INTRODUCTION
Iceland is an emerged, slow-spreading part of the Atlantic oceanic ridge–rift system, under the control of a hot spot underlying two different plates that host overlapping volcanic zones, both subject to plate tension. Iceland has also been covered, completely or partially, by ice since the Pliocene (3 Ma), having had a large ice cap since 2.2 Ma (Geirsdottir and Eiriksson, Reference Geirsdottir and Eirıksson1994).
Most of the tectonic and seismic activity in Iceland is connected via fissure systems and is generated by hot-spot volcanism with correlation between volcanic activity in the East Volcanic Zone (EVZ) and Holocene seismic activity in the western South Iceland Seismic Zone (SISZ; Larsen et al., Reference Larsen, Guðmundsson and Björnsson1998). More specifically, during the Holocene, the SISZ (Fig. 1C) accommodated left-lateral transform movement between the West Volcanic Zone (WVZ) and the more active EVZ (Fig. 1). This transform zone included N–S right-lateral strike-slip faults that largely controlled seismicity during the Holocene (Einarsson and Eiríksson, Reference Einarsson and Eiriksson1982; Stefánsson et al., Reference Stefánsson, Bödvarsson, Slunga, Einarsson, Jakobsdottir, Bungum, Gregersen, Havskov, Hjelme and Korhonen1993).
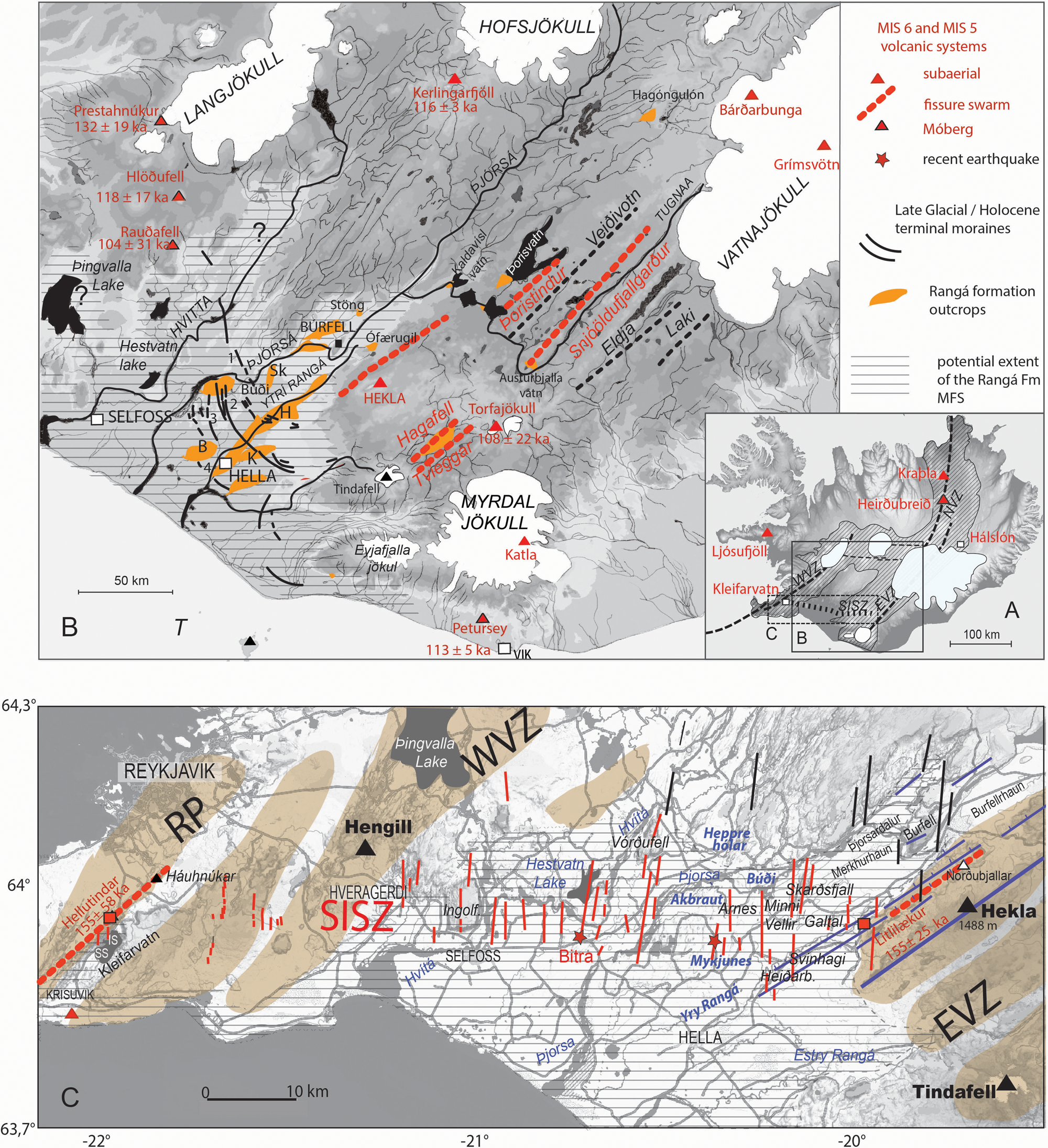
Figure 1. Location of the investigated sites: (A) general view and (B) extent of the interglacial formation. Morainic arc complexes: 1, Finse-Búði; 2, Mykjunes; 3, Litlatunga; 4, Sόlvellir. Investigated sites: B, Bolstaður; K, Kirkjubær; H, Heiðarbrekka; Sk, Skarðsfjall; Stöng, archaeological site; O, Ofærugill. WVZ, West Volcanic Zone; EVZ, East Volcanic Zone; NVZ, North Volcanic Zone; SISZ, South Iceland Seismic Zone. (C) SISZ in relation to the interglacial formation estuaries and volcanic systems (in khaki). Names of late-glacial morainic arcs given in blue. IS, Innristapi; SS, Syðristapi; RP, Reykjanes Peninsula. White triangles, tuyas; Red stars, AD 2000 twinned earthquakes (modified and completed from http://www.norvol.hi.is/~thora/olfus.html). Note the Hekla flexural zone in violet. SISZ: historical and present-day active faults in red, inactive in black. For the ages (in red) see Table 3, Supplementary material (For interpretation of the references to color in this figure legend, the reader is referred to the web version of this article.)
Global volcanism seems to be influenced by Milankovitch cycles (Kutteroff et al., Reference Kutterolf, Jegen, Mitrovica, Kwasnitschka, Freundt and Huybers2013). The 1.8 and 5 ka tidal cyclicities of the solar system that induce some deformations in the lithosphere also modulate the climate in synchronicity with the warming leading to Dansgaard-Oeschger (DO) ice-rafted debris (IRD) cycles (Keeling and Whorf, Reference Keeling and Whorf2000). It is therefore not surprising to find a reasonable correlation in Iceland between the warmings and tectonic events that control the volcanic activity. Little is known concerning the timing of volcanic activity during ice-sheet building. Most subglacial activity is commonly attributed to the last glacial maximum (LGM) or its deglaciation (Walker, Reference Walker1965; Licciardi et al., Reference Licciardi, Kurz and Curtice2007; Eason et al., Reference Eason, Sinton, Grönvold and Kurz2015), which conflicts with the Plio-Pleistocene glacial history of the island (Geirsdottir and Eiriksson, Reference Geirsdottir and Eirıksson1994).
Based on the Reykjanes Peninsula postglacial volcanism study, Guðmundsson (Reference Guðmundsson1986) suggested that the rapid uplift and bending of the crust above the magma reservoirs, during the glacial unloading, favor the formation of ridges and shield volcanoes. Since that time, the mechanical effects of deglaciation on volcanism and the connection between glacial loading/unloading and volcanic activity have been discussed, analyzed, or modeled by many (Jull and McKenzie, Reference Jull and McKenzie1996; Slater et al., Reference Slater, Jull, McKenzie and Grönvold1998; Zielinksi, Reference Zielinksi2000; Maclennan et al., Reference Maclennan, Jull, McKenzie, Slater and Grönvold2002, Sinton et al., Reference Sinton, Grönvold and Sæmundsson2005; Andrew and Guðmundsson, Reference Andrew and Guðmundsson2007; Huybers and Langmuir, Reference Huybers and Langmuir2009; Sturkell et al., Reference Sturkell, Einarsson, Sigmundsson, Hreinsdóttir and Geirsson2003; Praetorius et al., Reference Praetorius, Mix, Jensen, Froese, Milne, Wolhowe, Addison and Prahl2016). Glacial unloading is thought to have been responsible for an increase in magma melting rates below the base of the crust, due to enhanced mantle decompression (Maclennan et al., Reference Maclennan, Jull, McKenzie, Slater and Grönvold2002; Sinton et al., Reference Sinton, Grönvold and Sæmundsson2005), in combination with unloading by subglacial erosion (Sternai et al., Reference Sternai, Caricchi, Castelltort and Champagnac2016). This resulted in a significant percentage of global volcanic eruptions during the early stages of deglaciation (Watt et al., Reference Watt, Pyle and Mather2013; Rawson et al., Reference Rawson, Pyle, Mather, Smith, Fontijn, Lachowycz and Naranjo2016), between ~13 ka and 11 ka, followed by an intensification at 10.3 ka, expressed by the Saksunarvatn eruption (Grímsvötn; Zielinksi, Reference Zielinksi2000; Eason et al., Reference Eason, Sinton, Grönvold and Kurz2015). The return to normal occurred progressively after 10.3 ka (Sinton et al., Reference Sinton, Grönvold and Sæmundsson2005; Eason et al., Reference Eason, Sinton, Grönvold and Kurz2015).
Unconsolidated deposits from the last deglaciation in southern Iceland (Fig. 1B) have been well studied (Van Vliet-Lanoë et al., Reference Van Vliet-Lanoë, Schneider, Guðmundsson, Guillou, Nomade, Chazot, Liorziou and Guégan2018), and the sedimentary deposits, with evidence of volcanic activities, are commonly faulted and have convoluted bedding of possible co-seismic origin,. Very few sequences recorded the last interglacial near 60°N latitude. The Rangá Formation, in southern Iceland (63°–64°N; Van Vliet-Lanoë et al., Reference Van Vliet-Lanoë, Schneider, Guðmundsson, Guillou, Nomade, Chazot, Liorziou and Guégan2018), is an extended sequence, including an estuarine Marine Isotopic Stage 5e (MIS 5e) complex preserved below the well-studied deposits of the last deglaciation. This long record crosses the SISZ and thus could provide information to link the ice-sheet dynamics, the underlying geodynamics, and the volcanic activity. In particular, it includes potentially datable lava and tephra. The complete sedimentary sequence has been fully described, and its significance, in terms of paleoclimate, has been discussed (Van Vliet-Lanoë et al., Reference Van Vliet-Lanoë, Schneider, Guðmundsson, Guillou, Nomade, Chazot, Liorziou and Guégan2018). As the SISZ is the most active onshore seismic zone in Iceland, it is the best place to determine any changes in seismicity or tectonic style following the strain imposed by glacial loading/unloading and any associated hydrological changes.
We consider, in this paper, the lastest and prior deglaciations as having temporarily enhanced volcanism. The aim of this work was, first, to attempt to understand from this extended sedimentary record the relationship between glaciations and volcano-seismo-tectonic activity along the SISZ (Fig. 1C). In this study, we focused on the 160–155 ka (MIS 6b) deglaciation, the Termination IIb (132 ka), the deglacial event (116 ka), the GI 25 of the Greenland ice cores, and Terminations Ia and Ib (14.5 and 12 ka, respectively). To understand the specific impacts of glacial loading, we examined the connection between the characteristics and the ages of the subglacial volcanoes (tuyas, or hyaloclastite ridges), from volcanic zones, using radiometric dating, facies, and the tephrochronological record from marine and glacial cores.
METHODS
Sedimentological studies in the Ytrí Rangá valley, and on surrounding volcanoes, were conducted from 2004 to 2018; the Lake Kleifarvatn system was analyzed in 2009 through 2017. Our observations are based on detailed mapping and section descriptions (see Van Vliet-Lanoë et al., Reference Van Vliet-Lanoë, Schneider, Guðmundsson, Guillou, Nomade, Chazot, Liorziou and Guégan2018, Supplement 1, and site locations in Table 1 of the Supplementary material). The station locations were obtained by Global Positioning System measurements, which were compared with the revised topographical map of Iceland (http://kortasja.lmi.is, 2014 and 2018), giving an average vertical error of ± 5 m. Tectonic studies of Quaternary to present-day faulting, based on both field studies and the focal mechanisms of earthquake analysis, were conducted in the EVZ and SISZ from 1995 to 2016. High-resolution aerial views and digital elevation models (DEMs) were produced using a drone (a Naza hexacopter). This allowed stereoscopic overlapping to construct a high-resolution and interactive DEM using the MicMac computer program specifically developed at Lyon I University (Pierrot-Deseilligny and Paparoditis, Reference Pierrot-Deseilligny and Paparoditis2006, see Supplementary Material). As a result, we expected to obtain DEMs and Orho-images at a resolution of better than 3 cm.
Petrographic analyses (thin sections, X-rays, scanning electron microscopy, geochemistry, inductively coupled plasma mass spectrometry (ICPMS AEL) were performed using the procedure outlined by Cotten et al. Reference Cotten, Le Dez, Bau, Caroff, Maury, Dulski and Brousse1995). Trace element concentrations on tephra and lava were also measured with a Thermo Element2 HR-ICP-MS in Brest (France), after repeated HF-HClO4 digestion and HNO3 dilutions (for details, see Li and Lee, Reference Li and Lee2006). Major elements were analyzed using the Brest CAMECA CAMEBAX SX100 electron microprobe with a specific protocol (see Supplementary Material, p. 11).
All the used K-Ar and 40Ar/39Ar dating are taken from previously published data (see Supplementary material, Table 3) produced using the methodology established by Guillou et al. (Reference Guillou, Van Vliet-Lanoë, Guðmundsson and Nomade2010, Reference Guillou, Scao, Nomade, Van Vliet-Lanoë, Liorzou and Guðmundsson2019). Reliable data for the Galalaekur lava were not available, so U-Th dating was performed on lavas from the Rangá valley at BRGM Orléans on a Neptune MC-ICPMS. Details on chemical separation and mass spectrometry can be found elsewhere (Innocent et al., Reference Innocent, Fléhoc and Lemeille2005; Millot et al., Reference Millot, Guerrot, Innocent, Négrel and Sanjuan2011). A reference age of 116 ka was derived, although no well-defined isochron could be obtained. Calculations were done using the method of Minster et al. (Reference Minster, Ricard and Allègre1979; see Supplementary Material, p. 8).
An attribution of the Rangá Formation to the MIS 5 sensu lato (MIS 5e and 5c, Member C) was measured using a uranium isostope desiquilibrium methodology (see Supplementary Material, p. 7), yielding an age of 116 ka on lava interstratified in the C3 unit. The radiometric ages (K–Ar ages) of the same units in Snafelness (132 and 129 ka) and in the Southwestern Volcanic Zone confirm this attribution (see Van Vliet-Lanoë et al., Reference Van Vliet-Lanoë, Schneider, Guðmundsson, Guillou, Nomade, Chazot, Liorziou and Guégan2018). It has also been confirmed by the tephrostratigraphic signature of the outcrops, which is consistent with the available regional data from marine and ice records (Davies et al., Reference Davies, Abbott, Meara, Pearce, Austin, Chapman, Svensson, Bigler, Rasmussen and Farmer2014). The timing of the Grímsvötn, Bárðarbunga, and Hekla volcanic eruptions from MIS 5e to the present was compiled from a published, synthesized tephrostratigraphic series (Óladóttir et al., Reference Óladóttir, Larsen and Sigmarsson2011; Davies et al., Reference Davies, Abbott, Meara, Pearce, Austin, Chapman, Svensson, Bigler, Rasmussen and Farmer2014; Voelker and Haflidason, Reference Voelker and Haflidason2015). As a complement, we report in the Table 3 of the Supplementary Material the available K–Ar and 40Ar/39Ar ages spanning the same interval and performed on subglacial (tuyas) and subaerial lavas. These ages are crucial for constraining the dynamics of the last large Icelandic ice sheet interacting with volcanic activity.
Regional geological setting of the extended sedimentary record
South-central Iceland is a wide depression, delimited by two volcano-tectonic zones—the WVZ to the west and the more active EVZ to the east (Fig. 1A). The EVZ is the youngest part of the Icelandic rift, and has been active since 2–3 Ma (Johannesson et al., Reference Johannesson, Sæmundsson and Jakobsson1990). It includes, among others, the Katla, Torfajökull, Hekla, and Tindafell Volcanoes. This depression is one of the main outlets for the Icelandic glaciers. The central plain is formed by a complex sandur, related to the Ytrí Rangá, Þjórsá, and Hvítá Rivers (Fig. 1B). It was regularly scoured by late-glacial/Holocene jökluhlaups—or flash floods—that were often triggered by the subglacial volcanic activity (Björnsson, Reference Björnsson2002). Late-glacial and Holocene lava flows also partly protected the old sediments, allowing for good outcrop exposures.
Overview
The south-central Icelandic depression is characterized by the presence of interstratified basalts and glacial and hyaloclastite deposits, ranging in age from early Quaternary to Holocene (Johanesson and Sædmundsson Reference Johanesson and Sædmundsson1998; Kristjánsson et al., Reference Kristjánsson, Duncan and Guðmundsson1998; Fig. 1B). Regional volcanic activity increased during the last deglaciation, especially for the Grímsvötn, Bárdarbunga, and Katla Volcanoes, with Hekla apparently showing activity later, close to 6060 cal yr BP (Larsen et al., Reference Larsen, Eiríksson, Knudsen and Heinemeier2002; Guðmundsdottir et al., 2016). The most widespread early Holocene lava inside the central zone is the Þjórsá Lava flow field (Fig. 1C), dated ca. 8.6 ka (Hjartarson and Ingólfsson, Reference Hjartarson and Ingólfsson1988; Halldorsson et al., Reference Halldorsson, Oskarsson, Grönvold, Sigurdsson, Sverrisdóttir and Steinthorsson2008), which issued from the Veiðivötn fault system (Bárðarbunga volcanic system; Fig. 1B). These lava flows capped most of the older sediments, previously considered to be Holocene in age (Einarsson, Reference Einarsson1994) and also postdate the Búði Preboreal moraine system (11.2 ka: Geirsdóttir et al., Reference Geirsdóttir, Hardardóttir and Sveinbjörnsdóttir2000; Fig. 1B).
South Iceland Seismic Zone
Southern Iceland is crossed from west to east by the SISZ (Fig. 1C), a transform zone that extends inland from the Reykjanes Peninsula (Fig. 1C), which represents the extension of the present-day Reykjanes Ridge (analyzed at Kleifarvatn; Clifton et al., Reference Clifton, Pagli, Jónsdóttir, Eythórsdóttir and Vogfjörd2003), and ends to the east with the Torfajökull volcanic system and probably the Laki fissure eruption (Jakobsdόttir, Reference Jakobsdóttir2008). The largest present-day earthquakes in Iceland occur within the SISZ (e.g., magnitude 6.6 in June 2000), which comprises a Riedel shear zone, where several sets of shear fractures rupture during earthquakes. Most present-day major seismic events (moment magnitude [Mw] > 5) are located in the western part of the SISZ (Jakobsdóttir, Reference Jakobsdóttir2008), from the Hengill Volcano to Lake Kleifarvatn (Hjaltadóttir, Reference Hjaltadóttir2009), where volcanic activity is low today, as well as in the Reykjanes Peninsula.
Despite the general E–W trend of the SISZ, the majority of present-day earthquakes occur along N–S-trending faults (e.g., Rögnvaldsson and Slunga, Reference Rögnvaldsson and Slunga1994; Slunga et al., Reference Slunga, Rögnvaldsson and Bödvarsson1995; Decriem et al., Reference Decriem, Árnadóttir, Hooper, Geirsson, Sigmundsson, Keiding, Ófeigsson, Hreinsdóttir, Einarsson, LaFemina and Bennett2010), suggesting that the left-lateral transform movement is, to a great extent, accommodated by right-lateral displacement along these N–S strike–slip faults, achieved by relatively shallow earthquakes (2–6 km on average with a maximum 10 km depth). The shallowness of this earthquake activity increases its sensitivity to the presence of the water table. Trigger earthquakes, which are the largest-magnitude earthquakes to occur in Iceland (Mw > 5), are commonly followed by a cluster of lower-magnitude events in the following days or months (Decriem et al., Reference Decriem, Árnadóttir, Hooper, Geirsson, Sigmundsson, Keiding, Ófeigsson, Hreinsdóttir, Einarsson, LaFemina and Bennett2010), usually in connection with a temporarily raised water table (Jὀnsson et al., Reference Jónsson, Segall, Pedersen and Björnsson2003).
Historic earthquakes are mainly expressed in the field as large N–S-trending faults arranged side by side (e.g., Einarsson and Eiriksson, Reference Einarsson and Eiriksson1982; Einarsson, Reference Einarsson1991; Bergerat and Angelier, Reference Bergerat and Angelier2003; Einarsson et al., Reference Einarsson, Khodayar, Clifton, Ófeigsson, Thorbjarnarson, Einarsson and Hjartardóttir2005; Bergerat et al., Reference Bergerat, Homberg, Angelier and Bellou2011; Fig. 1C), but left-lateral seismic faults also exist in the present day (e.g., Angelier and Bergerat, Reference Angelier and Bergerat2002) and occurred during historical periods and earlier (e.g., Bergerat et al., Reference Bergerat, Angelier, Guðmundsson and Torfason2003, Reference Bergerat, Homberg, Angelier and Bellou2011; Guðmundsson, Reference Guðmundsson2017). Measurements on basaltic rocks (dated at 0.8–3.3 Ma) cropping out along the Þjórsá River (Bergerat and Plateaux, Reference Bergerat and Plateaux2012) exhibited N45°–N65° (left-lateral) and N10°–E35° (right-lateral) strike–slip faults and N40°–N55° normal faults and veins. Broadly speaking the NE–SW trends correspond to the normal faults and dykes of the volcano-tectonic systems. The expanded sedimentary sequence in the Ytrí Rangá valley corresponds to the crossing zone between the SISZ known seismic faults (Skarðsfjall and Minnivellir; Fig. 1) and the Hekla fault system.
Main volcanic systems
Until now, only three main volcanic sources have been identified through geochemical analysis of tephra and lava from the MIS 5e sedimentary Member C (units C1 to C6) in the Rangá Formation—the Grimsvötn, Bárðarbungá, and Hekla Volcanoes.
The Grímsvötn volcanic system consists of six en échelon fault systems which are between 150 and 190 km in length and central volcanoes. The Grímsvötn Volcano itself is located at the apex of the Icelandic hot spot, some 150 km NE of the Hekla Volcano (Fig. 1). This seems to have been the most active volcano during the Holocene (Larsen et al.,Reference Larsen, Guðmundsson and Björnsson1998; Óladóttir et al., Reference Óladóttir, Larsen and Sigmarsson2011). Its eruption frequency is mostly ca. 10 yr, with an intensity ruled by a 60 to 80 yr cyclicity (Óladóttir et al., Reference Óladóttir, Larsen and Sigmarsson2011). Weichselian activity has been recognized in marine and ice cores down to 127 ka (Davies et al., Reference Davies, Abbott, Meara, Pearce, Austin, Chapman, Svensson, Bigler, Rasmussen and Farmer2014; Voelker and Haflidason, Reference Voelker and Haflidason2015). The volcano appears to have two reservoirs—a shallow one at ca. 3 km depth and a deeper one at 15 km (Reverso et al., Reference Reverso, Vandemeulebrouck, Jouanne, Pinel, Villemin, Sturkell and Bascou2014). The shallow depth of the upper reservoir makes it potentially highly sensitive to rapid unloading resulting from deglaciation events (Höskuldsson et al., Reference Höskuldsson, Sparks and Carroll2006). For example, the complex Saksunarvatn tephra (10.4 to 9.9 cal ka BP; Johannsdottir, Reference Johannsdottir2007) is related to the drainage of the Vatnajökull subglacial lakes or aquifers and the following incomplete deglaciation and rebound of the Erdalen cold events (Van Vliet-Lanoe et al.unpublished). It is connected to a fault system that was responsible for the Laki and older eruptions. This caldera is covered by about 400 m of ice (Bjornsson, Reference Björnsson2009). The subglacial topography of Vatnajökull shows a large triple caldera—a major source of jökluhlaups (Björnsson, Reference Björnsson2002).
The Bárðarbunga Volcano forms a wide caldera, located at the northwestern edge of the Vatnajökull ice cap, that is filled by about 400-m-thick ice (Bjornsson, Reference Björnsson2009). This volcano is located along a large fault system—the Veiðivötn—that parallels the Grimsvötn system but also continues to the northern coast of the island (North Volcanic Zone). Its lavas are tholeiitic in composition. The reservoir is located at about 12 km depth (Guðmundsson et al., Reference Guðmundsson, Jónsdóttir, Hooper, Holohan, Halldórsson, Ófeigsson and Cesca2016). During the Holocene, the eruption frequency was 5 eruptions/100 yr (Óladóttir et al., Reference Óladóttir, Larsen and Sigmarsson2011), also recognized in marine tephra down to 110 ka (Davies et al., Reference Davies, Abbott, Meara, Pearce, Austin, Chapman, Svensson, Bigler, Rasmussen and Farmer2014; Voelker and Haflidason, Reference Voelker and Haflidason2015). This volcano is now emerging from the ice sheet. Due to its wide caldera, it is a major source of jökluhlaups, especially in the southern embayment (Björnsson, Reference Björnsson2002).
The Hekla Volcano is an andesitic stratovolcano (Sigmundsson et al., Reference Sigmundsson, Einarsson, Bilham and Sturkell1995). Its summit reaches 1488 m above sea level (m asl), and it is located on an independent fault system. It is presently sparsely covered by glaciers. The positioning of Hekla corresponds, more or less, to the crossing of this fault system with the SISZ. It has only one deep reservoir, from 14 km to 20 km in depth (see synthesis in Reverso et al., Reference Reverso, Vandemeulebrouck, Jouanne, Pinel, Villemin, Sturkell and Bascou2014). Holocene activity was very frequent (Oladottir et al., Reference Óladóttir, Larsen and Sigmarsson2011), recognized in marine tephra down to 80 ka (Davies et al., Reference Davies, Abbott, Meara, Pearce, Austin, Chapman, Svensson, Bigler, Rasmussen and Farmer2014; Voelker and Haflidason, Reference Voelker and Haflidason2015). The onset of the Hekla Volcano is much older, dating at least back to ca. 417 ka (40Ar/39Ar age of feldspar; Van Vliet-Lanoë et al., Reference Van Vliet-Lanoë, Schneider, Guðmundsson, Guillou, Nomade, Chazot, Liorziou and Guégan2018) and lava and pumices are recorded within the MIS 5e record.
Glaciers
The larger Icelandic glaciers today are mostly polythermal and are frequently (66%) subject to surge dynamics (Björnsson et al., Reference Björnsson, Pálsson, Sigurðsson and Flowers2003; Björnsson, Reference Björnsson2009). Their motion is somewhat helped by the presence of subglacial lakes (Björnsson, Reference Björnsson1998; Auriac et al., Reference Auriac, Sigmundsson, Hooper, Spaans, Björnsson, Pálsson, Pinel and Feig2014). Glaciers are supposed to have completely disappeared during the Holocene thermal optimum (ca. 8 ka; Ingólfsson, Reference Ingólfsson, Maizels and Caseldine1991; Striberger et al., Reference Striberger, Björck, Holmgren and Hamerlik2012), but were restored from 4.5 ka. Nevertheless, while this disappearance may have been the case for small glaciers, such as Eyafjallajökull, it is likely that certain glaciers subsisted on western Vatnajökull, and probably on Langjökull and Hofsjökull, during the optimum (Flowers et al., Reference Flowers, Björnsson, Geirsdóttir, Miller and Clarke2007, Reference Flowers, Björnsson, Geirsdóttir, Miller, Black and Clarke2008; Björnsson, Reference Björnsson2009). The phreatomagmatic character of the MIS 5e 5e-low/BAS tephra in the Rangá Formation (Van Vliet-Lanoë et al., Reference Van Vliet-Lanoë, Schneider, Guðmundsson, Guillou, Nomade, Chazot, Liorziou and Guégan2018) suggests the persistence of some ice during the thermal optimum of the last interglaciation. The age of the tuyas south of the Langjökull ice cap (Van Vliet-Lanoë et al., Reference Van Vliet-Lanoë, Schneider, Guðmundsson, Guillou, Nomade, Chazot, Liorziou and Guégan2018: Table 1) also suggests an early re-extension of this ice cap (MIS 5d). The last deglaciation proceeded from the Bölling interstade (14.5 cal ka BP), with pulsed readvances (Geirsdóttir et al., Reference Geirsdóttir, Miller, Axford and Ólafsdóttir2009) and the development of ice streams (Principato et al., Reference Principato, Moyer, Hampsch and Ipsen2016). The final full deglaciation (beyond the present ice extent) occurred just after 10.3 cal ka BP (Geirsdóttir et al., Reference Geirsdóttir, Miller, Axford and Ólafsdóttir2009).
Permafrost today extends mostly from elevations above 800 m asl in northern Iceland (Etzelmüller et al., Reference Etzelmüller, Farbrot, Guðmundsson, Humlum, Tveito and Björnsson2007) and ca. 1000 m asl to the south. Sporadic permafrost is more widespread down to 600 m asl in southern Iceland. During the MIS 5e cold event (Greenland glacial stadial GS26) at Halslón, permafrost developed from ca. 600 m asl in freshly deposited tills (Van Vliet-Lanoë et al., Reference Van Vliet-Lanoë, Guðmundsson, Guillou, van Loon and De Vleeschouwer2010). This means that early glacier development during MIS 5e and MIS 5d was probably initially polythermal.
Sedimentary record east of the SISZ—the Ytrí Rangá valley
The sedimentary record in this region has been subdivided into several members, with Member A representing the oldest features, and Member B being a glaciovolcanic complex with a K–Ar age close to ca. 155 ka (Van Vliet-Lanoë et al., Reference Van Vliet-Lanoë, Schneider, Guðmundsson, Guillou, Nomade, Chazot, Liorziou and Guégan2018; Table 3)
Member C sensu stricto (Rangá Formation) has an MIS 5 age (Van Vliet-Lanoë et al., Reference Van Vliet-Lanoë, Schneider, Guðmundsson, Guillou, Nomade, Chazot, Liorziou and Guégan2018) and crops out in the Rangá and Þjórsá valleys. It is the main, and most complex, deposit of the Rangá Formation, with an average thickness of 30 m. Most of the material that comprises Member C was inherited from the subglacial eruption(s) of the Hekla Volcano and the old hyaloclastite ridges of the Veiðivötn system (Fig. 1B). The spectacular preservation of this interglacial prism is partly related to major topographical shaping by the MIS 6 ice sheet, the rapid deglaciation of Termination IIb, and the cold-based and low-erosive characters of the Weichselian glaciation (Van Vliet-Lanoë et al., Reference Van Vliet-Lanoë, Van Cauwenberge, Bourgeois, Dauteuil and Schneider2001; Geirsdóttir et al., Reference Geirsdóttir, Miller and Andrews2007). Members D, E, and F encompass the last glacial period, the late-glacial transition, and the Holocene period, respectively. Members E and F are much less interconnected spatially and are much thinner. With the record of a first deglaciation close to 155–150 ka at Ófærugill and Kleifarvatn, Member C in the Ytrí Rangá valley, and the late-glacial–early Holocene record in the south embayment, we have a long sedimentary sequence that covers at least four main deglaciation events. Detailed descriptions and interpretations of the members of this record have been reported in Van Vliet-Lanoë et al. (Reference Van Vliet-Lanoë, Schneider, Guðmundsson, Guillou, Nomade, Chazot, Liorziou and Guégan2018; Table 1 in Supplementary material, p. 9); a composite stratigraphic column is shown in Figure 2.
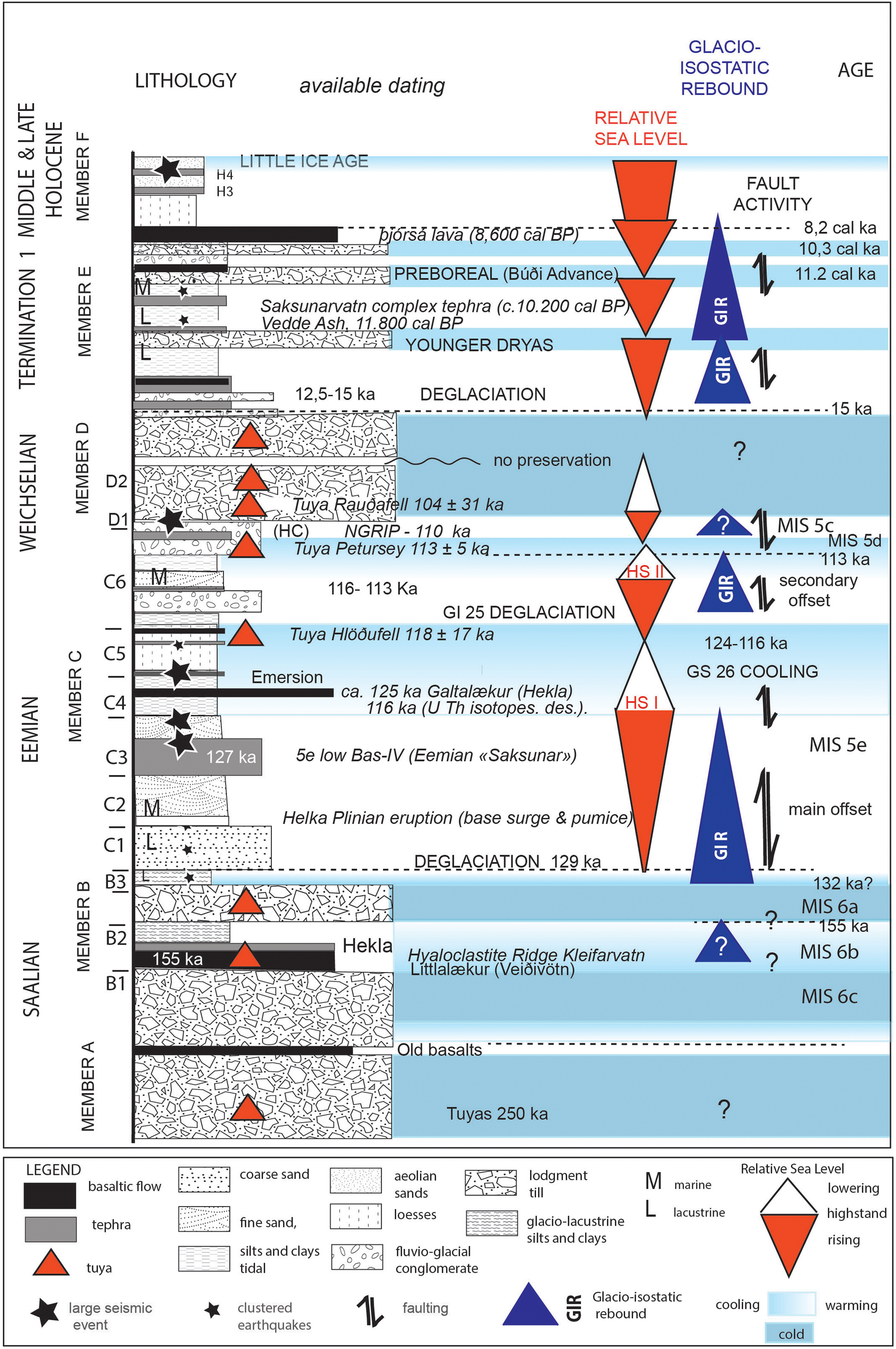
Figure 2. (color online) Composite log of the Rangá Formation. Large seismic events correspond to ruptures in the field. Clustered earthquakes correspond to low-magnitude events (Ms < 5).
Timing
The Ofærugill Member B records a long interstadial, lasting ca. 10 ka under cool conditions, MIS 6b (162–150 ka, Zeiffen interstadial; Seidenkrantz et al., Reference Seidenkrantz, Bormalm, Dansgaard, Johnsen, Knudsen, Kuijpers and Lauritzen1996), followed by the very cold MIS 6a (Kattegat stadial). MIS 6b is associated with the major retreat of all European ice sheets (Toucanne et al., Reference Toucanne, Zaragosi, Bourillet, Cremer, Eynaud, Van Vliet-Lanoë and Penaud2009). The deglaciation was partial, but extensive, occurring here at least south of the Búrfell (Fig. 1B), but also in northern and northeastern Iceland based on K-Ar dates from subaerial lavas (Guillou et al., Reference Guillou, Van Vliet-Lanoë, Guðmundsson and Nomade2010). To the west, hyaloclastite ridges developed as at Kleifarvatn (dated 155 ± 58 ka).
Member C represents an interglacial period, MIS 5e–5d in age, recording a time span of ca. 129–110 ka (Van Vliet-Lanoë et al., Reference Van Vliet-Lanoë, Schneider, Guðmundsson, Guillou, Nomade, Chazot, Liorziou and Guégan2018). Analysis of the uranium isotope disequilibrium yielded a date of 116 ka for the Galtalækur lava (Unit C3), with field evidence suggesting an age of 125 ka (see Fig. 2). The age of this member is confirmed on the basis of paleoclimatic correlations, the occurrence of the 127 ka Grimvötn 1/5e-low/BAS tephra (confirmed by single-grain laser ICPMS analysis; Van Vliet-Lanoë et al., Reference Van Vliet-Lanoë, Schneider, Guðmundsson, Guillou, Nomade, Chazot, Liorziou and Guégan2018, Supplement 3) in Unit C3a, and the presence at Hellar Cave in upper Unit C6, of the “110 ka NGRIP 2745.6 m” tephra (Davies et al., Reference Davies, Abbott, Meara, Pearce, Austin, Chapman, Svensson, Bigler, Rasmussen and Farmer2014 : Grimsvötn volcanic source with a limited Katla Volcano input extracted from microprobe analysis). From the ice-core record of the North Greenland Eemian Ice Drilling project (Dahl-Jensen et al., Reference Dahl-Jensen2013) and the Red Sea coral record (Siddal et al., Reference Siddall, Bard, Rohling and Hemleben2006), the penultimate deglaciation (Termination IIb, 132–130 ka) is known to have been extremely rapid, much more so than the late-glacial deglaciation (Termination Ib). In Iceland, once initiated, this rapid deglaciation proceeded under the direct influence of the mild Irminger Marine Current. Member C is a record of Termination IIb (ca. 130–129 ka), the Eemian optimum (127 ka), the intra-Eemian cooling (GS26 of Greenland stadials, ca. 120–116 ka), and the late warming of glacial interstadial GI25 (116–113 ka; North Greenland Ice-core Project [NGRIP]; McManus et al., Reference McManus, Oppo, Keigwin, Cullen and Bond2002; Andersen et al., Reference Andersen2004; Rasmussen et al., Reference Rasmussen, Thomsen, Kuijpers and Wastegård2003). It preserves two successive and distinct transgressive system tracts, separated by ca. 9 ka—Estuary 1 and Estuary 2—which were forced by two successive glacioisostatic rebounds (Fig. 2) controlled by the vicinity of the main ice sheets. This internal marine regression records the distal signature of a complex glacial advance—GS26—as observed in central Iceland (Van Vliet-Lanoë et al., Reference Van Vliet-Lanoë, Guðmundsson, Guillou, van Loon and De Vleeschouwer2010). The Grímsvötn and Hekla Volcanoes were highly active (tephra, lapilli, and lava flows) during all the deglaciation events, confirming previous observations of Termination Ib (11 ka; Óladóttir et al., Reference Óladóttir, Larsen and Sigmarsson2011). This was also true during the emplacement of Unit C6, during a brief deglaciation—Termination IIc (116–113 ka; Van Vliet-Lanoë et al., Reference Van Vliet-Lanoë, Schneider, Guðmundsson, Guillou, Nomade, Chazot, Liorziou and Guégan2018).
A record of the early Weichselian ice sheet is absent from the sedimentary record in the central embayment, up to at least 110 ka (based on the Hellar Cave tephra), but one is present south of the Langjökull and Vatnajökull Ice Sheets, based on dated tuyas.
Deglaciation was important inland from 14.5 ka cal BP, with a lacustrine facies being deposited close to Hepprehὀlar (Geirsdottir et al., Reference Geirsdóttir, Miller, Axford and Ólafsdóttir2009), which was deformed by the Younger Dryas and Pula–Mykjunes advances, capped by the Vedde Ash (11.8 ka cal BP; Van Vliet-Lanoë et al., Reference Van Vliet-Lanoë, Schneider, Guðmundsson, Guillou, Nomade, Chazot, Liorziou and Guégan2018), and finally by the Preboreal Búði moraine system (11.2 ka cal BP; Geirsdóttir et al., Reference Geirsdóttir, Hardardóttir and Sveinbjörnsdóttir2000; Fig. 1B). The sediments associated with the late-glacial and Preboreal advances are interstratified with jökulhlaup deposits, basaltic tephra, and marine deposits. The highest marine transgression during the Preboreal (Termination Ib) reached a regional elevation of 110 m asl inland in the southern Icelandic embayment, dated as ca. 11.2 ka cal BP, resuming at around 9 ka (as synthesized in Geirsdóttir et al. [2000] and Biessy et al. [2008]). The Holocene record continued with the Þjὀrsá Lava and sandur deposits that were covered by loess from ca. 8.5 ka cal BP and mainly sand dunes during the Little Ice Age (Jackson et al., Reference Jackson, Oskarsson, Trønnes, McManus, Oppo, Grönvold, Hart and Sachs2005).
DATA
Paleoseismicity and fault activity along the SISZ
Paleoseismicity (Rangá Formation)
Deformed sedimentary deposits with co-seismic origins have been clearly differentiated from deformations in periglacial, glacial, and storm-wave breaking conditions on the basis of their topographic locations in basinal or more proximal settings, their drainage capability and grain size, and also their internal organization and fabric (oriented patterns, clustering in nodal positions, shortening fabrics, nontidal layer fragmentation; Van Vliet-Lanoë et al., Reference Van Vliet-Lanoë, Maygari and Meilliez2004, Reference Van Vliet-Lanoë, Bourgeois, Dauteuil, Embry, Guillou and Schneider2005). Evidence includes the occurrence of several clustered co-seismic patterns (e.g., Fig. 3) or/and faulting affecting the same sedimentary unit(s) (e.g., Fig. 4). Their stratigraphic attributions are commonly given by the age of the affected upper boundary of the unit, except for small-sized synsedimentary patterns. This approach has not been applied to volcanogenic sedimentary sections in connection with existing fault zone (Fig.5).

Figure 3. (color online) Low-intensity co-seismic sedimentary deformations at Heidarbrekka West: (A) Unit C2; (B) Unit C3a, synsedimentary water escape that reworked C2 material and was emitted from the top of Unit C3a; (C) dish structures disturbing the stratification (Unit C3a); (D) flame structures or injections of Unit C2 material along fractures (SISZ) in Unit C3a; (E) oriented and clustered microloads in early Unit C4; (F) Synsedimentary and clustered loadcasts of the Grim 1 redeposited tephra in Unit C3a.

Figure 4. Faulting and co-seismic activity during the late-glacial period and early Holocene (Member F). (A, B) SISZ faults from aerial views (N10°E in lavas overlapping the Bύði moraine, 10.2 ka), sealed by the Þorsá Lava (8.6 ka). (C) Synsedimentary micro-involutions due to tremors (Kelvin–Helmholtz convolutions) in early Holocene tidal rhythmites (ca. 9.6 ka). (D) Earthquake-graded gravel in a raised beach, close to Bitra (SISZ), south of Lake Hestvan (ca. 9.6 ka). (E) Offset accommodation of a tillite (11.4 ka BP) versus glaciofluvial sediments in a small graben located on a SISZ fault, Akbraut. Thick line, fault; thin line, glaciotectonic feature.

Figure 5. Fault systems in the Rangávellir. (A) Unit C2. UVA-generated orthophotograph showing the net of strike faults. The Hekla system is N45° to N60°. The SISZ is more variable in direction, including N45° and N155° fractures, but the main faults trend N10°. Typical trends are indicated here as “SISZ” and “HEKLA.” The detailed picture is located 100 m to the right of the main view. Here, the faulting also affected Unit C3b. (B) Normal feed dykes of the Hekla fault system below a pillow lava accumulation, at the base of the Stöng sequence (Unit B1).
Two types of paleoseismic records have been observed.
Type 1. Low surface-magnitude earthquakes: Evidence of recurrent low surface–magnitude (Ms) seismicity includes small (<25 cm) water-escape structures (Obermeier, Reference Obermeier and McCalpin1996; Fig. 3A), small loadcasts (Sims, Reference Sims1975; Van Loon, Reference Van Loon2009; Fig. 3F), oriented loadcasts in nodal position (Van Vliet-Lanoë et al., Reference Van Vliet-Lanoë, Maygari and Meilliez2004; Fig. 3E), Kelvin–Helmholtz subaqueous instabilities (Heifetz et al., Reference Heifetz, Agnon and Marco2005), and dish-and-plate structures (Lowe and LoPiccolo, Reference Lowe and LoPiccolo1974; Fig. 3C) during the deposition of Units B2 and B3 (ca. 155–150 ka, deglaciation event) and C1 and C2 (ca. 129–127 ka; Fig. 2) of the Ytrí Rangá long sequence (Van Vliet-Lanoë et al., Reference Van Vliet-Lanoë, Schneider, Guðmundsson, Guillou, Nomade, Chazot, Liorziou and Guégan2018). Co-seismic synsedimentary layer fragmentation (Owen et al., Reference Owen, Moretti and Alfaro2011) is uncommon in the Rangá sediments, as are layer stretching and disruption. This is related to the low-angled slopes in the paleo-estuary compared with their frequency in the incised valleys of northern Iceland (Van Vliet-Lanoë et al., Reference Van Vliet-Lanoë, Bourgeois, Dauteuil, Embry, Guillou and Schneider2005).
These events are synchronous with the first transgressive event within MIS 5e (Termination IIb). A similar situation also prevailed during the inundation of Termination Ib at Akbraut, between ca. 11.2 (from 14C) and 11.4 cal ka BP (Figs. 1C, 4C), with synsedimentary fragmented layers and small loadcasts in association with some limited activity in the SISZ fault system (see Supplementary Material).
Type 2. High surface–magnitude earthquakes: Large MIS 5e earthquakes have mostly been recorded in the Ytri Rangá valley. At least two successive seismic crises were recorded during emplacement of Units C3–C4 (Figs. 3, 6D, and 7B) at Heiðarbrekka. Plurimetric convolutions were observed at Kirkjubær (Fig. 1), with shortening figures (Fig. 7B), just below the second transgression surface (base of Unit C6).
The earthquake evidence at the top of Unit C4 (ca. 126–125 ka; Fig. 6D) was associated with evident ruptures in the SISZ (Fig. 1C) and superficial folding (Fig. 7A, basaltic tephra He 2 in C4), synchronous with the hydrostatic unloading resulting from the first regression. It should be noted that the traces of paleo-earthquake ruptures are located in the southern prolongation of the Minnivellir–Skardsfjall fault system (earthquake of 1630; Einarsson and Eiriksson, Reference Einarsson and Eiriksson1982; Einarsson et al., Reference Einarsson, Böttger and Thorbjarnarson2002; Bergerat et al., Reference Bergerat, Homberg, Angelier and Bellou2011; Fig. 1C). The rupture observed in lower Member D (Fig. 7C and D) occurred on the same system, but at ca. 110 ka during the last unglaciated interval in the Rangá valley.
The first type is rather ubiquitous in deglaciation sedimentary facies, together with fault offsets in the sediments (Figs. 3, 5, and 6, Table 2 in the Supplementary Material) as commonly observed in western Europe and Alaska (Sauber and Molnia., Reference Sauber and Molnia2004; Hampel et al., Reference Hampel, Hetzel, Maniatis and Karow2009; Brandes and Winsemann Reference Brandes and Winsemann2013). The second is commonly observed near known Holocene SISZ faults during full interglacial intervals (Fig. 4).
Figure 6. (color online) Fault activity at Heiðarbrekka West. (A) Synsedimentary rollover on a N48° trending fault of the Hekla system. (B) Same structure observed to the north. (C) Crossing of the SISZ and Hekla fractures. (D) Superficial evidence of rupture in Unit C4, SISZ-trending fractures sealed by Units C5 and C6.
Figure 7. (color online) Faulting, folding, and synsedimentary loading, Rangávellir. (A) Heiðrabrekka West, local synsedimentary compressive structure (SISZ), sealed by unit C5. (B) Kirkjubær, Unit C3a, mega loadcasts with shortening figures. (C, D) Heiðrabrekka East, faulted units from the base of Member D in the SISZ system, cropping out in the Vikinglækur River, sealed by intact till (Member E), older than the Preboreal terminal moraine at ca.150 m north of the faults.
Fault activity (Rangá Formation)
Significant tectonic activity occurred during the progressive inundation of the Ytrí Rangá valley (Units C1 and C2; Fig. 5), in connection with low-magnitude earthquakes. There are two major sets of fractures—N40° to N70°, belonging to the Hekla system and being more scattered, and N170° to N210°, related to the SISZ. The fault set that follows the Hekla system is limited to the eastern side of the paleo-estuary (Fig. 2B). In the Rangá Formation, this fault set is associated with eruptions of the Hekla system with an early Plinian one (pumice and base surge in upper C2 unit, Supplementary Material) or basaltic tephra and the dated lava flow at Galtalækur (C3 unit). It also seems to support rapid unloading-related dyke injection in a subglacial position (Johnston, Reference Johnston, Gregerson and Basham1989; Stewart et al., Reference Stewart, Sauber and Rose2000), as with the older pillow lava close to the base of the Stöng and Ofærugill sequences (base of Unit B2; Fig. 5B). Renewed, slow fault activity, accommodated by a tidal rhythmite, seems to have been synchronous with the second inundation (Unit C6 at Heiðarbrekka, maximum flooding surface 2; Fig. 2) and was related to a temporary full deglaciation close to 116 ka, probably at altitude (>500 m asl), on the Hekla fault system (Figs. 1C and 6). Similarly, during the early Holocene at Akbraut, SISZ fault activity is recorded as a metric offset, deforming glaciofluvial deposits. This is associated with recurrent low-magnitude seismicity, further accommodated by an early tillite from an 11.4 cal ka glacial advance (Fig. 4E) deforming a Bárðarbunga tephra estimated at ca.11.35 cal ka (Lind and Wastergård, Reference Lind and Wastegård2011).
West of the SISZ—Kleifarvatn and the hyaloclastite ridge of Hellutindar
Kleifarvatn is presently a lake, located in a N30°- and N90°-controlled graben, with its western side occupied by a complex hyaloclastite ridge. N–S-striking faults have been observed at several locations on the Reykjanes Peninsula (Guðmundsson, Reference Guðmundsson1987; Clifton et al., Reference Clifton, Pagli, Jónsdóttir, Eythórsdóttir and Vogfjörd2003). Kleifarvatn corresponds with the connection between the SISZ and the Reykjanes Peninsula, west of Hengill Volcano (Fig. 2). This sequence has not yet been described. The base of the hyaloclastite ridge of Hellutindar (Fig. 8B) seems monophasic to the northeast, in the quarry at the foot of the Háuhnύkar Tindar (ridge prolongation; Fig. 1C, Supplementary Material), represented by an accumulation of slightly stratified, nonvesicular pillow lava (>40 m high; Fig. 8C). A late graben, with a complex morainic infill, longitudinally deforms this accumulation (Fig. 8D). Stratified hyaloclastites, tuffs, and, very locally, lapilli facies dominate toward the southwest. All these surfaces are glacially abraded. Several SISZ faults cross the ridge in narrow grabens, especially near the north coast of the Innristapi Peninsula, (Fig. 8D, Supplementary Material). Similar fault directions exist in the lake (Friðriksson, Reference Friðriksson2014), associated with a N–S sublacustrine ridge and hot springs (Fig. 8A), also seen north of Krisuvík. It should be noted that this N–S ridge is composed of pillow lava, is connected to the main hyaloclastite ridge (Friðriksson, Reference Friðriksson2014; Fig. 8A), and lacks traces of glacial erosion. Some dark dismantled lava crops out at the top of Hellutindar ridge (Fig. 8E, black).

Figure 8. (color online) Kleifarvatn volcanic ridge record. (A, B) Pillow lava ridge, 800 m long, extending along a N–S fissure, south of Syðristapi (multibeam DEM, modified after Friðriksson, Reference Friðriksson2014, Figure 24, exaggerated twice in altitude; global picture from Lanmælingar Island). Pillow lavas also crop out at the southern extremity of the lake, at Lambatangi, with both N90° and N30° fractures; they are glacially abraded. (C) Pillow lava accumulation with feed dyke in the same hyaloclastite ridge, Háuhnύkar Quarry. (D) Longitudinal graben in the same hyaloclastite ridge, Háuhnύkar Quarry, filled by faulted tillite. (E) Sketch of deglaciation of the Hellutidar–Helgafell ridge, with basal pillow lava, laminated hyaloclastites to the south, and tuff accumulation, in early deglaciated zones (the time scale is hypothetical). Black bodies are postglacial basaltic scoria splays (Eemian?).
The sedimentary sequence crops out on the Syðristapi (165 m asl) and Innristapi Peninsulas (210 m asl) in direct continuity of the last deposits of the hyaloclastite ridge. It is not possible to define precise units, because the facies is rather similar from the base to the top of the sequence. The lower unit—Unit A—consists of hyaloclastite deposits, washed downslope and faulted, on the Innristapi Peninsula (165 m asl; Figs. 8B and 9), attesting to an early collapse of the Kleifarvatn graben, scoured by a stratified till. It seems to correspond to a first transgression, associated with a deglaciation. It is covered by a local, thick basaltic tephra (lapilli) and is overlapped unconformably by later terrace sand deposits. These peninsulas are both covered by two sets of marine or lacustrine deposits that are devoid of bioturbation. Unit B, above Syðristapi, rests unconformably on the hyaloclastites at ca. 210 m asl and appears to be mostly subaquatic, exhibiting a few dropstones, water-escape features (Fig. 9), and reworked encapsulated tephra pellets (phreatomagmatic activity on the ridge). It probably signifies a highstand. The latest unit—Unit C (165 m asl)—is simpler and better stratified, with only limited small-convoluted bedding, and rests unconformably on Unit A. It signals a second highstand. It is further strongly faulted in the N30° and N90° directions. This water-lain formation mainly formed raised coastal ridges at 165 and 200 m asl (Supplementary Material) in the vicinity of the present-day coastline. It was strongly consolidated, latterly reddened, and deformed by a listric strike collapse toward the present lake. The lower level in altitude (Unit C) is much less developed, but forms a flat terrace at 165 m asl, also latterly reddened. The entrance to the graben at Krýsuvík is marked by a wide, perched marine abrasion surface at 80–110 m asl, supporting the old, abraded Selalda volcanic cones (reactivated during Termination Ib (Sæmundsson et al., Reference Sæmundsson, Sigurgeirsson, Hjartarson, Kaldal and Kristinsson2016), armored by N120° dykes, and also rubified. The sequence at Kleifarvatn was further faulted by glaciotectonics and incised by meltwater channels.
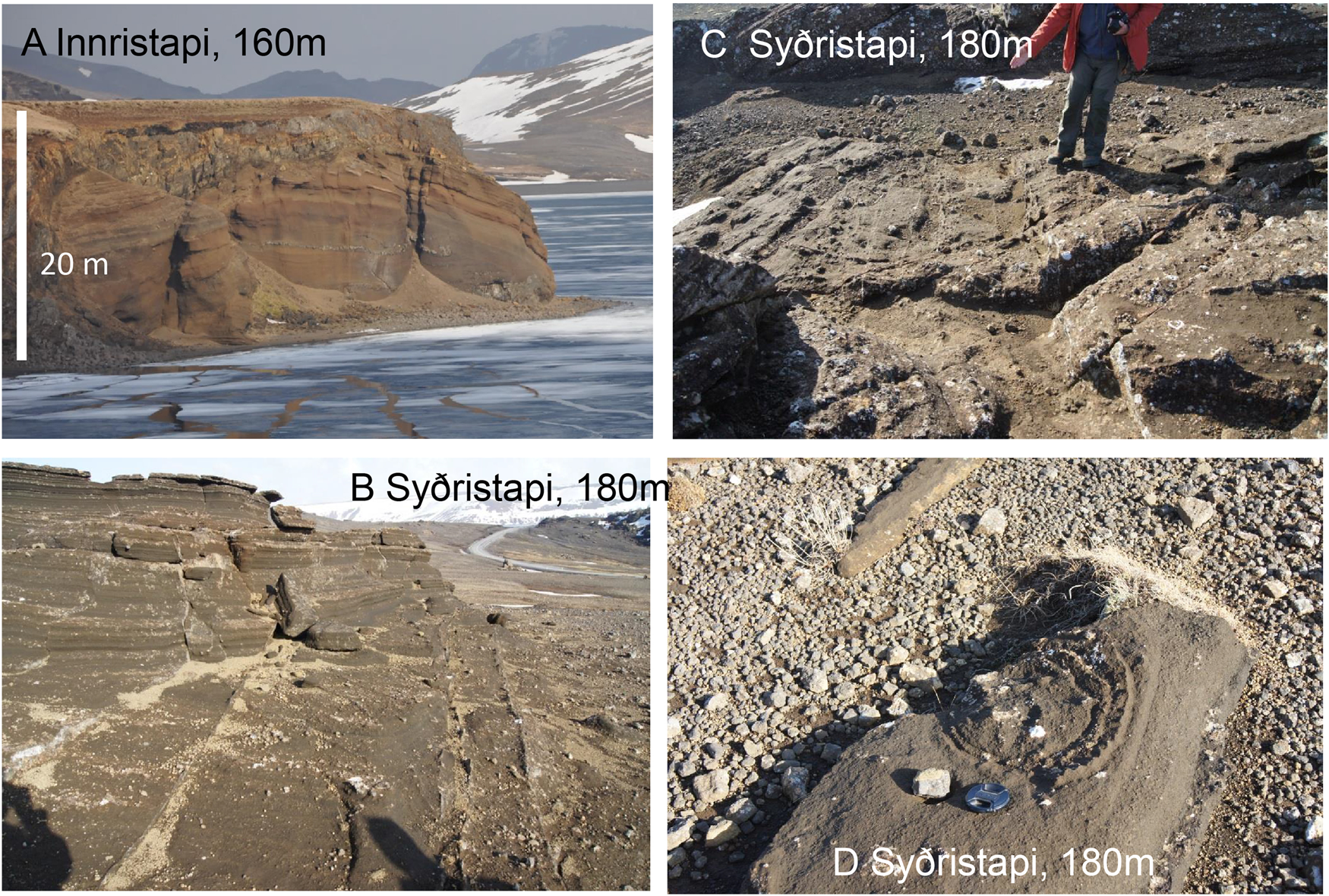
Figure 9. (color online) Kleifarvatn graben sedimentary record. (A) View of the Innristappi Peninsula, showing the strike-faulted Rangá Formation (lower unit of the formation; section ca. 17 m high, summit 160 m asl). (B, C) Two sets of faulting on the Syðristapi Peninsula (180 m asl), Unit B. (D) Horizontal cross-cutting of a water escape in Unit B. Glacial erosion was significant during the last deglaciation.
The Hellutindar laminated hyaloclastite ridge was dated at 155 ± 58 ka (MIS 6b deglaciation age) based on a subglacial laminated hyaloclastite interstratified with basaltic sills (Sample ISLN-109; Table 3, Supplementary material) and signifies an ice margin position (Smellie, Reference Smellie2008). Its prolongation to the northeast, the Háuhnύkar Tindar ridge, is deformed as a graben with a complex morainic infill probably of MIS 6a age, further glacially abraded. This infill as also the subaerial sedimentary sequence of Kleifarvatn may thus be attributed to an interglacial period younger than the MIS 6a, but prior to the last glacial period (late-glacial abrasion). It thus records the MIS 5e interglaciation and yields a similar history to those observed in the central embayment (Table 1). Major faulting on the Innristappi Peninsula, following the Reykjanes fault system, most likely records the 155–145 ka deglaciation (MIS 6b), similar to the Ófærugill record at the foot of the Hekla Volcano (Van Vliet-Lanoë et al., Reference Van Vliet-Lanoë, Schneider, Guðmundsson, Guillou, Nomade, Chazot, Liorziou and Guégan2018). During the MIS 5e, Kleifarvatn formed a narrow bay that opened to the ocean during the deglaciation, with a relative sea level close to 210 m asl, probably corresponding to the level of MIS 5e Transgression I, with some volcanic activity on the ridge (lapilli), as recorded in Ytri–Rangá (215 m asl), and associated with marked faulting following both the SISZ and Reykjanes Peninsula trends. The second highstand is not well represented by sediments at 165 m asl (coastal ridges on an abrasion surface), due to the morphology of the bay (no fluvioglacial input), but the formation of the large tidal flat, close to 80–110 m asl, at the entrance to the bay at Krýsuvík (Fig. 2) attests to a progressive lowering of sea level between the two highstands, or, latterly, during the onset of the last glaciation.
Table 1. Timing of the tectonic and seismic activity on the southern Icelandic embayment.

a The number of + indicates the relative frequency of jökulhlaups.
b Rupture: fault with offset recorded in stratigraphy and morphology; co-seismic: any kind of co-seismic features.
DISCUSSION
Our observations have defined the first relative chronological record for all of the seismic and tectonic activity detected during several deglaciations in southern Iceland (Fig. 2, Table 1) in connection with the setting of tephras, lavas, and hyaloclastites ridges.
The knowledge of the stress state is important to distinguish faulting induced by glacial unloading (e.g., Wu and Hasegawa, Reference Wu and Hasegawa1996; Lund, Reference Lund, Hora and Jensen2005; Connor et al., Reference Connor, Chapman and Connor2009), often pasted with silty coatings, as opposed to pure tectonic activity. In Iceland, the stress state, controlled first by both plate divergence and volcanism, without glaciers, is responsible for the SISZ fault zone. The magma in Iceland is mostly supplied by the hot spot and the oceanic ridge, independent of glacial activity. The extension of the fault system seems to have been essentially controlled by magmatic overpressure, and commonly induced swell and fracture/dyke propagation and dyke intrusions appear to reduce the accumulated strain energy and bring the state of stress close to lithostatic (Guðmundsson, Reference Guðmundsson2000). It resulted in the emplacement of significant subglacial volcanic products related to the supplementary melting in the upper mantle, such as tuyas and hyaloclastite ridges at the outer margins of the ice sheet, on reactivated rifts. Moreover, the ice cap also interacts as a confining system (Edwards et al., Reference Edwards, Guðmundsson, Russell, Sigurdsson, Houghton, McNutt, Rymer and Stix2015). This should be especially true during a full glaciation, with the subglacial and subaerial lava emissions being emitted to the ice-sheet margins. This emission was prolonged during deglaciation by a remaining flat potentiometric surface.
Volcanism
The difference in height of the subglacial volcanoes or tuyas above the Icelandic plateau is considered to reflect a normal decrease in Pleistocene ice thickness toward the coast (Walker, Reference Walker1965; Licciardi et al., Reference Licciardi, Kurz and Curtice2007); however, it can also be considered to be a measure of the slope of the associated potential that over millennia (timing in ka), drove magma from the hot spot along the volcanic zones to the SISZ (Guðmundsson, Reference Guðmundsson2000). Iceland is thus responding, in part, to the increased production of magma and its hydration (Wylie et al., Reference Wylie, Helfrich, Dade, Lister and Salzig1999), as well as the propagation over centuries of fluidized magma limited to the already/recently unloaded zones (Eason et al., Reference Eason, Sinton, Grönvold and Kurz2015).
During MIS 5, Grímsvötn seems to have been the most active and deglaciation-sensitive volcano (Van Vliet-Lanoë et al., Reference Van Vliet-Lanoë, Schneider, Guðmundsson, Guillou, Nomade, Chazot, Liorziou and Guégan2018), probably in reaction to the location of its upper magmatic chamber (3 km) and its recurrent feeding by dykes issuing from the hot spot (Guðmundsson, Reference Guðmundsson2000). This was also true during the Holocene (Óladóttir et al., Reference Óladóttir, Larsen and Sigmarsson2011) and the entire last glaciation (Davies et al., Reference Davies, Abbott, Meara, Pearce, Austin, Chapman, Svensson, Bigler, Rasmussen and Farmer2014; Voelker and Haflidason, Reference Voelker and Haflidason2015; Fig. 10). The thick phreatomagmatic Grímsvötn 5e-low/BAS tephra of Unit C3 in its combination of successive eruptions presents a volume and a setting similar in timing to the complex Holocene Saksunarvatn tephra (see “Main Volcanic Systems”). This could signal the full entrance of the volcano into an interglacial regime. To control Grímsvötn's eruption susceptibility, we also analyzed past eruptive activity in the studied and adjacent volcanic zones. In the Ytrí Rangá valley, Member B (Unit B2) was emplaced during the penultimate glaciation (MIS 6; Fig. 10). The significant loading during this major glaciation apparently caused the Hekla fault system controlling the volcanic zone to become enlarged (Bourgeois et al., Reference Bourgeois, Dauteuil and Van Vliet-Lanoë1998), extending to the Norðubjallar ridge, 4 km distant from the main eruptive fissure, or even to Stöng (10 km; Figs. 1B and 5). Similarly, the early Plinian eruption of the Hekla occurred close to 129–128 ka (Fig. 3), after the deglaciation of Termination IIb and an early sagging of the estuary.

Figure 10. (color online) Stratigraphical and chronological relationships between volcanic activity (tephra, ridges and tuyas) and climate. NGRIP δ18O curve (Andersen et al., Reference Andersen2004). Calving events and thermohaline circulation restoration after Rassmusen et al. (Reference Rasmussen, Thomsen and Moros2016). Numbers between brackets correspond to estimated ice thickness on each tuya. Dating of tuyas shown in Table 1. Tephra: Oladottir et al. (Reference Óladóttir, Larsen and Sigmarsson2011), Davies et al. (Reference Davies, Abbott, Meara, Pearce, Austin, Chapman, Svensson, Bigler, Rasmussen and Farmer2014), Voelker and Haflidason (Reference Voelker and Haflidason2015), Van Vliet-Lanoë et al. (Reference Van Vliet-Lanoë, Schneider, Guðmundsson, Guillou, Nomade, Chazot, Liorziou and Guégan2018). Ocean Drilling Program 983 IRD record after Barker et al. (Reference Barker, Chen, Gong, Jonkers, Knorr and Thornalley2015).
A similar situation was highly probable during the deglaciation event close to 155 ka (MIS 6b), when subglacial lava flows issued from Veiðivötn fault system (Fig. 1) and reached at least the Búrfell in the Ytri Rangá valley to become subaerial (Littlalækur; Table 1). The setting of the Unit B2a volcaniclastic deposits represents an intra-MIS 6b eruption of the Hekla Volcano at about the same age. This suggests synchronous major subglacial fissural activity in the entire EVZ (Fig. 1B). The early lapilli deposit at Inristappi yielded a similar signature for the WVZ. Other ridges were probably also synchronously active to the east (Fig. 1B), the south, and the west on the Reykjanes Peninsula (ca. 155 ka; Fig. 1B and C, Table 1). Their peculiar preservation is probably linked to the rapid glacial re-extension from 145 ka (MIS 6a). This volcanic activity on these enlarged subglacial ridges led to the emplacement of large volumes of hyaloclastite-derived sediments, located along the fault system during the MIS 6 deglaciation (Member C; Units C1 to C3). The record from ice and marine-sediment cores has not previously provided such evidence for a tephra emission from Grímsvötn Volcano at that time, but has well favoured a massive exportation of hyaloclastites-derived sediments (Bout-Roumazeilles et al., Reference Bout-Roumazeilles, Debrabant, Labeyrie, Chamley and Cortijo1997).
Buildup and decay of the Icelandic ice sheet
The buildup of the Icelandic ice sheet was quite discrete in terms of timing, being under the control of available precipitation (Broecker and Denton, Reference Broecker and Denton1990). Its thickness was modulated by warming events or by precipitation starvation. It was favored by higher precipitation to the south and west, with a low snowline (Ahlmann and Þorarrinsson, Reference Ahlmann and Þorarrinsson1937), as shown by the early MIS 5e development of the Mýrdalsjökull and Langjökull glaciers (Van Vliet-Lanoë et al., Reference Van Vliet-Lanoë, Schneider, Guðmundsson, Guillou, Nomade, Chazot, Liorziou and Guégan2018). The northeast was more arid than today with a raised snowline (Hanna et al., Reference Hanna, Jonsson and Box2004), thus relatively starving the development of the ice cap. During cooling events, the glacial dynamics seem to have been cold-based, with steep borders. During warming events, precipitation rose and glaciers evolved into polythermal or maritime types, with much more gradual slopes (Kerr, Reference Kerr1993). These warming events were driven by DO events (Rasmussen et al., Reference Rasmussen, Thomsen and Moros2016). Consequently, interstadial events raised the plasticity of the ice and increased sliding, favoring surging (Pattyn, Reference Pattyn2003), ice shelf building, and glacial thinning. DO events resulted in rather continuous calving, with IRD recorded around Iceland (Fig. 10), especially from 40 ka (Jennings et al., Reference Jennings, Syvitski, Gerson, Grönvold, Geirsdóttir, Hardardóttir, Andrews and Hagen2000; van Kreveld et al., Reference van Kreveld, Sarthein, Erlenkeuser, Grootes, Jung, Nadeau, Pflaumann and Voelker2000; Geirsdottir et al., Reference Geirsdóttir, Andrews, Ólafsdóttir, Helgadóttir and Harðardóttir2002; Bauch and Erlenkeuser, Reference Bauch and Erlenkeuser2008; Barker et al., Reference Barker, Chen, Gong, Jonkers, Knorr and Thornalley2015; Fig. 10). Surging is common during melting events, especially in temperate-based or polythermal Arctic glaciers (Fowler et al., Reference Fowler, Murray and Ng2001; Sevestre et al., Reference Sevestre, Benn, Hulton and Bælum2015), due to gravitational spreading of the inland ice sheet with the development of tidal calving and ice-stream thinning (Fig. 10). A volcanogenic water supply may have also helped the surging (Björnsson, Reference Björnsson2009).
To evaluate the thickness of the ice sheet, we compared the altitudes of the tuya plateaus (Walker, Reference Walker1965) with their topographical heights, arbitrarily adding a supplementary 400 m, which corresponds to the present-day ice thickness on the Grímsvötn volcanic caldera (Björnsson, Reference Björnsson2009). This value is a minimum that could have been significantly greater, but still less than twice as thick from inland field data (nunataks, dated tuyas), with the exception of the thicker and more extended MIS 6, 10, 12, and 16 ice sheets. MIS 6 ice sheets were usually very thick and extended in the Northern Hemisphere (Svendsen et al., Reference Svendsen, Gataullin, Mangerud, Polyak, Ehlers and Gibbard2004; Lang and Wolf, Reference Lang and Wolff2011), as well as in Iceland (Van Vliet-Lanoë et al., Reference Van Vliet-Lanoë, Bourgeois, Dauteuil, Embry, Guillou and Schneider2005; Geirsdóttir et al., Reference Geirsdóttir, Miller, Axford and Ólafsdóttir2009), fitting the 1500–2000 m requirements used for models (e.g., Patton et al., Reference Patton, Hubbard, Bradwell and Schomacker2017). In the south, such an average ice thickness was quite regular over the last glacial period, being between 800 and 1000 m (Fig. 10), much thinner than the 1500–2000 m used in models of massive “Weichselian inlandsis,” speculated on offshore undated terminal moraines (e.g., Ingólfsson et al., Reference Ingólfsson, Norddahl and Schomaker2010; Patton et al., Reference Patton, Hubbard, Bradwell and Schomacker2017). This has been confirmed by the analysis of pressure-sensitive gas inclusions in quenched basalts by Tuffen et al. (Reference Tuffen, Owen and Denton2010) and volcaniclastic subglacial facies.
Glacioisostatic pressure, rebound, and seismic activity
The seismic activity in the SISZ during the Holocene was mostly related to plate-pull, accommodated by volcanic activity shear stress (Guðmundsson, Reference Guðmundsson2000; Guðmundsson, Reference Guðmundsson2006). The current seismic strain release in Iceland is controlled by rifting and by glacial loading and unloading (Sauber and Monia, Reference Sauber and Molnia2004). As the lithosphere deforms, the downbending depending primarily on the extent, the area covered by the ice sheet (Guðmundsson, Reference Guðmundsson1999) under control by the viscosity of the mantle, and the regional ice-loading history (Cuffey and Paterson, Reference Cuffey and Paterson2010). During interstadials and deglaciations, the SISZ reacted differently, as a significant amount of the shear stress (differential uplift and plate-pull) was compensated for by the injection of magma into dykes (Guðmundsson, Reference Guðmundsson2000) and fault offsets. Presently, uplift related to the rapid deglaciation caused by modern global warming has reached ca. 2 cm/yr for the Vatnajökull ice sheet (Sigmundsson et al., Reference Sigmundsson, Pinel, Lund, Albino, Pagli, Geirsson and Sturkell2010). Faster rates (6.9 to 8.2–10.5 cm/yr) have been estimated for Termination Ib in southern Iceland (Ingólfsson et al., Reference Ingólfsson, Norðdahl and Haflidason1995; Biessy et al., Reference Biessy, Dauteuil, Van Vliet-Lanoë and Wayolle2008).
Two types of paleoseismic records exist in the SISZ region. The first is associated with sediments offset by faults (abrupt or progressive) and recurrent low-Ms seismicity during the deposition of units the deglaciation of MIS 6b and ca. 129–127 ka (final MIS 5e deglaciation) of the Ytri–Rangá long sequence. This supports rapid unloading events, with active volcanism and dyke injection (Johnston, Reference Johnston, Gregerson and Basham1989; Stewart et al., Reference Stewart, Sauber and Rose2000) along the Hekla fault system and also along the N–S faults of the SISZ (at Kleifarvatn). This is also the case for the last deglaciation (12–10.3 ka), as recorded at Akbraut (Fig. 4) and in northern Iceland (15–10.3 ka; Van Vliet-Lanoë et al., Reference Van Vliet-Lanoë, Bourgeois, Dauteuil, Embry, Guillou and Schneider2005) and for recent global warming (Bergerat and Angelier, Reference Bergerat and Angelier2003; Lindman et al., Reference Lindman, Lund and Roberts2010) in the vicinity of Vatnajökull. Moreover, these events are often associated, based on the stratigraphy, with evidence of jökulhlaups (Table 1), a phenomenon commonly triggered by subglacial eruptions related to deglaciation (Björnsson, Reference Björnsson2002, Reference Björnsson2009).
The second type, corresponding to large interglacial earthquakes, is mostly recorded in the Ytrí Rangá valley during the end of the first transgression, in association with true ruptures in the SISZ, volcanic eruptions related to a rapid hydrostatic unloading downstream (onset of the first regression), and rapid glacial loading on the mountains (cooling GS26).
It should be noted that traces of paleo-earthquake ruptures are located in the southern prolongation of the Minnivellir–Skarðsfjall fault system, which has been activated several times historically (Einarsson et al., Reference Einarsson, Böttger and Thorbjarnarson2002; Bergerat and Angelier, Reference Bergerat and Angelier2003; Bergerat et al., Reference Bergerat, Guðmundsson, Angelier and Rögnvaldsson1998; Bergerat et al., Reference Bergerat, Homberg, Angelier and Bellou2011). The type and size of the co-seismic features suggest events of Mw > 6 (Obermeier et al., Reference Obermeier, Martin, Frankel, Youd, Munson, Munson and Pond1993; Olson et al., Reference Olson, Green and Obermeier2005) as was also the case in the early Holocene, as evidenced by the massive graded gravels in a raised beach at Þjoðolfshag (SISZ, Fig. 6D) following the experiments of Lagerbäck and Sundh (Reference Lagerbäck and Sundh2008).
Deglaciation microseismicity
Large glacial loads generally suppress earthquakes, but conversely, rapid deglaciation promotes earthquakes in freshly deglaciated zones (Johnston, Reference Johnston, Gregerson and Basham1989; Hasegawa and Basham, Reference Hasegawa, Basham, Gregerson and Basham1989), as seen in Greenland today (Olivieri and Spada, Reference Olivieri and Spada2015). In Iceland, seismicity is complicated by volcanism and the possibility of dyke injection. Low-level seismicity has been systemic in Iceland during deglacial events, whatever their significance, usually associated with dyke injection in northern Iceland (Einarsson and Brandsdottir, Reference Einarsson and Brandsdottir1980; Van Vliet-Lanoë et al., Reference Van Vliet-Lanoë, Bourgeois, Dauteuil, Embry, Guillou and Schneider2005). Dyke intrusions along the Hekla or Kleifarvatn systems seem to have lowered the accumulated strain under deglacial conditions, especially close to the margins of the melting ice sheet. This is also valid for interglacial aftershocks that temporarily raise the water table and increase microseismicity (Saar and Manga, Reference Saar and Manga2003; Jὀnsson et al., Reference Jónsson, Segall, Pedersen and Björnsson2003; Decriem et al., Reference Decriem, Árnadóttir, Hooper, Geirsson, Sigmundsson, Keiding, Ófeigsson, Hreinsdóttir, Einarsson, LaFemina and Bennett2010).
As explained earlier, the Termination IIb continental deglaciation (130–129 ka) proceeded faster than Termination Ib (11.7 ka). This explains the presence of lacustrine facies (Units B3 and C1) before marine flooding, with sea-level rise beginning around 135 ka (Termination IIa; Siddal et al., 2006; Fig. 10). Termination Ia (14.5 ka; Bölling Interstadial) and the MIS 6b long interstadial (155–145 ka; Ofærugill sedimentary record) evolved similarly. Because MIS 6 resulted in a relatively very large ice sheet, deglaciation rapidly moved landward with sagging, as proven by the progressive extent of the Rangá paleoestuary to the northeast (Van Vliet-Lanoe et al., Reference Van Vliet-Lanoë, Schneider, Guðmundsson, Guillou, Nomade, Chazot, Liorziou and Guégan2018).
Deglaciation faulting
Increased pore pressure during deglaciation tends to weaken fault stability (Sigmundsson, 2006; Lund and Näslund, Reference Lund, Näslund, Connor, Chapman and Connor2009), with the greatest effects at the end of glaciation due to a greater available water supply. On the other hand, rapid ongoing marine transgressions are responsible for hydrostatic loading on the formerly emerged platform, allowing relatively very fast flooding of the isostatically depressed zone. Loading may have increased N45° tension features along the Hekla fault system, and widening it by dyke injection, generating hyaloclastite ridges. To this day, these faults guide the superficial fluvial drainage (see Supplementary Material) and are frequently armored with dykes along the northwestern flank of the ridge. Moreover, this fault system represents evidence of an en échelon functioning (Allemand et al., Reference Allemand, Brun, Davy and Van Den Driessche1989) of the Hekla system, in agreement with the thin and brittle character of the regional crust (Einarsson et al., 2006). If this system has been active since ca. 417 ka (dated feldspar from the ISLN112 Hekla pumice; Van Vliet-Lanoe et al., Reference Van Vliet-Lanoë, Schneider, Guðmundsson, Guillou, Nomade, Chazot, Liorziou and Guégan2018), then this would imply an offset of 0.25 mm/yr, restricted to deglaciation periods only, with the displacement possibly reaching ca. 10 m by the end of the deglaciation event (over 1 ka). The observed vertical offset in Unit C6 at Heiðarbrekka is of this order of magnitude (4 m).
Evidence on the western side of the Hekla fault system suggests that rifting events occurred during at least two deglaciations—the early MIS 6b deglaciation and the MIS 6a/MIS5e—such as at Ofærugill (Unit B2) and with the Plinian eruption close to 128 ka. These events resulted in sagging, in the form of a hemi-graben, toward the embayment (Fig. 1C). This partly explains the preferential preservation of the interglacial formation on this side of the embayment. It probably also occurred during Termination Ib, enabling the flow of lava (Þjὀrsá Lava) and jökuhlaup along the eastern coast of the embayment, excavating the Rangá valley. This arrangement in asymmetric grabens has also been observed in Holocene formations of the Bárðarbunga–Veiðivötn system (Fig. 1B), and seems to be a classic feature of the Holocene volcanic systems of the EVZ, with fault offsets ranging between 4 and 22 m (Plateaux, Reference Plateaux2012). To the west, along Kleifarvatn, fault-offset strikes follow the Reykjanes fault system (Clifton and Kattenhorn, Reference Clifton and Kattenhorn2006).
Large interglacial earthquakes in the Ytri Ranga valley
Glacial unloading temporarily modified the stress field, allowing the opening of fractures in the SISZ and South Volcanic Zone during interglaciations. During the onset of the last Bárðarbungá eruption (Guðmundsson et al., Reference Guðmundsson, Lecoeur, Mohajeri and Thordarson2014), fissure opening was initially accompanied by a left-lateral shear that ceased with increased opening and magma injection. In the Northern Volcanic Zone (Jökulsà à Fjöllum valley), fissure opening occurred with the deposition of MIS 5e deglacial sediments (Van Vliet-Lanoë et al., 1995), before the settling of the Grímsvötn 5e-Low/BAS IV tephra (ca. 127 ka; Van Vliet-Lanoë et al., Reference Van Vliet-Lanoë, Schneider, Guðmundsson, Guillou, Nomade, Chazot, Liorziou and Guégan2018), and ended in the Mófell sector (north of Krafla Volcano), with lava fountains and spatter cones occurring inside the fissures.
The earthquake evidenced in synchronicity with the first MIS 5e regression in the YtrÍ Rangá valley (ca. 126–125 ka) was related to a rapid hydrostatic unloading downstream and to a rapid glacial loading upstream and associated with true ruptures in the SISZ (Fig. 6D) and also with eruptions in the Hekla system (Fig. 3). The accumulation of an extension deficit—responsible for large Holocene earthquakes—seems to have been primarily related to low water table levels in the eastern part of the SISZ during the drained full interglaciation, and secondarily to limited volcanic activity (dyke injection) in the west, the EVZ still being regularly active. Guðmundsson (Reference Guðmundsson2000) proposed that the Laki eruption in 1783 induced one of the largest historical earthquake in Iceland in 1784 (estimated magnitude 7.1). Because fluid injections (magma or water) into fissures are much less available during full interglaciations, it seems logical that the extension deficit would increase at that time. This could also be the case for early interstadials (MIS 5d, low water table) in regions with a delayed glaciation.
Most of the other factures are arranged en échelon, with the N–S orientation typical of the SISZ, organized in several fault arrays (e.g., Sigmundsson et al., Reference Sigmundsson, Einarsson, Bilham and Sturkell1995; Einarsson et al., Reference Einarsson, Böttger and Thorbjarnarson2002; Bergerat et al., Reference Bergerat, Homberg, Angelier and Bellou2011; Angelier et al., Reference Angelier, Bergerat, Bellou and Homberg2004). They are influenced by preexisting fractures (Ruch et al., Reference Ruch, Wang, Xu, Hensch and Jónsson2016), re-using faults activated during the MIS 5e and probably earlier. These were probably initiated during the first stage of the development of the SISZ, as shown by fractures that occur outside the present range of the SISZ, north of the Búrfell (Fig. 1C), at large angles to the main left-lateral shear and displaying an opposing sense of motion (i.e., right-lateral displacement; e.g., Bergerat and Angelier, Reference Bergerat and Angelier2008). Fracturing could also have allowed for a rapid breaking of the tectonic displacements, and thus a change in earthquake type, through rapid drainage of the upper crust, similar to the drainage that occurred at Kleifarvatn after the 2000 earthquake (Clifton et al., Reference Clifton, Pagli, Jónsdóttir, Eythórsdóttir and Vogfjörd2003).
Subglacial volcanism
Magma and water potentials
The observed recurrent microseismicity seems to be related in time to upper mantle decompression, with a deglaciation-forced basal reservoir and dyke injection along existing volcanic systems (Guðmundsson, Reference Guðmundsson2000; Hartley and Thordarson, Reference Hartley and Thordarson2013), not only with the thickness of the ice sheet as commonly stressed (Walker, Reference Walker1965; Licciardi et al., 1997). As inferred by numerical models by Andrew and Guðmundsson (Reference Andrew and Guðmundsson2007), the pressure on the magma reservoirs increases under glacial load, and so does the melt temperature, meaning there is less melt available under the glacial load (glacial periods) than during melting periods (early interglacial periods).
With a maximal ice load (ca. 1500 m of ice at the LGM), a crustal deflection of 500 m inland would be expected for a mean plateau altitude of around 600 m. Downbending generates horizontal compressive stress, which can stop dyke injections and inhibit eruptions (Guðmundsson, Reference Guðmundsson1986, Reference Guðmundsson1999; Andrew and Guðmundsson, Reference Andrew and Guðmundsson2007; Sinton et al., Reference Sinton, Grönvold and Sæmundsson2005; Sigmundsson et al., Reference Sigmundsson, Pinel, Lund, Albino, Pagli, Geirsson and Sturkell2010; Eason et al., Reference Eason, Sinton, Grönvold and Kurz2015). The present surface of the basaltic basement below the ice sheet will evolve into a flat or down-warped subglacial topography.
Downbending also implies a minimal magma overpressure to produce lava eruption, with the potentiometric surface being very low angled, making for easier dyke injection and eruption in zones where the ice load is less significant, such as at the margins of the ice cap (Fig. 8E) or at the thinned head of an ice stream. Moreover, due to unloading, the melting glacial margins will experience the largest stress increase (Pagli and Sigmundsson, Reference Pagli and Sigmundsson2008), with emission of extensive aerial lava flows (Guðmundsson, Reference Guðmundsson1986, Reference Guðmundsson2000) extending outside the ice sheet, often in the form of lava shield. In this case, the slope of the magma potential is much more important compared with major deglacial times, and probably a limited production of mantle melting would be enough to produce large lava splay, as at Holuhraun in 2014 or in the Ytrí Rangá in the early MIS 5e.
Furthermore, during early deglaciation, the isostasy-forced flat topography simultaneously aided in the formation of large, subglacial aquifers or event lakes at the ice-sheet base. It enhanced the effects of earthquakes (Sibson, Reference Sibson and Parnell1994; Mulargia and Bizzarri, Reference Mulargia and Bizzarri2014), and finally provoked basal sliding of the ice sheet, promoting glacial surges and accelerating glacial thinning.
Tuya and hyaloclastite ridge formation
Magma supply is key to all subglacial injections, shutting down once its pressure falls below the glaciostatic pressure (Smellie and Skilling, Reference Smellie and Skilling1994; Smellie, Reference Smellie2008). Guðmundsson (Reference Guðmundsson2011a, Reference Guðmundsson2011b) demonstrated that magma emergence is related to a complex combination of overpressure of the magma in the dykes, magma and crustal densities and effects of layering in the crust. Tuya and hyaloclastite ridges should develop under glaciers with an ice thickness being assumed to be sufficient (>500 m) to restrict volcanic explosivity to phreatomagmatic processes (Guðmundsson et al., 2004; Höskuldsson et al., Reference Höskuldsson, Sparks and Carroll2006; Smellie, Reference Smellie, Mcintosh, Esser and Fretwell2006; Edwards et al., Reference Edwards, Guðmundsson, Russell, Sigurdsson, Houghton, McNutt, Rymer and Stix2015), allowing the construction of a subaqueous tuff cone superimposed on the pillow lava volcano (Smellie, Reference Smellie and Sigurdsson2000; Tuffen et al., Reference Tuffen, Owen and Denton2010; Edwards et al., Reference Edwards, Guðmundsson, Russell, Sigurdsson, Houghton, McNutt, Rymer and Stix2015). This is observed in the southwestern part of the hyaloclastite ridge of Hellutindar. Conversely, the common basal accumulation of non-vesiculated pillow lava—the first step in building tuyas or ridges—is a result of high cooling rates from water at hydrostatic pressures of more than 600–700 m (Jones, Reference Jones1969; Aumento, Reference Aumento1971; Zimbelman and Gregg, Reference Zimbelman and Gregg2000; Russell et al., Reference Russell, Edwards, Porritt and Ryane2014), as in the Háuhnύkar Tindar, north of Kleifarvatn. The preliminary construction phase of the Hlöðufell tuya (240 m thick; Skilling, Reference Skilling2009) would have required a pressure equivalent to 740 m of ice thickness. These values are in agreement with a thinned ice sheet and some melting.
The ridges seem also to be predominantly related to greater magma injection than the tuyas, feeding on a preexisting wide volcanic system containing tension fractures, normal faults, and dykes (Guðmundsson, Reference Guðmundsson2000; Hartley and Thordarson, Reference Hartley and Thordarson2013; Rubin and Gillard, Reference Rubin and Gillard1998), as seen at Kleifarvatn, supplied by the Mid-Atlantic Ridge (Höskuldsson et al., Reference Höskuldsson, Hey, Kjartansson and Guðmundsson2007). Another system for ridge formation is likely magma injection mostly fed by a large, elongated reservoir at the base of the crust (Hartley and Thordarson, Reference Hartley and Thordarson2013; Guðmundsson et al., Reference Guðmundsson, Lecoeur, Mohajeri and Thordarson2014), rather than by a chamber, because fissure eruptions result from subvertical dykes rupturing the surface during rifting events and may occur close to or independent of the central volcano. This would explain the volume of such ridge emplacement during relatively brief rifting events, unlocked by glacial unloading and melting production at the base of the crust and, following our observation, near the ice-sheet margin (Fig. 8E). For tuyas, the homogeneity of their geochemistry suggests these are chamber-fed features (Eason et al., Reference Eason, Sinton, Grönvold and Kurz2015), probably initially driven by deeper, large, elongated reservoirs at the base of the crust (Hartley and Thordarson, Reference Hartley and Thordarson2013). Their formation is thus less sensitive to major unloading than the ridges, but could be more sensitive to local unloading.
Volcaniclastic interstratified tuff and basalt facies are good indicators of full or limited deglaciation episodes (Smellie, Reference Smellie2008), although ice thickness cannot be precisely constrained based on this lithofacies (Lachowycz et al., Reference Lachowycz, Pyle, Gilbert, Mather, Mee, Naranjo and Hobb2015). In the absence of dating, the development of hyaloclastite/pillow lava ridges has been considered to have mostly occurred around the time of the LGM at the ice divide (Carrivick et al., Reference Carrivick, Russell, Rushmer, Tweed, Marren, Deeming and Lowe2009; Bourgeois et al., Reference Bourgeois, Dauteuil and Van Vliet-Lanoë1998), but also during the deglaciation, especially tuya (cf. Licciardi et al., Reference Licciardi, Kurz and Curtice2007). From our data, tuyas and hyaloclastite ridges (Fig. 10; other ridges of southern Iceland, Supplementary Material, p. 12) seem mostly to have formed during MIS 6b and correspond to transitional periods during buildup phases of the ice sheet, temporary melting phases in interstadials, or even during full deglaciations.
Dating of tuyas and hyaloclastite ridges
As mantle production and hot-spot activity pre-dated the glaciation, they have certainly interfered with the impact of a full or a limited deglacial unloading and the chain of interactions that this has induced. The K–Ar ages obtained from the Hlöðufell and Rauðafell tuyas (Table 3, Supplementary material) are significantly older than the He-exposure ages obtained by Licciardi et al. (Reference Licciardi, Kurz and Curtice2007) and Eason et al. (Reference Eason, Sinton, Grönvold and Kurz2015).
Minor amounts of unresolved excess 40Ar can significantly bias K–Ar ages obtained from samples containing very small amounts of radiogenic Ar, as is the case in the K–Ar-dated samples from the Hlöðufell and Rauðafell tuyas and as discussed in Guillou et al. (Reference Guillou, Scao, Nomade, Van Vliet-Lanoë, Liorzou and Guðmundsson2019). Such excess Ar could explain the differences between the K–Ar and He-exposure ages. An alternative explanation is that the tuyas might be polygenic. Indeed, a single tuya may erupt several times during a single glaciation, such as the Snæfell (Höskuldsson and Imsland, Reference Höskuldsson and Imsland1998; Guillou et al., Reference Guillou, Van Vliet-Lanoë, Guðmundsson and Nomade2010). More recent tuya eruptions, such as the upper Herðubreið (Werner et al., Reference Werner, Schmincke and Sigvaldason1996), seem to correspond to the late reactivation of an older tuya related to the Herðubreiðtögl tuya (256 ± 66 ka; Guillou et al., Reference Guillou, Van Vliet-Lanoë, Guðmundsson and Nomade2010) and are clearly multiphasic. Eason et al. (Reference Eason, Sinton, Grönvold and Kurz2015) considered that most of the tuyas they investigated were monogenic, on the basis of uniformity in geochemical composition alone, which is insufficient evidence to support such a view. Step-like platforms on tuyas can also be formed by successive lava-fed hyaloclastite deltas (Smellie et al., Reference Smellie, Mcintosh, Esser and Fretwell2006). The dating of different units in a single tuya is crucial, but has yet to be done.
Plotting the ages of the subglacial activity from K–Ar dating (Table 3 Supplementary material) over the last 150 ka (Fig. 10), it appears, on the surface, that tuyas and hyaloclastite ridges mostly formed during ice-sheet buildup/loading phases that increased the primary mechanical vertical stress (σ1; Fig. 10), especially during the early glacial stages, but also blocked the dyke propagation. A more detailed analysis, however, indicates that volcanic activity occurred during both the interglaciations and the glaciations (Guillou et al., Reference Guillou, Van Vliet-Lanoë, Guðmundsson and Nomade2010). The high frequency of formation of tuyas and hyaloclastite ridges seems to have occurred at least twice—during the early glacial time, probably linked with large interstadials, and after the Ålesund interstadial (35–27 ka). This most likely resulted from rapid variations in ice thickness, as unloading events triggered by melting intervals such as DO events (Fig. 10) probably temporarily destabilized the magmatic chamber(s) (Höskuldsson et al., Reference Höskuldsson, Sparks and Carroll2006), although the ca. 10% accuracy of the K–Ar dating with low potassium content does not allow for a more precise chronology at this point, especially for the upper last glacial period. Relatively brief interstadial deglaciation events lasting for less than 5 ka during a period of ice-sheet reconstruction could also, and more logically, drive the formation of subglacial volcanoes (Fig. 10). A rapid ice-melt thinning of 100 m per century could be expected for DO events, given the present melting rate of the Vatnajökull, estimated at ca. 1 m/yr (https://www.glacierguides.is/vatnajokull-general-information, November 2018). Another thinning is expected from ice-stream formation, as presently seen on Victoria Land (Antarctica).
The MIS 6c glacial stage was cold and wet, and thus susceptible to the accumulation of a relatively very thick ice sheet, more significant than that of the previous glaciation (Fig. 10). Unloading, particularly during the MIS 6b interstadial (155–145 ka), was significant enough to generate ridges on the Kleifarvatn and Veiðivötn fault systems but also at the ridge of Kárahnjúkar (Sandfell) with emersion of the ice sheet (Jökuldalsheiði, west of Halslὀn lake; Table 3, Supplementary material). It is possible that the outer ridges of the Kverkfjöll, analyzed by Carrivick et al. (Reference Carrivick, Russell, Rushmer, Tweed, Marren, Deeming and Lowe2009), also correspond to MIS 6b, as these ridges crop at the same distance of the Vatna magma feeding sources, with limited reactivation during Termination I. The small Skarðsengi ridge in the North Volcanic Zone (northeastern Iceland) is more recent, but is also related to a limited deglaciation (80 ka, MIS 5a interstadial; Guillou et al., Reference Guillou, Van Vliet-Lanoë, Guðmundsson and Nomade2010). A computer-generated map of the Vatnajökull subglacial topography (Björnsson, Reference Björnsson2009) shows that many fewer ridges formed during the final deglaciation than in the Younger Dryas stadial at the terrestrial margins of the Weichselian ice sheet.
Tephra and glaciation
The drainage of a subglacial volcanic lake can further (re)activate explosive eruptions by lowering the pressure on the magma chamber (Höskuldsson et al., Reference Höskuldsson, Sparks and Carroll2006; Albino et al., Reference Albino, Pinel and Sigmundsson2010; Smellie, 2010). Tephra-emitting eruptions at Grímsvötn Volcano (Davies et al., Reference Davies, Abbott, Meara, Pearce, Austin, Chapman, Svensson, Bigler, Rasmussen and Farmer2014; Voelker and Haflidason, Reference Voelker and Haflidason2015) occurred relatively constantly throughout the last glaciation, were relatively irregular in the Veiðivötn-Bárðarbunga volcanic system, were more clustered at Hekla Volcano, and were apparently rare during the period of maximal ice-sheet load (Fig. 10).
Concerning Bárðarbunga, the tephra signal is quite clear, in relation to a 12-km-deep magmatic chamber. Activity was, based on the entire available tephra record (Holocene to early MIS 5e; Davies et al., Reference Davies, Abbott, Meara, Pearce, Austin, Chapman, Svensson, Bigler, Rasmussen and Farmer2014; Voelker and Haflidason, Reference Voelker and Haflidason2015; Guðmundsdóttir et al., Reference Guðmundsdóttir, Larsen, Björck, Ingólfsson and Striberger2016; Van Vliet-Lanoë et al., Reference Van Vliet-Lanoë, Schneider, Guðmundsson, Guillou, Nomade, Chazot, Liorziou and Guégan2018), limited during the MIS 5e, but persistent during the Weichselian and late-glacial times, probably due to a straightforward connection to a deep, large subcrustal reservoir. Activity was sustained during the major DO events, from 62 to 48 ka (Fig. 10), specifically the MIS 3 warmings and Heinrich event (HE) 6 (Marshall et al., Reference Marshall and Koutnik2006), just before a period of ice-cap thickening. The warmings were responsible for a thinning of the ice sheet, probably resulting from the development of ice streams (enhanced ice plasticity), as observed today in Antarctica (Rignot et al., Reference Rignot, Bamber, Van Den Broeke, Davis, Li, Van De Berg and Van Meijgaard2008), and calving, as proven by recorded IRD (Fig. 10). The present position of this volcano, on the western side of Vatnajökull, seems very susceptible to ice thinning, as has been seen recently. A second peak of activity developed from 35 to 27 ka in connection with HE 4 and a warming equating to the Ålesund interstadial (Norway; Mangerud et al., Reference Mangerud, Gulliksen, Larsen, Longva, Miller, Sejrup and Senstegaard1981). The final activity began ca. 18 ka, ending with Termination Ib, including HE 1 and the major warming of the Bölling interstadial (Fig. 10).
The Hekla volcanic system appears to have expanded during Termination IIb. This enabled volcanic activity to occur with early rhyolitic pyroclastic flows, ca. 129–128 ka, at the C2/C3 unit boundary, apparently at the onset of glacial thinning in the southern embayment and basaltic eruption close to the thermal optimum at ca. 127–126 ka, during the deposition of Units C4 and C5 (Galtalækur lava flow, 2 tephra; Fig. 3), synchronous with the end of a rapid glacio-isostatic rebound and with activity associated with the SISZ, as is discussed for the Holocene by Guðmundsson and Brenner (Reference Guðmundsson and Brenner2003). Following this, the activity became more episodic, even nonexistent, from 90 to 55 ka—the main period of ice-cap thickening with limited IRD production (Fig. 10). Similar to Bárðarbunga, activity at Hekla increased from MIS 3, after HE 6, persisting until Termination I (Fig. 10). Activity became quite regular due to Hekla's emergence, probably as a result of a thinning of the glacier via the ice streams that occupied the southern embayment. This volcano definitively pierced the ice sheet from ca. 55 ka, producing a good record of tephra emissions. As it is a fissure stratovolcano, a direct link to a subcrustal melt, driven by unloading, can be proposed from 55 ka. The Þórsmörk valley, north of the Myrdaljökull glacier, was also ice-free when thick tephra fell at ca. 55 ka (Torfjökull Volcano; Moles et al., Reference Moles, McGarvie, Stevenson, Sherlock, Abbott, Jenner and Halton2019). Today, Hekla Volcano reaches 1488 m asl, 400–500 m higher than the surrounding tuyas, and thus probably emerged to ca. 300 m above the maximal ice thickness (900 m) after 55 ka. It is logical, using these results, to propose an early collapse, by surging, of the southern Icelandic ice sheet from the H6 event. The marine core record from the Gardar Ridge, southwest of Iceland, shows a massive input of IRD and ashes from 48 ka (Ash Zone II; Haflidalson et al., Reference Haflidason, Eiriksson and van Kreveld2000).
CONCLUSIONS
In southern Iceland, late-glacial deposits and lava flows, the MIS 5e interglacial stage, and the Ófærugill/Hellutindar deglaciation event attest to six main unloading phases (MIS 6b, Terminations IIa, IIb, and IIc and Ia and Ib; Fig. 10). All of these were associated with synsedimentary seismic activity of low intensity, usually linked to dykes, ice-edge hyaloclastite ridge, and perhaps tuya injection. The occurrence of intrusive and extrusive volcanic activity during glacial unloading seems to have occurred commonly in association with dynamic extension, uppermost mantle decompression, magmatic fracturing, and volcanism. Glacial unloading seems to be the major trigger for surface geodynamics in glaciated volcanic provinces, even though surface geodynamics is primarily the triggered by deeper, mantle-driven rifting events, independent of glaciations. Also, high water levels, as commonly occur during deglaciations and maximal inundations (early interglaciations) likely eased the rifting by lowering the shear resistance close to the surface. From the record in the Ytrí Rangá long sequence, fault offsets in the WVZ and EVZ were more likely linked to deglacial unloading alone, unlocking local volcanic activity, even during short-lived events.
Evidence for a higher frequency of large earthquakes was not found in relation to deglaciation events, such as during the early MIS 5e and the Greenland interstadial GIS 25. Higher-magnitude seismic events appear to be linked to active N–S segments of the SISZ that also reactivated during the Holocene, and triggered by stress disequilibrium related to regional volcanic activity and drained conditions in the upper crust.
From the tephrostratigraphic records of marine and ice cores, the activity of the Grímsvötn Volcano seems to have been quite constant since MIS 5e. Fissure system activity seems to have been controlled by glacial unloading, with fault system enlargement favored by fracture opening related to unlocked rifting and major dyke injection. The activity of Hekla Volcano seems to have been sporadic since the middle Pleistocene. Fissural volcanic activity in the Bárðarbunga–Veiðivötn system was similar, but more constrained by the glacial unloading of the volcanic system.
ACKNOWLEDGMENTS
This study was funded by the French Polar Institute Paul-Emile Victor (IPEV), Arctic Research Program no. 316 (ICPROCI I, II, and III), and in part by the 4th and 5th programs of the European Union (PRENLAB 1 and 2, PREPARED) for fieldwork in Iceland. We especially thank Kristjan Sæmundsson for providing the original dating for the Krafla caldera. We also thank Audrey Wayolle and Guillaume Gosselin for their assistance during fieldwork in 2006 and 2008. This paper has benefited from helpful reviews and comments by Agust Guðmundsson (Royal Holloway), Kelly Russell, and an anonymous reviewer and from the editorial suggestions of N. Lancaster and J. Urrutia Fucugauchi.
SUPPLEMENTARY MATERIAL
The supplementary material for this article can be found at https://doi.org/10.1017/qua.2019.68