INTRODUCTION
Radiocarbon (14C) dating of skeletal material is severely hindered by the poor survival of protein in the hot environments that are common between latitudes of 40ºN and 40ºS (roughly between the Mediterranean and southern Australia). Even with screening protocols to identify bones which may contain marginal levels of collagen (Brock et al. Reference Brock, Wood, Higham, Ditchfield, Bayliss and Ramsey2012; Jacob et al. Reference Jacob, Querci, Caparros, Barroso Ruiz, Higham and Devièse2018), many sites contain no datable bones, or too few to produce a precise Bayesian chronology (Storm et al. Reference Storm, Wood, Stringer, Bartsiokas, De Vos, Aubert, Kinsley and Grün2013; Wood et al. Reference Wood, Barroso-Ruíz, Caparrós, Jordá Pardo, Santos and Higham2013; Calo et al. Reference Calo, Prasetyo, Bellwood, Lankton, Gratuze, Pryce, Reinecke, Leusch, Schenk and Wood2015).
Several researchers have attempted to produce accurate dates on carbonate within the hydroxyapatite phase in unburnt bone, which out-survives the organic phase in all but the most acidic of environments, but with little success outside of arid regions (Haynes Reference Haynes1968; Zazzo Reference Zazzo2014; Zazzo and Saliège Reference Zazzo and Saliège2011). Carbonate in the mineral phase of tooth enamel may provide an alternative material, but again, attempts to 14C date the material have met with limited success outside of arid regions (Hedges et al. Reference Hedges, Lee-Thorpe and Tuross1995; Zazzo Reference Zazzo2014; Wood et al. Reference Wood, Duval, Mai Huong, Tuan, Bacon, Demeter, Duringer, Oxenham and Piper2016) despite the application of a range of pretreatment methods (Hedges et al. Reference Hedges, Lee-Thorpe and Tuross1995; Surovell Reference Surovell2000; Cherkinsky Reference Cherkinsky2009; Hopkins et al. Reference Hopkins, Snoeck and Higham2016). With no pretreatment, more than 10% of the carbonate in tooth enamel from karstic environments in Vietnam was found to be a contaminant (Wood et al. Reference Wood, Duval, Mai Huong, Tuan, Bacon, Demeter, Duringer, Oxenham and Piper2016), and in some cases tooth enamel appears to contain more carbonate contamination than bone apatite (Zazzo Reference Zazzo2014). This is somewhat surprising given that, in comparison with bone, enamel porosity is low (Hedges et al. Reference Hedges, Lee-Thorpe and Tuross1995; Millard and Hedges Reference Millard and Hedges1996), and enamel crystallites are more stable as they are larger (26.3 × 100–1000 nm vs. 5 × 100 nm) (Bottero et al. Reference Bottero, Yvon and Vadot1992; Cui and Ge Reference Cui and Ge2007) and contain less carbonate (3.5 wt% vs. 6 wt%) (Elliott Reference Elliott2002), but may be related to protection offered by the close relationship between the mineral and protein components in well preserved bone (Zazzo Reference Zazzo2014).
Carbonate contaminants can range in age, but they are likely to have a younger 14C age than the buried tooth. Although some of the dissolved carbonate in water percolating sediments in limestone caves may derive from the bedrock and be 14C free, most is likely to derive from processes occurring within the soil above the cave, such as plant and soil respiration (Cerling Reference Cerling1984; Fohlmeister et al. Reference Fohlmeister, Voarintsoa, Lechleitner, Boyd, Brandtstätter, Jacobson and Oster2020), and thus contain young carbon. As a consequence, dates on enamel are usually found to be erroneously young (Zazzo Reference Zazzo2014).
Improvement to 14C dating methods for enamel is hindered by the limited knowledge of how tooth enamel degrades, and where the exogenous carbon sits within the hierarchical structure of enamel. Tooth enamel consists of crystallites separated by a high magnesium amorphous phosphate (Gordon et al. Reference Gordon, Cohen, MacRenaris, Pasteris, Seda and Joester2015; La Fontaine et al. Reference La Fontaine, Zavgorodniy, Liu, Zheng, Swain and Cairney2016), grouped into prisms which are woven into the enamel fabric (Simmelink and Piesco Reference Simmelink, Piesco and Avery2001). Diagenesis mechanisms which can affect carbonate isotope signatures are thought to include:
-
1. the deposition of secondary carbonates on the surface and in cracks
-
2. diffusion and adsorption of carbonate ions into pores between both the prisms and the crystallites
-
3. isotopic exchange during dissolution/precipitation of hydroxyapatite
(Lee-Thorp and van der Merwe Reference Lee-Thorp and van der Merwe1991; Zazzo et al. Reference Zazzo, Lécuyer and Mariotti2004; Zazzo and Saliège Reference Zazzo and Saliège2011; Wood et al. Reference Wood, Duval, Mai Huong, Tuan, Bacon, Demeter, Duringer, Oxenham and Piper2016). These require water to be present, meaning that teeth from arid regions are often found to be less affected by contaminants (Zazzo Reference Zazzo2014).
Enamel is rarely 14C dated outside of forensic applications, with only occasional use in arid and tropical regions. However, it is regularly analyzed for carbon and oxygen stable isotopes which are less sensitive to small amounts of contamination (Lee-Thorp Reference Lee-Thorp1989; Makarewicz and Sealy Reference Makarewicz and Sealy2015; Roberts et al. Reference Roberts, Perera, Wedage, Deraniyagala, Perera, Eregama, Petraglia and Lee-Thorp2017). Despite this, Pellegrini and Snoeck (Reference Pellegrini and Snoeck2016) have called for a consistent protocol to be adopted as results appear to differ depending on pretreatment, suggesting that the endogenous isotopic value of tooth enamel is changed by pretreatment and/or that carbonate contaminants may affect the stable isotope signature of tooth enamel.
Although a variety of methods have been proposed, both 14C and stable isotope fields typically use an acetic acid leach to remove secondary carbonates prior to analysis (Haynes Reference Haynes1968; Lee-Thorp et al. Reference Lee-Thorp, van der Merwe and Brain1989; Cherkinsky Reference Cherkinsky2009; Zazzo and Saliège Reference Zazzo and Saliège2011; Ventresca Miller et al. Reference Ventresca Miller, Fernandes, Janzen, Nayak, Swift, Zech, Boivin and Roberts2018). It is recommended that enamel is finely powdered before leaching to allow removal of carbonate contaminants in the pores between the prisms and crystallites (Lee-Thorp et al. Reference Lee-Thorp, Manning and Sponheimer1997; Wood et al. Reference Wood, Duval, Mai Huong, Tuan, Bacon, Demeter, Duringer, Oxenham and Piper2016). Whilst the surfaces exposed to acid will dissolve rapidly, the acid leach will also preferentially remove the more soluble endogenous phases of tooth enamel such as the high magnesium amorphous phosphate (Gordon et al. Reference Gordon, Cohen, MacRenaris, Pasteris, Seda and Joester2015) and crystallite cores which contain the highest concentration of carbonate (Simmelink and Piesco Reference Simmelink, Piesco and Avery2001).
Two studies have investigated whether the type of acid used to leach enamel affects the 14C date obtained (Table 1). Hedges et al. (Reference Hedges, Lee-Thorpe and Tuross1995) found that two out of three Pleistocene-aged samples from Kents Cavern, UK, produced older ages after a leach in acetic acid than HCl, and concluded that a leach with acetic acid may produce better, though still inaccurate, dates. In contrast, Hopkins et al. (Reference Hopkins, Snoeck and Higham2016) recommended the use of 0.05M HCl to leach tooth enamel after examining one Pleistocene-aged sample from Sutton Courtenay, UK, where they found treatment with acetic acid produced a younger age than treatment with more concentrated HCl. The difference was small at just 680 ± 230 14C years. Neither study reacted the sample under vacuum. Neither study was able to propose a reason why the two acids could give different results, although Hedges et al. (Reference Hedges, Lee-Thorpe and Tuross1995) surmised that “Presumably, the dissolution of hydroxyapatite by HCl is less discriminating.”
Table 1 Published 14C dates comparing age after leaching in HCl and acetic acid. Data from Kent’s Cavern is from Hedges et al. (Reference Hedges, Lee-Thorpe and Tuross1995) and data from Stanton Harcourt is from Hopkins et al. (Reference Hopkins, Snoeck and Higham2016). Data referring to dates on collagen is given in italics. Further associated data (for example yield, stable isotope, and FTIR analysis) is included in the original publication.
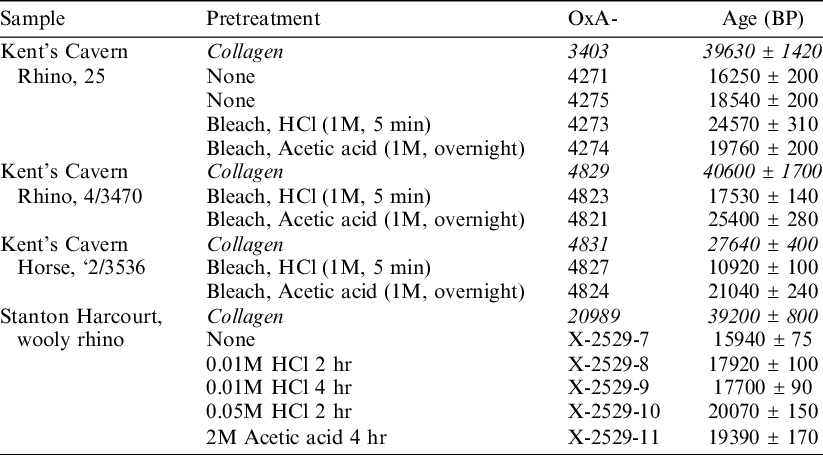
Dissolution of teeth is caused by reaction with the hydrogen ion from acids and by chelating agents (anions which can bind or complex calcium) (Featherstone and Lussi Reference Featherstone, Lussi and Lussi2006). Both play a role in enamel erosion and the formation of caries, and have been studied within dentistry. When a whole tooth is exposed to erosion, the two types of reactions preferentially attack different regions of the enamel (Simmelink et al. Reference Simmelink, Nygaard and Scott1974). When human enamel is exposed to acid the prism centers are preferentially lost, but when exposed to a chelator, the peripheral regions of the prisms are lost probably because of the larger pore sizes in this area. However, EDTA and acid have the same effect on the dissolution of separated crystals, removing the surface and the core. Despite these early observations, there has been little work on how the different types of acid may attack different parts of the enamel and in particular, the crystallite structure.
To examine whether different acids remove carbonate contaminants from tooth enamel to different extents, this study examined a larger number of teeth from both Holocene and Pleistocene sites with a wider range of acids than Hedges et al. (Reference Hedges, Lee-Thorpe and Tuross1995) and Hopkins et al. (Reference Hopkins, Snoeck and Higham2016) alongside the strong chelating agent EDTA.
METHODS
Samples
In total 10 teeth split into three groups have been studied. The first group contains three teeth from beyond the limit of 14C, providing a sensitive indicator for modern carbon contamination. Five teeth from a late Holocene site demonstrate the effectiveness of the protocol on young materials from a very different depositional environment, and two modern teeth act as standards. All samples are Sus scrofa third molars. Pigs are abundant in South East Asian Pleistocene and Holocene assemblages and their third molars are large, with enough material for multiple dates.
The three Pleistocene teeth (DU795, 798 and 801) are from the site of Duoi U’Oi (Man Duc village, ca. 85 km SW Hanoi), a cave in tower karst in humid subtropical northern Vietnam where an assemblage of faunal remains was recovered from a breccia in 2003 (Bacon et al. Reference Bacon, Demeter, Duringer, Helm, Bano, Vu, Nguyen, Antoine, Bui and Nguyen2008, Reference Bacon, Westaway, Antoine, Duringer, Blin, Demeter, Ponche, Zhao, Barnes and Sayavonkhamdy2015). A speleothem found towards the top of the bone bearing breccia has been dated by U-series to 66 ± 3 ka (Bacon et al. Reference Bacon, Demeter, Duringer, Helm, Bano, Vu, Nguyen, Antoine, Bui and Nguyen2008), and a U-series date on DU795 suggests an age of at least 100 ka (Wood et al. Reference Wood, Duval, Mai Huong, Tuan, Bacon, Demeter, Duringer, Oxenham and Piper2016). As the teeth date beyond the limit of 14C, any 14C present must be a contaminant.
Five teeth from Lo Gach (Table SOM 1), in southern Vietnam provide an indication of the success of pretreatment from a younger and very different depositional environment. Lo Gach is a late-Neolithic to Early Metal Age site on the banks of the Vam Co Tay river. A ca. 1 m cultural deposit was excavated in 2014 by a collaborative team from Long An Provincial Museum, the Southern Institute for Archaeological Research, Ho Chi Minh City, and the School of Archaeology and Anthropology, Australian National University (Piper personal communication 2019). The archaeological site consisted of a series of external deposits, probably associated with a nearby dwelling, that had built up through various processes including the deliberate laying of surfaces, dumping of organic and inorganic refuse and detritus from agricultural (rice processing) and the stalling of animals (Castillo Reference Castillo2019). Together, these processes produced a complex sequence of depositional events and multiple thin strata. No collagen is preserved in the tooth dentine, and comparative ages are not available.
One modern sample (FP) was from a road kill in Corsica, collected defleshed in 2014. The other (BB9) was taken from a pig farm near Byron Bay, Australia, in 2015 and was again collected defleshed. Both died a few years prior to collection and are in the early phases of diagenesis having been weathered on the surface and/or partially buried.
Pretreatment
Following Wood et al. (Reference Wood, Duval, Mai Huong, Tuan, Bacon, Demeter, Duringer, Oxenham and Piper2016) the dentine, surface and material within cracks was carefully removed from enamel with a DremelTM drill with a tungsten carbide drill bit and diamond cutting disk. Dust was removed by repeatedly ultrasonicating in MilliQTM water, before the enamel was crushed by hand in an agate pestle and mortar under MilliQ water. The powder was then ground under MilliQ water in a McCroneTM microniser with agate beads for 30 min (in 5-min intervals to avoid overheating) and freeze-dried.
Initially, two acid leaching protocols similar to Hedges et al. (Reference Hedges, Lee-Thorpe and Tuross1995) and Hopkins et al. (Reference Hopkins, Snoeck and Higham2016) were applied, comparing the effectiveness of acetic acid (VWR HiPerSolv ChromanormTM) and HCl (VWR AnalR NormapurTM) in teeth from Lo Gach and DU801. Subsequently, DU798 was also pretreated with propionic (Sigma AldrichTM) and lactic (Alfa AesarTM) acids to examine a potential link between contaminant removal and pKa. Acid concentration and volume was designed to remove approximately 30% of the enamel, and conditions are listed in Table 2. Most samples were reacted for 20 hr at room temperature under a weak vacuum in a VacutainerTM. To achieve a weak vacuum, the Vacutainer was evacuated to <10–1 Torr prior to the addition of acid. After 1 hr, the acid was frozen in a dry-ice/ethanol slush to protect the vacuum pump, and evacuated to <10–1 Torr. Samples leached in HCl from Lo Gach, were leached for 1 hr after which time the reaction was complete.
Table 2 Summary of pretreatment conditions used in this study. When the pKa of a weak acid equals the pH, 50% of the acid is dissociated. The higher the pKa, the weaker the acid. LogKCa refers to the stability constant with calcium ion (thermodynamic values for 25°C). Higher values indicate stronger binding. pKa and LogKCa values are from Featherstone and Lussi (Reference Featherstone, Lussi and Lussi2006).

Reaction under vacuum was used to speed the rate of reaction. Acid leaching of enamel removes both carbonates (e.g. calcite) and bioapatite producing CO2 gas and dissolved phosphates. The latter can precipitate under some conditions as secondary phosphates, such as brushite. Therefore, reaction under a vacuum may also avoid the inclusion of young carbon from the atmosphere or evolved from the sample, which could be incorporated as trace contaminants in such secondary phosphates.
To test the effect of a strong chelating agent, EDTA was used to clean two teeth, DU795 and DU801. The ability of a molecule to act as a chelating agent is related to the stability constant (K) for the anion-calcium interaction. This is a measure of the strength of interaction, and the stronger the interaction the more likely the anion is to pull the calcium ion from the apatite surface and into solution. EDTA (log KCa 10.7) is a well-known chelating agent that can demineralize bone and enamel at neutral pH (Tuross et al. Reference Tuross, Fogel and Hare1988; Korlević et al. Reference Korlević, Talamo and Meyer2018). Some acids can act in both ways, continuing to demineralize enamel in a neutral pH. For example, lactic acid (log KCa 1.45), can demineralize enamel in a neutral pH more readily than acetic acid (log KCa 1.18) (Featherstone and Lussi Reference Featherstone, Lussi and Lussi2006).
Samples were ultrasonicated in 1 mL of MilliQ water for 1 hr to break aggregates, and 1 mL/100 mg enamel of 0.1 M EDTA (Sigma AldrichTM, ACS) (at pH9) was added, resulting in 2 mL of 0.05 M EDTA/100 mg enamel. Samples were reacted for 10 min, before being rinsed in MilliQ water. As CO2 is soluble in a basic solution, the samples were subsequently treated with acetic acid under vacuum for 20 hr. Again, the pretreatment conditions were designed to remove around 30% of the initial enamel weight. In an experiment on modern enamel, the EDTA treatment removed around 10% of the sample and the acetic acid around 20%.
Radiocarbon Dating
The routine S-ANU laboratory protocol for carbonates includes freeze-drying of the cleaned sample, before reaction with phosphoric acid (85%, 80ºC, 2 hr) in a VacutainerTM previously evacuated to less than 3 x 10–3 Torr. The CO2 generated is cryogenically collected and reacted with hydrogen over an iron catalyst to produce graphite, prior to measurement in an NEC Single Stage AMS (Fallon et al. Reference Fallon, Fifield and Chappell2010). Dates are calculated according to Stuiver and Polach (Reference Stuiver and Polach1977) using an AMS derived δ13C.
However, we have noticed that it often takes more than 6 hr to graphitize the CO2 generated from reaction of enamel with phosphoric acid. This is presumably due to the presence of sulphur dioxide, although it is unclear why this should be present in enamel. We therefore followed protocols common when dating cremated bone which can contain high concentrations of sulphur dioxide (Naysmith et al. Reference Naysmith, Scott, Cook, Heinemeier, van der Plicht, van Strydonck, Ramsey, Grootes and Freeman2007). After reaction with phosphoric acid, the CO2 was cryogenically collected into a quartz ampule evacuated to less than 3 × 10–3 Torr, and heated to 900ºC for 6 hr in the presence of silver foil and copper oxide wire prior to graphitsation. Dates on enamel from S-ANU 54306 onwards have been treated with this acidification-combustion method. This extra step introduces slightly more laboratory derived CO2 than the routine protocol for carbonates, and the background calculation is described in the supplementary online material (SOM 2.1). Dates on enamel with S-ANU 54305 or earlier have had the routine laboratory background subtracted based on the long-term average of dates on IAEA-C1 graphitized without the precombustion step.
Given the exceptionally large surface area of the micronized samples, it was thought prudent to check whether enough atmospheric CO2 could adsorb to the enamel surface between freeze-drying and reaction with phosphoric acid to affect the 14C age. DU798 was exposed to air for up to 3 weeks, but contamination from atmospheric CO2 was not observed (SOM 2.2). As a precaution, acid cleaned samples were exposed to atmosphere for ≤1 hr before graphitization in this study. Where samples were not exposed to atmosphere, yield information is not available.
FTIR
FTIR was undertaken to assess preservation of the original enamel structure and identify secondary phosphates and carbonates. Methodology details are given in the supplementary online material (SOM 3.1). As a likely carbonate contaminant, calcite presence was assessed using a band at 711 cm–1 (Lee-Thorp and van der Merwe Reference Lee-Thorp and van der Merwe1991). When enamel is left in a weakly acidic solution, brushite (CaHPO42H2O) can form, with amounts increasing as reaction time is extended (Lee-Thorp and van der Merwe Reference Lee-Thorp and van der Merwe1991). Reaction was carried out under a weak vacuum and brushite will only contain carbon as an impurity, meaning the 14C dates and stable isotope analyses should not be greatly affected. However, %C measurements could be affected. Brushite was primarily identified by a band at –525 cm–1 resulting from a ν4 O-P-O deformation. A sharp band at –1645 cm–1 resulting from the ν2 water H-O-H deformation was seen in samples containing the most brushite, and a band at –792 cm–1 resulting from a γOH out of plane P-O-H deformation was used to identify smaller quantities of brushite (Berry and Baddiel Reference Berry and Baddiel1967; Petrov et al. Reference Petrov, Šoptrajanov, Fuson and Lawson1967; Lee-Thorp and van der Merwe Reference Lee-Thorp and van der Merwe1991).
A variety of indices derived from FTIR spectra are widely used amongst the stable isotope community to assess enamel preservation and thus argue for the integrity of the stable isotope data (Table SOM 3) (Roche et al. Reference Roche, Ségalen, Balan and Delattre2010; Roberts et al. Reference Roberts, Stewart, Alagaili, Breeze, Candy, Drake, Groucutt, Scerri, Lee-Thorp and Louys2018). The Infra Red Splitting Factor (IRSF) gives information about crystal order, a combination of crystal size and level of impurities. BPI and API give information on the amount of carbonate in the B (substituting for the phosphate ion) and A (substituting for the hydroxyl ion) positions in the hydroxyapatite lattice.
Bandwidth increases with increasing grain size (Ruppin and Englman Reference Ruppin and Englman1970), strongly affecting indices (Surovell and Stiner Reference Surovell and Stiner2001) making it difficult to compare the untreated samples which were hand-ground with the micronized and leached samples. It is however possible to compare within the micronized samples. One sample (LGa923HG) has been analyzed 3 times. Uncertainties are <0.1, <0.02, and <0.01 for IRSF, BPI and API respectively for indices derived from both the grinding curve and individual KBr pellet methods.
IRMS
%C, δ13C, and δ18O analyses were undertaken either on a Gas Bench connected to a Sercon 20-22 isotope ratio mass spectrometer operating in continuous flow mode, or on a Thermofisher Delta Advantage mass spectrometer coupled to a Kiel IV carbonate device.
On the Sercon 20-22 instrument, approximately 22 mg enamel was reacted with 0.5 mL 99% H3PO4 at 80ºC to generate CO2. Samples were measured against an in-house calcium carbonate standard and normalized to Vienna Peedee Belemnite scale (VPDB) using NBS18, LSVEC and an in-house standard P3. %C was calculated from beam area.
On the Thermofisher instrument results have been normalized with the NBS-19 and NBS-18 carbonate standards. The composition of the reference gas used during analysis is δ13C –20.620‰ VPDB and δ18O –3.450‰ VPDB. Samples were reacted with 105% H3PO4 at 75ºC to produce CO2. The pressure of the purified CO2 gas in the Kiel device (MV1) is related to the weight of the carbonate in the sample/standard in a linear fashion. This was used to calculate an estimate of the %C in the samples by feeding their MV1 into the linear correlation obtained using the weight and MV1 pressure of the carbonate standards (from the same and previous day’s run). Since the correlation is not perfectly linear and can vary with the conditions of the run, the systematic error associated to this calculation can be up to 20% in %CaCO3 and therefore %C is used as an estimate.
1-sigma random uncertainties, calculated as the standard deviation of repeat measurements of tooth enamel, were typically 0.01%, 0.1‰, and 0.2‰ for %C, δ13C, and δ18O, respectively on the Sercon 20-22 instrument, and 0.01%, <0.1‰ and 0.2‰ for %C, δ13C, and δ18O, respectively on the Thermofisher instrument.
The low number of samples available for each tooth limits the statistical analysis possible, so only broad trends have been identified within the data.
RESULTS AND DISCUSSION
Preservation of Tooth Enamel
FTIR analysis of the enamel shows that calcite is not present in any sample (Table 3). Although the hydroxyapatite appears relatively well preserved when compared with modern pigs, small differences are seen between the modern and ancient pig teeth in this study. Unexpectedly, the IRSF of the ancient enamel (ranging between 3.8–4.0) is lower than the modern pigs (ranging between 4.1–4.3) suggesting lower crystal order. The reverse is expected from a recrystallization process which is likely to result in larger crystals with less carbonate (Asscher et al. Reference Asscher, Regev, Weiner and Boaretto2011). %C is generally higher in the ancient teeth (ranging 0.69–0.85 %C) than in the modern teeth (ranging 0.67–0.72 %C), perhaps in part explaining the lower crystal order of the ancient samples.
Table 3 Results of the 14C, stable isotope and FTIR analyses on samples pretreated with different acid protocols. 1 σ random uncertainties are listed. Given potential large systematic uncertainties in %C, these values should only be used as estimates. Protocol 0 represents no treatment, 1. hydrochloric acid, 2. lactic acid, 3. acetic acid, 4. propionic acid, and 5. acetic acid and EDTA (Table 2). BPI refers to the B-carbonate on phosphate index, and API the A-carbonate on phosphate index (Table SOM 3).

s – Small peak around –792 cm–1, but no shoulder or band at –525 cm–1. y – Shoulder or band at –525 cm–1. May also have a sharp band at –1645 cm–1. n – No brushite observed. * – FTIR indices are derived from a grinding curve.
API and BPI are both generally higher in the ancient samples (0.12–0.18 and 0.27–0.39 respectively) than the modern teeth (0.10–0.11 and 0.28–0.30). This contrasts to Roche et al. (Reference Roche, Ségalen, Balan and Delattre2010) and Roberts et al. (Reference Roberts, Perera, Wedage, Deraniyagala, Perera, Eregama, Petraglia and Lee-Thorp2017) who both found that BPI did not increase during burial, whilst API did. However, the ratio between these (API/BPI) is slightly higher in the ancient teeth (ranging 0.40–0.47) than the modern teeth (0.33–0.40) suggesting a larger amount of A-type carbon relative to the B-type carbon, in line with Roche et al. (Reference Roche, Ségalen, Balan and Delattre2010) and Roberts et al. (Reference Roberts, Perera, Wedage, Deraniyagala, Perera, Eregama, Petraglia and Lee-Thorp2017).
Currently, it is unclear why the ancient samples contain more carbonate within the apatite lattice, and why the location of this carbonate within the crystal structure seems to vary in comparison with previous studies. Whilst likely diagenetic, it may also reflect the small sample size and substantial natural variation. For example, carbonate content decreases during tooth formation (Sydney-Zax et al. Reference Sydney-Zax, Mayer and Deutsch1991), between permanent and deciduous teeth (Sønju Clasen and Ruyter Reference Sønju Clasen and Ruyter1997) and across the enamel thickness (Xu et al. Reference Xu, Reed, Gorski, Wang and Walker2012).
Pretreatment
% yield ranged between 60 and 80%, with an average of 66 ± 3% surviving the acetic acid leach, and 73 ± 2% surviving the HCl treatment. Brushite was sporadically observed in the pretreated apatite, and was caused by both weak and strong acids (Table 3).
Effect of Leaching on Radiocarbon Dating
The Pleistocene Duoi U’Oi samples are heavily contaminated, as previously found (Wood et al. Reference Wood, Duval, Mai Huong, Tuan, Bacon, Demeter, Duringer, Oxenham and Piper2016) (Table 3). If we assume that the contaminant is modern in age, nearly 15% of the carbon in the carbonate fraction of both DU795 and DU798 must be a contaminant. The actual contaminant load is likely to be much higher as the contaminant is probably several thousand 14C years old due to dissolved limestone in the breccia water.
All Duoi U’Oi samples produced markedly different results between HCl (protocol 1) and acetic acid (protocol 3) treatments (Figure 1), and the effect of pretreatment was very consistent when replicated on DU801, despite the large contaminant load. After HCl treatment, nearly 8% modern carbon remained, but this was more than halved when acetic acid was used. In terms of BP, ages improved from around 20 kBP to 27 kBP between HCl and acetic acid treatments. Our HCl acid leach removed slightly less material than the acetic acid (27± 2% and 34 ± 3% respectively). Unfortunately, the enamel samples were small and did not allow for a repeat of the experiment. However, the small difference in yield between the two pretreatments is unlikely to have caused such a large difference in F14C. Brushite was not observed in the enamel leached with HCl from Duoi U’Oi and could not have affected the results.

Figure 1 The effect of the type of acid used in pretreatment on F14C for (a) samples known to date beyond the limit of 14C from Duoi U’Oi and (b) samples of ca. 2000 cal BC from Lo Gach. 0 represents no treatment, 1. hydrochloric acid, 2. lactic acid, 3. acetic acid, 4. propionic acid, and 5. acetic acid and EDTA.
At Lo Gach, substantial amounts of brushite were present in all HCl leached samples. Whilst one sample, LGa786, sees little difference in age between the two treatments (an increase of just 9 ± 37 14C years between acetic acid and HCl), the remaining four samples saw the age increase after treatment with acetic acid in comparison with hydrochloric acid. However, the increase was only significant for LGa935 at 95 % probability (X2, df 1, T=7.4 (5% 3.8)). This sample also has the greatest reduction in F14C between unleached and leached samples, suggesting it is the most contaminated and therefore the most likely to be affected by different leaching protocols. Indeed, it would not be possible to distinguish between samples similar in age to those at Lo Gach with 6% and 4% modern carbon contamination. It is likely that the less pronounced trends at Lo Gach reflect the reduced sensitivity to contamination in younger periods. However, the consistency of the decrease in F14C between HCl and acetic acid leaches at Lo Gach supports the pattern seen in the much older and more sensitive samples from Duoi U’Oi.
To examine why these differences occurred, one Pleistocene sample was also subjected to lactic acid (protocol 2) and propionic acid (protocol 4) to cover a range of pKa’s. With increasing pKa the age appears to increase, with lactic acid producing an age mid-way between the sample treated with HCl and the sample treated with acetic acid. Propionic acid produced a similar, though slightly younger age, to acetic acid. This implies a link between acid strength and removal of carbonate contaminant.
The combined acetic acid-EDTA treatment (protocol 5) gave an age indistinguishable from that produced by the acetic acid treatment for tooth DU801 and slightly younger for tooth DU795. It is likely that EDTA does not remove more contamination than the acetic acid alone.
Effect of Acid Leaching on Stable Isotopes
Phases that are enriched in carbonate are preferentially removed by all pretreatments, as %C tends to decrease during pretreatment (Table 3, Figure 2a–c). These carbon rich phases also contain a higher contaminant load, as at Duoi U’Oi %C increases with F14C in all three teeth (Figure 3a). The presence of large amounts of brushite in the teeth from Lo Gach leached in HCl hinders interpretation of %C.

Figure 2 The effect of the type of acid used in pretreatment on (a–c) %C, (d–f) δ13C, (g–i) δ18O, for teeth from Duoi U’Oi (a, d, g), Lo Gach (b, e, h) and modern teeth (c, f, i). 0 represents no treatment, 1. hydrochloric acid, 2. lactic acid, 3. acetic acid, 4. propionic acid, and 5. acetic acid and EDTA.

Figure 3 Comparison of (a–b) %C, (c–d) δ13C, (e–f) δ18O with F14C for teeth from Duoi U’Oi (a, c, e) and Lo Gach (b, d, f). For legend, please refer to Figure 2.
δ13C also appears related to F14C in enamel from both Duoi U’Oi and Lo Gach (Figure 3c–d), with offsets between the untreated and leached samples being more than 2‰ in DU801 and LGa935. Correlation analyses for samples with just 3 data points are difficult to analyze statistically, and so a relationship is implied by all teeth showing increasing δ13C with F14C, rather than assessed with e.g. Pearsons Correlation Coefficient. Treatments that are more efficient at removing 14C contamination, also remove carbonate higher in δ13C.
Although several studies have noticed changes in the δ13C of modern enamel leached in acetic acid (Koch et al. Reference Koch, Tuross and Fogel1997; Pellegrini and Snoeck Reference Pellegrini and Snoeck2016), this change is often much smaller. For example, Koch et al. (Reference Koch, Tuross and Fogel1997) found a decrease of just 0.27 ± 0.09‰ between untreated enamel and enamel leached in 1M acetic acid after a bleaching step. There is also only a little variation in the two modern pig molars examined here, with FP values varying by 0.3‰ (with an analytical uncertainty of <0.1‰) and BB9 by 0.4‰ (with an analytical uncertainty of 0.1‰) (Figure 2f). Moreover, this variation is not systematic with some treatments giving values more enriched in 13C and others giving values depleted in 13C compared to the untreated sample, and variation may just imply inhomogeneity within the sample.
δ18O shows little systematic change between leached and unleached enamel and does not appear related to F14C (Figures 2g–i and 3e–f). This is probably due to fractionation during the long acid leach (Balter et al. Reference Balter, Saliège, Bocherens and Person2002).
Effect of Acid Leaching on Enamel Structure; FTIR
Detailed analysis of FTIR parameters is complicated by the variable bandwidth between the handground unleached samples and the micronized leached samples. For example, IRSF tends to be higher in the leached samples (Figure SOM 2a–c), although this trend would be expected based on the smaller grain size of the leached samples. Overall, there seems little relationship between F14C and IRSF within the leached samples (Figure SOM 3a–b).
As may be expected from the reduction in %C after pretreatment both BPI and API decrease during pretreatment (Figure SOM 2d–i), and a positive relationship between F14C and BPI is particularly clear for the teeth from Lo Gach (Figure SOM 3d). Indeed, the relationship between BPI or API and F14C is clearer than for %C at this site, probably because of the presence of brushite in the enamel leached with HCl. Both BPI and API indices were elevated in the untreated ancient teeth in comparison to the modern teeth (Figure SOM 2g–l), and both decrease to approximately the same value as the modern teeth treated in the same way. However, although API/BPI was also elevated in the ancient untreated teeth (Figure SOM j–l), pretreatment does not consistently reduce the ratio towards the values seen in the modern teeth, and there seems little relationship between API/BPI and F14C at either Duoi U’Oi or Lo Gach (Figure SOM 2g–h). Therefore, acids with a higher pKa generally remove more carbon from ancient teeth than those with a lower pKa, but this does not seem to be specific to either B or A type carbonate environments.
CONCLUSION
The type of acid used to remove contamination from tooth enamel appears to have a significant effect on the 14C date obtained. In contrast to Hopkins et al. (Reference Hopkins, Snoeck and Higham2016), but in agreement with Hedges et al (Reference Hedges, Lee-Thorpe and Tuross1995), we find that weaker acids (with higher pKa’s) remove more contamination than stronger acids. This effect is consistent across two sites containing teeth likely to have had different diagenetic histories. Brushite is sporadically formed during leaching in both weak and strong acids. Unexpectedly, HCl treatment appeared to cause larger quantities of brushite to form more regularly than acetic acid.
Despite the presence of brushite in some samples and not others, the relationship between δ13C and F14C is maintained in all teeth presumably because brushite only contains a small amount carbonate as an impurity. This relationship either implies that contamination causes both the δ13C and F14C to be shifted to higher values, or that contaminants are located in the same location in the enamel structure as endogenous carbonate with higher δ13C values (Koch et al. Reference Koch, Tuross and Fogel1997), and both are removed at the same rate by different acid leaching treatments. These possibilities are not exclusive of each other. However, it is clear from this study (alongside August et al. in prep.) that young carbonate contaminants are also present in the ancient teeth and it is likely that these have a different isotopic signature to those of the enamel. It would seem implausible that the shift seen in the δ13C of ancient tooth enamel is not related to contamination to some degree.
The reason weaker acids remove more contaminants than stronger acids is unclear from the rather coarse FTIR data obtained here. A number of non-exclusive possibilities exist.
-
1. The nanoparticles produced by mechanical grinding are electrostatic and prone to form aggregates. This could hinder contaminant removal as surfaces of crystals at the center of the aggregate may be protected during acid leaching. Although the aggregates are not easily broken apart by vortex mixing or ultrasonication, they can be disrupted by chelating agents which bond strongly to the surface of the crystal (Corrêa de Araujo et al. Reference Corrêa de Araujo, Wesley Poling, de Magalhães Viana and Zhang2010). Few visible aggregates were observed when EDTA was used in this study, but we see no improvement between the EDTA and acetic acid treatments. Therefore, formation of aggregates may not play a major role in the unsuccessful removal of contamination.
-
2. It is possible that physical or chemical mechanisms control which part of the enamel structure and what kind of carbonate the acid reacts with. If it is assumed the majority of contamination is located at the surface of the prisms and crystallites and/or in the amorphous phase (Wood et al. Reference Wood, Duval, Mai Huong, Tuan, Bacon, Demeter, Duringer, Oxenham and Piper2016), the large bubble generated by exceptionally vigorous and fast reaction of the strong acids may hinder access of the hydrogen ion into the pores. The slower and continuous reaction of the weaker acids over the full 20 hr may allow a more even reaction over the surface and some reaction with the surface of the pores.
-
3. Different acids may preferentially react with different phases within the tooth enamel. Acid preferentially attacks apatite rich in carbonate with %C, API and BPI all decreasing during acid leaching and with F14C. However, it is not clear that enamel richer in the A type carbonate, which Roche et al. (Reference Roche, Ségalen, Balan and Delattre2010) and Roberts et al. (Reference Roberts, Perera, Wedage, Deraniyagala, Perera, Eregama, Petraglia and Lee-Thorp2017) propose may be diagenetic, is attacked preferentially. Alternatively, the weaker acids may preferentially react with the more soluble amorphous phase which is present between the hydroxyapatite crystallites (Gordon et al. Reference Gordon, Cohen, MacRenaris, Pasteris, Seda and Joester2015), whilst the stronger acids are able to rapidly react with the hydroxyapatite. The standard FTIR indices only examine the relative proportion of carbonate within the A and B positions in apatite, and it is unlikely that this would be affected by such a mechanism. However, we would expect a difference in carbonate in surface positions or within the amorphous phase. So called “labile” carbonate has been observed in FTIR spectra of bone and enamel, for example at around 866 cm–1 (Rey et al. Reference Rey, Collins, Goehl, Dickson and Glimcher1989, Reference Rey, Renugopalakrishnan, Shimizu, Collins and Glimcher1991). Unfortunately, we were unable to produce consistent results by peak deconvolution in the very low intensity carbonate band in this study.
Although the reason for the differences between the acids remains uncertain, it does appear from this dataset that hydrochloric acid should not be used to leach tooth enamel for either 14C or stable carbon isotope analysis, and acetic acid should be favored, as normally employed. Regardless of these pretreatment protocols, significant contamination still resides in ancient tooth enamel from sub-tropical environments resulting in an underestimate of the age of the tooth.
ACKNOWLEDGMENTS
The research and R. Wood were funded by an Australian Research Council DECRA fellowship (DE150100070). Infrared spectral analyses were supported by Australian Research Council grants to Penelope L King (DP15014606 and FT130101524) and ANU Major Equipment Committee grants to Jörg Hermann and John Mavrogenes. Jaime Swift, Matthew Cupper, Rainer Grun, Malte Willmes, and Hannah James are thanked for providing the modern pig teeth. Julien Louys is thanked for suggesting Vietnamese pigs as a possible sample type. Philip Piper is thanked for permission to use teeth from Lo Gach, and provision of unpublished contextual information. The excavations at Lo Gach were funded through an ANU College of Arts and Social Sciences Small Grant awarded to Philip Piper. Anne-Marie Bacon, Fabrice Demeter and Philippe Duringer are thanked for providing information on the geological context of Duoi U’Oi. The excavation of Duoi U’Oi was financed by the CNRS (Centre National de la Recherche Scientifique), the Collège de France, France, and the Tohoku University School of Medecine, Sendai, Japan. Charles Le Soq is thanked for attempts to deconvolute the FTIR spectra. Laura Rodriguez is thanked for stable isotope analysis of the tooth enamel on the Thermofisher instrument. Rebecca Esmay of the ANU radiocarbon facility is thanked for discussions about tooth enamel and acids.
Supplementary material
To view supplementary material for this article, please visit https://doi.org/10.1017/RDC.2021.32