the mouth of the loch as well as sills between the head, inner and outer basins. Additionally, density flows can occur seasonally following heavy rain periods and enhanced riverine inputs (Cefas 2011).
The Faroe Islands are situated between Iceland, Norway and Scotland (Figure 1), in a region where Atlantic Ocean waters enter the Nordic Seas. A persistent tidal flow encompasses Faroes’ shelf waters separating this coastal environment from the open ocean. Faroes coast is jagged by many fjords and consists of steep cliffs and mountainous plateaus, which are part of the North Atlantic basalt region that formed in the Tertiary during a period of intense volcanism (Gillespie and Clague Reference Gillespie and Clague2009).
Overall, these six sites are either calm depositional fjordic environments (Shetland, Minch and Hebrides) or low energy enclosed coastal basins sheltered from the open ocean (Cromarty, Forth and Faroes), with relatively stable annual sea surface temperatures and salinities (Figure 1). With the exception of the Forth, there are no limestones in the area of study which means that we can exclude potential “old” carbon contaminations due to 14C-depleated dissolved inorganic carbon in all other sites. A fairly uniform water mass bathe the UK continental slope as its steepness limits the amount of deep North Atlantic waters entering the shelf (Inall et al. Reference Inall, Gillibrand, Griffiths, MacDougal and Blackwell2009; Baxter et al. Reference Baxter, Boyd, Cox, Donald, Malcolm, Miles, Miller and Moffat2011). To the west, the main water mass stems from the warm and saline Eastern North Atlantic Central Water, which travels northward along the west coast of Scotland (Inall et al. Reference Inall, Gillibrand, Griffiths, MacDougal and Blackwell2009). Similarly, the Scottish Coastal Current also travels northward carrying waters from the Irish and Clyde Seas and experiencing a slight freshening in proximity of the fjords peppering the coast of western Scotland (Inall et al. Reference Inall, Gillibrand, Griffiths, MacDougal and Blackwell2009; Baxter et al. Reference Baxter, Boyd, Cox, Donald, Malcolm, Miles, Miller and Moffat2011) (Figure 1). To the east, the circulation in the North Sea is typically anti-clockwise and fresh and cool North Atlantic waters enter this region from Orkney and Shetland and along the Norwegian Trench (Baxter et al. Reference Baxter, Boyd, Cox, Donald, Malcolm, Miles, Miller and Moffat2011) (Figure 1).
Sampling Strategy
Samples were collected from both intertidal areas by hand-sampling a fixed volume (0.5 × 0.5 × 0.15 m), or from below the low watermark using a Petersen grab (0.1 m3 volume). Material was washed through 2 mm (intertidal samples) and 1.5 mm (grab samples) mesh sieves, before counting and identification of the mollusk fauna. The collection now resides with the National Museum of Scotland (reg. no.: NMSZ 1951.19), and specimens are stored dry in airtight glass vials or sealed polyethylene bags. Table 1 lists the nine species analyzed in this study and their ecologies and habitats; Table 2 includes the samples available for stable isotopes and 14C analysis. Five of the six sites contain more than one of these species, typically including a combination of suspension feeding, deposit feeding or mixed feeding species (Table 2). No records exist to conclusively indicate all specimens were live-collected, although this was certainly the intent of the original project, and so the remote possibility exists for some of the material to be reworked and potentially diagenetically altered. To improve the likelihood of the samples being live on collection, all samples analyzed were articulated bivalves with intact pereostracum, and frequently with internal organic matter still present.
Table 1 Shells species, ecologies, and habitats.*

* Ecology and habitat information is from Tebble (Reference Tebble1966).
Table 2 Sample list.*

* All samples come from coastal waters. Feeding strategies are largely based on the interpretations of Tebble (Reference Tebble1966).
** Replicate analyses. Please note that replicates (a), (b), and (c) refer to individual samples, not repeat measurements on the same shell.
Stable Isotopes and Radiocarbon Analyses
Samples were weighed and placed in a 0.2 M HCl solution overnight to ensure dissolution of about 20% of the outer carbonate. This pre-treatment also loosened any pereostracum adhering to the outer shell, which was mechanically removed along with any other surficial organic material attached to the shell. Samples were washed repeatedly with distilled water to remove any remaining acid, then freeze-dried and finally ground prior to analysis. Subsamples were taken for stable isotope mass spectrometry and 14C analyses. Stable isotopes measurements were performed at the University of St Andrews in a Gasbench autosampler coupled to a ThermoScientific Finnigan Delta plus mass spectrometer. Standard reference materials (IAEA C2 travertine and Iceland Spar calcite) were analyzed along with the samples for quality control with a calcite acid fractionation factor of 1.01025 at 25ºC (Sharma and Clayton Reference Sharma and Clayton1965) and internal precision (zero enrichment test) of ±0.05‰ for δ13C and ±0.1‰ for δ18O measurements. For accelerator mass spectrometry 14C (AMS 14C) analysis, samples were hydrolyzed to CO2 using 100% H3PO4 (phosphoric acid), and then gas cryogenically trapped into sealed quartz-glass tubes. Age measurements were made at the NERC Radiocarbon Laboratory, East Kilbride (SUERC).
Statistical Treatment of Data
We subdivided our dataset in three subsets to investigate: in situ variability (same species—same locality), intra species variability (same species—different localities) and inter species variability (different species—same locality). Note that replicate samples used to discuss in situ variability refer to individual samples, not repeat measurements on the same shell. We used the method detailed in Ward and Wilson (Reference Ward and Wilson1978) to test for homogeneity within groups of 14C measurements.
To understand whether ages are comparable and thus can be combined together, we determined the pool mean (Ap) of each subset of measurements

and then calculated

where Ai is the measured 14C age and Si is the associated 1σ error. When T was less than the critical value for α=0.05, then 14C ages are not statistically different and can be combined together.
RESULTS AND DISCUSSIONS
In situ Variability: Same Species—Same Locality
For the Shetland dataset, we have replicate samples only of A. nitida (Table 3). Based on three measurements (5 shells were measured in total), δ13C varies from –0.1 to 0.1‰, δ18O varies between 2.7 and 3.2‰ and 14C dates vary from 467 to 510 BP (Figures 2 and 3).
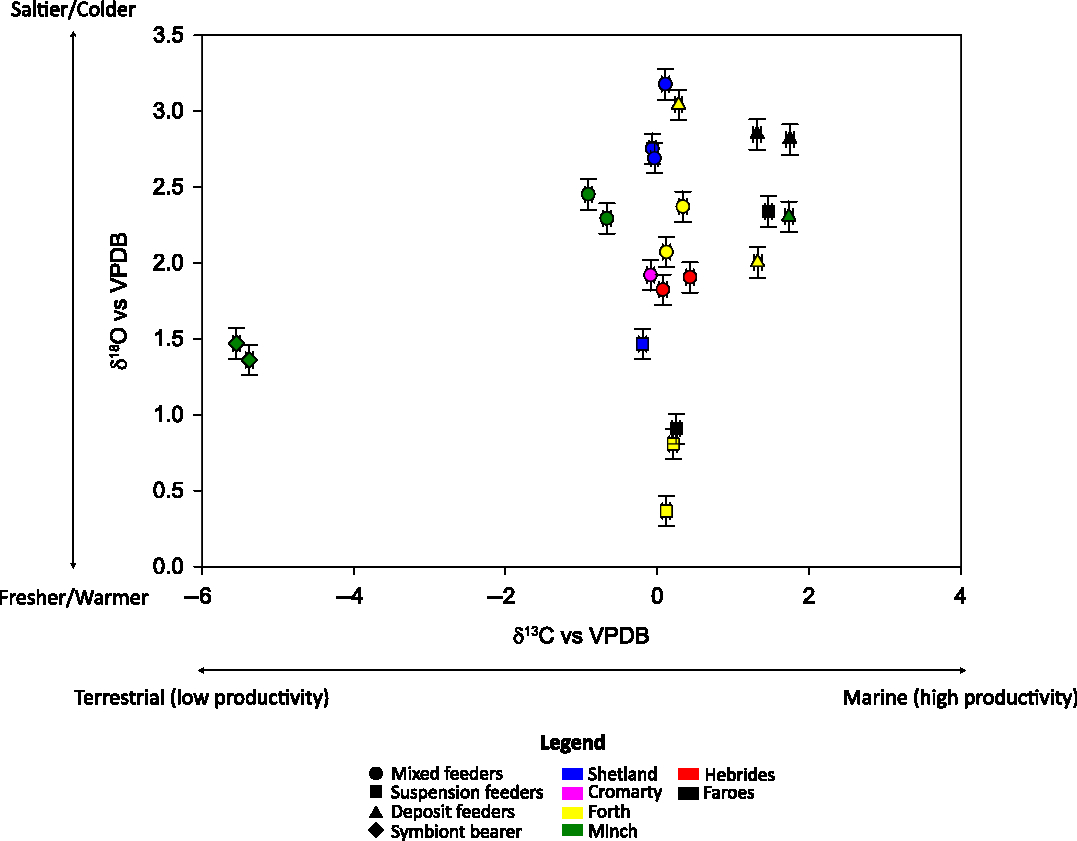
Figure 2 Stable isotopes discriminate between different ecological settings. Circles mark mixed feeders, squares suspension feeders, triangles deposit feeders and diamonds symbiont bearers. Samples from Shetland are in blue, from Cromarty in pink, from Forth in yellow, from Minch in green, from Hebrides in red, and from Faroes in black. Error bars represent the instrumental errors on δ18O and δ13C.

Figure 3 14C measurements. a) In situ variability. b) Spatial variability in Abra spp. c) Spatial variability in Nucula spp. d) Inter species variability; stars mark average 14C age at each location regardless of species. Red lines mark the average 14C age of each subset of data, empty symbols mean no replicate measurement was available. Error bars represent ± 1σ error of 14C ages.
Table 3 In situ variability.*

* Red numbers indicate when in situ variability is statistically significant for α=0.05. (Please see electronic version for color.)
For the Forth dataset, duplicate samples of A. alba (5 shells), C. gallina (4 shells) and N. turgida (4 shells) were analyzed (Table 3). For A. alba, δ13C varies between 0.1 and 0.3‰, δ18O varies from 2.1 to 2.4‰ and 14C ages vary from 496 to 517 BP (Figures 2 and 3).
For C. gallina, δ13C varies between 0.1 and 0.2‰, δ18O varies from 0.4 to 0.8‰ and 14C dates vary between 417 and 464 BP (Figures 2 and 3).
For N. turgida, δ13C varies between 0.3 and 1.3‰, δ18O varies between 2.0 and 3.0‰, and 14C ages vary between 447 and 462 BP (Figures 2 and 3)
For the Minch dataset, we have duplicate samples of A. alba (4 shells) and T. flexuosa (3 shells) (Table 3). For A. alba, δ13C varies between –0.9 to –0.7‰, δ18O varies from 2.3 to 2.5‰, and 14C dates vary from 448 to 552 BP (Figures 2 and 3).
For T. flexuosa, δ13C varies between –5.5 and –5.4‰, δ18O varies from 1.4 to 1.5‰, and 14C ages vary between 506 and 532 BP (Figures 2 and 3).
For the Faroes dataset, we have duplicate measurements only of N. nucleus (5 shells) (Table 3). δ13C varies between 1.3 and 1.7‰, δ18O varies around 2.8 ‰ (Figure 2) and no duplicate 14C date is available for this sample.
In general, in situ variability in stable isotope measurements is low due to the precision of the measurements, yet statistically significant (Figure 2). In Figure 2 we reported individual stable isotope measurements to show the overall variability of the dataset. Four groups can be identified based on mollusks’ feeding strategies and shells stable isotope composition: symbiont bearer, suspension feeders, mixed feeders and deposit feeders (Figure 2—different symbols shape). The highest in situ variability in δ13C measurements was found in N. turgida and N. nucleus possibly because they are deposit feeders that can incorporate into their shells carbon from different sources (Yonge Reference Yonge1939; Ruppert et al. Reference Ruppert, Fox and Barnes2004; Cage et al. Reference Cage, Heinemeier and Austin2006; Mangerud et al. Reference Mangerud, Bondevik, Gulliksen, Karin Hufthammer and Høisæter2006). Notably, terrestrial organic matter is enriched in 12C from soils resulting in lower δ13C values than marine organic matter (e.g. Ficken et al. Reference Ficken, Barber and Eglinton1998; Balasse et al. Reference Balasse, Tresset, Dobney and Ambrose2005; Schmidt and Gleixner Reference Schmidt and Gleixner2005; Marconi et al. Reference Marconi, Giordano and Raven2011; Schiener et al. Reference Schiener, Black, Stanley and Green2014). Similarly, A. alba δ13C in situ variability is statistically significant albeit small (Figure 2). Most likely this is the result of A. alba being a mixed feeder that can process a large volume of sediments to feed on detritus when it does not suspension feed (Dame Reference Dame1996). The presence of symbionts seems to drive the unique δ13C of T. flexuosa (Mangerud et al. Reference Mangerud, Bondevik, Gulliksen, Karin Hufthammer and Høisæter2006), whereas no in situ variability is observed in the suspension feeder C. gallina δ13C (Figure 2). In situ variability in δ18O is significant in A. alba, C. gallina and N. turgida from Forth and in A. nitida from Shetland. We suggest that in situ changes in δ18O could be either due to changes in microhabitats or to differences in time-averaging among individuals. These mollusks are infaunal burrowers able to move within the sediments depending on food availability and changes in environmental conditions. This implies that the measured δ18O possibly reflects microhabitat preferences. Additionally, five specimens of A. nitida (Shetland), four of C. gallina, five of A. alba and four of N. turgida (Forth) were ground together before being measured, which means that the observed variability could also relate to seasonal changes in sea water conditions over a number of years, depending on the individual specimen’s lifespan. While these are known to be short-lived species, no information is available on shells’ lifespan, thus we have no means to check for differences in ontogenetic ages.
In contrast, no significant in situ variability is observed in 14C ages and all shells are the same age within the ± 1σ error, with the exception of A. alba from Minch (Table 3). However, all shells are the same age when considering the ± 2σ error on 14C ages, and no “old” carbon effect was observed in the Forth dataset suggesting lack of weathering of the Carboniferous limestone (Figure 3a).
Intra-Species Variability: Same Species—Different Location
The mixed feeder Abra spp. (A. alba and A. nitida together) is present at five of the six locations: Shetland, Cromarty, Forth, Minch and Hebrides (Figure 1), thus enabling inter-locality comparison of stable isotope and 14C measurements. Based on all five localities, spatial variability is significant in Abra spp. stable isotopes (Figure 2—circles), whereas it does not affect 14C ages within the ± 1σ level of uncertainty (Figure 3b; Table 4).
Table 4 Abra spp. (mixed feeder) inter-locality variability.

Nucula spp. is present at three of the six locations: Forth, Minch and Faroes (Figure 1), allowing to assess spatial variability of stable isotope and 14C measurements in deposit feeders. Considering all three localities together, spatial variability is significant in Nucula spp. δ13C and δ18O (Figure 2—triangles), whereas it does not affect 14C ages within the ± 1σ level of uncertainty (Figure 3c; Table 5).
Table 5 Nucula spp. (deposit feeder) inter-locality variability.

In summary, marine bivalve stable isotopes record seasonal changes in local environments and can be a useful source of palaeoarchives in coastal and continental shelf waters (Figure 2). The spread in δ18O measurements in Abra spp. seems to reflect the temperature gradient between the sites with colder temperature in Shetland (high δ18O) and warmer temperature at the Hebrides (low δ18O) (Figures 1 and 2). The range of δ13C values most likely represent changes in local productivity often related with seasonal variations in freshwater and nutrient supplies (Figures 1 and 2). In contrast, 14C ages do not change significantly with location, and all measured bivalves (Abra spp. and Nucula spp.) are the same age within the ± 1σ level of uncertainty (Figures 3b and 3c; Tables 4 and 5).
Inter-Species Variability: Different Species—Same Location
To discuss inter-species variability, in Shetland, we measured stable isotopes and 14C ages in the shells of A. nitida (mixed feeder) and T. ovata (suspension feeder) (Table 6). Inter-species variability is significant in δ13C and δ18O measurements (Figure 2), whereas it does not affect 14C ages, as the two species of bivalves are the same age within the ± 1σ level of uncertainty (Figure 3d; Table 6).
Table 6 Inter-species variability.*

* Red numbers indicate when inter species variability is statistically significant for α=0.05.
In Forth, we measured stable isotopes and 14C ages in the shells of A. alba (mixed feeder), C. gallina (suspension feeder) and N. turgida (deposit feeder) (Table 6). Considering all three species together, inter-species variability is significant in δ13C and δ18O measurements (Figure 2), whereas it does not affect 14C ages, as all samples are the same age within the ± 1 σ level of uncertainty (Figure 3d; Table 6).
In Minch, we measured stable isotopes and 14C in the shells of A. alba (mixed feeder), T. flexuosa (suspension feeder with chemosynthetic symbiont) and N. nucleus (deposit feeder) (Table 6). Considering all three species together, inter-species variability is significant in δ13C and δ18O measurements (Figure 2), whereas it does not affect 14C ages, and all samples are the same age within the ± 1 σ level of uncertainty (Figure 3d; Table 6).
In Faroes, we measured stable isotopes and 14C in the shells of Astarte borealis (suspension feeder), Astarte elliptica (suspension feeder) and N. nucleus (deposit feeder) (Table 6). In this particular case, combining data from all three species, inter-species variability is significant for both stable and radiogenic isotopes (Figures 2 and 3d; Table 6).
In summary, stable isotopes in marine bivalve shells can be reliably used to identify feeding strategies and ecological settings (Figure 2). Only A. elliptica falls outside the corresponding “feeding box” (Figure 2; black square in triangles group), perhaps suggesting a problem with the measurements performed in this shell or its identification. In contrast, 14C ages show no evidence of sensitivity to mollusk diets or microhabitats, as all analyzed shells are the same age within the ± 1σ level of uncertainty (Figure 3d; Table 6). This includes the measurements from Faroes that locally showed significant interspecies variability (Table 6). Nevertheless, we acknowledge that with higher precision dates (± 1σ < 35 yr) the difference in 14C ages between specimens with different ecologies and feeding strategies might become significant.
Does Diet Affect Radiocarbon Ages?
Previously published 14C ages, based on several species of mollusks and covering a range of environments, showed significant age differences between contemporaneous specimens (e.g. Forman and Polyak Reference Forman and Polyak1997; Hogg et al. Reference Hogg, Higham and Dahm1998; Mangerud et al. Reference Mangerud, Bondevik, Gulliksen, Karin Hufthammer and Høisæter2006; England et al. Reference England, Dyke, Coulthard, Mcneely and Aitken2013; Petchey et al. Reference Petchey, Ulm, David, McNiven, Asmussen, Tomkins, Dolby, Aplin, Richards, Rowe, Leavesley and Mandui2013; Hadden and Cherkinsky Reference Hadden and Cherkinsky2017a; Rick et al. Reference Rick, Henkes, Lowery, Colman and Culleton2012; Rick and Henkes Reference Rick and Henkes2014). In these studies, older than expected 14C ages were consistently measured in deposit feeding mollusks, as these species were argued to be able to incorporate old carbon in their shells. This apparent ‘aging effect’ can be due to two sources of “old” carbon: inorganic carbon from old calcareous sediments and/or terrestrial inputs (Forman and Polyak Reference Forman and Polyak1997; England et al. Reference England, Dyke, Coulthard, Mcneely and Aitken2013), and old organic matter (yedoma) from the underlying sediments in samples coming from arctic regions (Forman and Polyak Reference Forman and Polyak1997; Guillemette et al. Reference Guillemette, Bianchi and Spencer2017). The latter source of old carbon could potentially explain the older 14C ages reported in Mangerud et al. (Reference Mangerud, Bondevik, Gulliksen, Karin Hufthammer and Høisæter2006) and England et al. (Reference England, Dyke, Coulthard, Mcneely and Aitken2013) as their samples come from Svalbard, the Barents Sea and the Arctic Ocean. Additionally, high variability in 14C ages within and between shells was found in samples collected in estuarine and brackish environments due to the mixing of marine and 14C-depleted fresh waters and to carbon recycling (Hadden and Cherkinsky Reference Hadden and Cherkinsky2017a; Rick et al. Reference Rick, Henkes, Lowery, Colman and Culleton2012; Rick and Henkes Reference Rick and Henkes2014). As a consequence, several researchers have suggested to either (i) exclude deposit feeders from palaeoenvironmental reconstructions, (ii) recommended species-specific correction factors to calibrate 14C ages measured in deposit feeders, or (iii) advocated for the calculation of regional and sub-regional reservoir ages (Forman and Polyak Reference Forman and Polyak1997;Petchey et al. Reference Petchey, Ulm, David, McNiven, Asmussen, Tomkins, Dolby, Aplin, Richards, Rowe, Leavesley and Mandui2013; Hadden and Cherkinsky Reference Hadden and Cherkinsky2017a; Rick et al. Reference Rick, Henkes, Lowery, Colman and Culleton2012; England et al. Reference England, Dyke, Coulthard, Mcneely and Aitken2013; Rick and Henkes Reference Rick and Henkes2014).
In this study, we combine stable isotopes and 14C measurements for a range of marine bivalves having different feeding strategies and inhabiting a variety of ecological niches in the NE Atlantic. By pairing stable isotopes and 14C measurements, we were able to better understand the mollusks’ ecology (Figure 2) and thus better assess the reliability of 14C ages (Figure 3). In situ variations of shell δ13C may reflect changes in carbon source, namely whether a mollusk is feeding on marine or terrestrial organic matter or switching between feeding strategies (Figure 2). Spatial changes in shell δ13C may indicate seasonal variations in marine productivity often related to freshwater input in fjordic and coastal systems (Figures 1 and 2). Similarly, in situ variations of δ18O values can be due to differences in microhabitats and/or in shells time-average due to possibly different ontogenetic ages of the analyzed specimens (Figure 2), whereas spatial changes in δ18O may relate to seasonal changes in seawater temperature and salinity (Figures 1 and 2). In contrast, no statistically significant variation in 14C measurements has been recorded in the compiled dataset (Figure 3d) and we would argue that all studied species can be used to provide a robust 14C chronology independently of their diet. We speculate that, given the oceanography and geology of these sites in the NE Atlantic, seawater dissolved inorganic carbon is fairly homogeneous and unaffected by “old” carbon derived from the weathering of older rocks, ultimately resulting in the robust 14C ages we measured (Figure 3). As already proposed by Petchey et al. (Reference Petchey, Ulm, David, McNiven, Asmussen, Tomkins, Dolby, Aplin, Richards, Rowe, Leavesley and Mandui2013), pairing stable isotopes and 14C ages provides an opportunity for a deeper understanding of environmental influences on 14C dates, ultimately enabling an improved resolution of 14C based chronologies.
CONCLUSIONS
The main conclusion that can be drawn from these results is that marine bivalve shells from the NE Atlantic can be used not only as reliable proxies for environmental and ecological change, but also for dating such reconstructions. As previously described in the literature, stable isotopes record changes in local environment, circulation (water mass) and ecology, being useful sources of palaeoarchives in coastal and continental shelf waters. Additionally, our results highlight that, in the NE Atlantic, mollusk carbonate provides a reliable basis for building the chronology of marine environmental change through 14C dating, independently of vital effects and differences in microhabitats, feeding strategies and sample location. Reliable 14C dating may still be compromised by the unknown incorporation of “old” carbon in mollusk shells in regions with “old” limestones. We therefore recommend the routine pairing of 14C and stable isotopes (δ13C and δ18O) measurements to provide an improved ecological context for interpreting 14C ages.
ACKNOWLEDGMENTS
We acknowledge the support of NERC and an award to WA through the RAPID program (project NE/C000137/1) and we thank the National Museum of Scotland for making the shell material available to us. Many of these analyses were conducted with the support of Louise Brown at the University of St Andrews, working alongside colleagues at SUERC, East Kilbride—notably Steve Moreton and Charlotte Bryant; we thank all these colleagues for their help. We thank two anonymous reviewers and the Associate Editor Dr Quan Hua for valuable and constructive comments that improved the manuscript. We acknowledge the support of the University of St Andrews in providing laboratory facilities for this research.