INTRODUCTION
The complex interactions between organisms and their environments are an important aspect of the planet’s evolution. Biotic and abiotic systems evolve in time and leave tracks in the biosedimentary record (e.g., Kowalewski et al., Reference Kowalewski, Wittmer, Dexter, Amorosi and Scarponi2015; Wyosocka et al., Reference Wysocka, Radwański, Górka, Bąbel, Radwańska and Złotnik2016; Martinelli et al., Reference Martinelli, Soto, González and Rivadeneira2017; Scarponi et al., Reference Scarponi, Azzarone, Kowalewski and Huntley2017a, Reference Scarponi, Kusnerik, Azzarone, Amorosi, Bohacs, Drexler and Kowalewski2017b). Unfolding such a record to pinpoint how ecosystems changed over time in response to paleoenvironmental modifications, however, requires a multidisciplinary approach including a well-established stratigraphic framework, a careful taxonomy, and a comprehensive ecological background (Dodd and Stanton, Reference Dodd and Stanton1990). The basic data for paleoecology are adequately identified body fossils and trace fossils, which record the behavioral patterns of organisms through time, both correctly positioned within the stratigraphic framework. Though such a multidisciplinary approach is widely recognized to be powerful in reconstructing paleoecosystems and their evolution, it is seldom implemented in the literature.
The lower Pleistocene marine succession of the Arda River (northern Apennines, Italy) represents an excellent site at which to apply multidisciplinary investigations (Crippa et al., Reference Crippa, Angiolini, Bottini, Erba, Felletti, Frigerio and Hennissen2016). The wealth of sedimentary structures and the excellent preservation of body and trace fossils make this marine succession a case study where we can integrate the abiotic and biotic components to resolve past ecosystems dynamics within a key time interval of climate change.
The early Pleistocene was characterized by climatic oscillations related to glacial/interglacial cycles, with the Mediterranean area being affected by these changes in both marine and continental settings (e.g., Bertini, Reference Bertini2010; Fusco, Reference Fusco2010; Scarponi et al., Reference Scarponi, Huntley, Capraro and Raffi2014; Combourieu-Nebout et al., Reference Combourieu-Nebout, Bertini, Russo-Ermolli, Peyron, Klotz, Montade and Fauquette2015; Crippa et al., Reference Crippa, Angiolini, Bottini, Erba, Felletti, Frigerio and Hennissen2016; von Leesen et al., Reference von Leesen, Beierlein, Scarponi, Schöne and Brey2017). The most important biotic events recorded in the marine environment of the Mediterranean are the disappearance of taxa of subtropical affinity and the occurrence of “northern guests,” i.e., organisms presently living at higher latitudes in the Northern Hemisphere, such as the bivalve Arctica islandica, the foraminifera Hyalinea balthica, and Neogloboquadrina pachyderma left-coiling, which migrated into the entire Mediterranean Sea through the Strait of Gibraltar during glacial periods since the Calabrian (early Pleistocene; Suess, 1883–Reference Suess1888; Raffi, Reference Raffi1986; Martínez-García et al., Reference Martínez-García, Rodríguez-Lázaro, Pascual and Mendicoa2015). Recently, by analyzing the isotopic composition of the bivalve shells from the Arda River section, Crippa et al. (Reference Crippa, Angiolini, Bottini, Erba, Felletti, Frigerio and Hennissen2016) observed that seawater temperature seasonality was the main variable of climate change within the study area during the early Pleistocene, in turn controlled by the Northern Hemisphere glaciation dynamics. In particular, strong seasonality and low winter paleotemperatures were assumed to be the main drivers for the widespread establishment of “northern guest” populations in the Paleo-Adriatic Sea.
Here, we pursue an integrated approach involving facies analyses and paleoecological observations to investigate the relationships between body and trace fossils and their environment in the early Pleistocene of the Arda Section. The purpose of this paper is twofold: first, to compare the results of the analyses of sedimentology and body and trace fossils, evaluating to which extent these three different approaches complement one another and derive general implications for their combined use; and second, to reconstruct the paleoenvironmental evolution of the Arda River sedimentary succession based on the integration of three different tools and to interpret the succession taking into account the interplay between tectonic and climatic factors (both local and global).
GEOLOGICAL SETTING
The Arda River section is located in northern Italy near the town of Castell’Arquato, at the margin of the northern Apennines facing the Po plain (Fig. 1). The northern Apennines are an orogenic wedge that started to form since the Oligocene as a result of the collision between the Corsica-Sardinia microplate and the Adria promontory, following the closure of the Mesozoic Ligurian-Piedmont Ocean (Carminati and Doglioni, Reference Carminati and Doglioni2012). Deformation migrated through time toward the east-northeast, gradually involving the Ligurid oceanic units and the Adria continental margin.
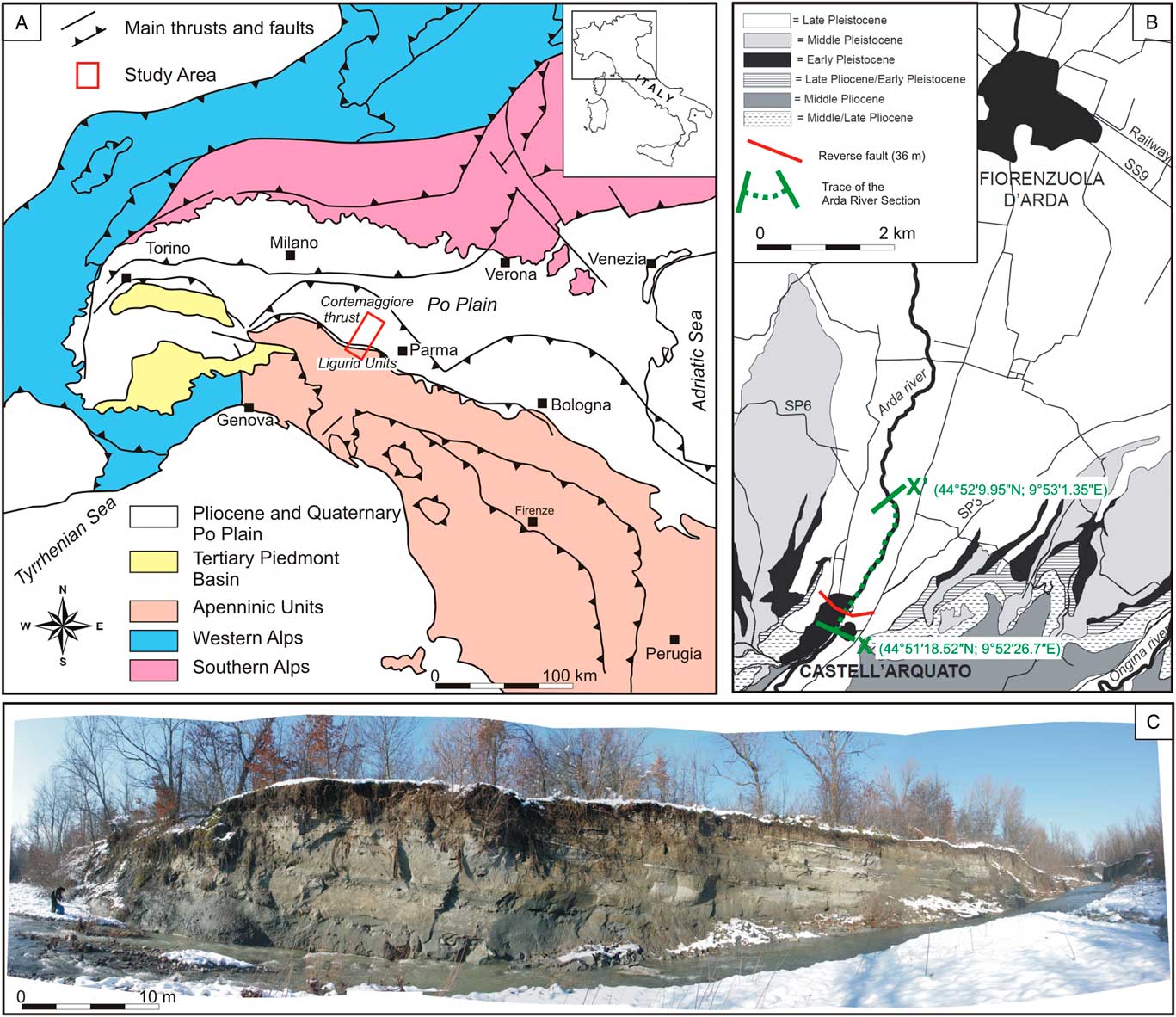
Figure 1 (color online) (A) Geological sketch map of northern Italy showing the study area (modified after Crippa et al., Reference Crippa, Angiolini, Bottini, Erba, Felletti, Frigerio and Hennissen2016). (B) Enlarged geological map of the Castell’Arquato basin with indication of the measured Arda River section (modified after Monesi et al., Reference Monesi, Muttoni, Scardia, Felletti, Bona, Sala, Tremolada, Francou and Raineri2016). (C) Photograph of a part of the outcrop along the Arda River section (metric interval on the stratigraphic section; Fig. 2, 120–140 m).
The Arda River section belongs to the Castell’Arquato basin, a small wedge-top basin developed since the Messinian (Miocene) at the eastern edge of the northern Apennine orogenic wedge as a result of the fragmentation of the Po Plain foredeep (Roveri and Taviani, Reference Roveri and Taviani2003; Artoni et al., Reference Artoni, Bernini, Papani, Rizzini, Barbacini, Rossi, Rogledi and Ghielmi2010; Fig. 1). It forms part of the northwestern extension of the Paleo-Adriatic Sea and is bounded to the north by the Cortemaggiore thrust and to the south by the emerged front of Ligurid units (Monegatti et al., Reference Monegatti, Raffi, Roveri and Taviani2001; Fig. 1A). The basin infill is characterized by several basin-wide, unconformity-bounded sedimentary cycles, recognized both on the outcrops and in the subsurface of the Po Plain and the central Adriatic Sea (Pieri, Reference Pieri1983; Ori et al., Reference Ori, Roveri and Vannoni1986; Ricci Lucchi, Reference Ricci Lucchi1986). The Castell’Arquato basin is filled by a sedimentary succession of late Messinian (upper Miocene) to Holocene age, organized in a large-scale transgressive-regressive cycle controlled by tectonics, with beds forming a regular monocline dipping towards the north-northeast (Monegatti et al., Reference Monegatti, Raffi, Roveri and Taviani2001). The basal part of the filling succession comprises deep sea sediments postdating the Messinian salinity crisis (Ceregato et al., Reference Ceregato, Raffi and Scarponi2007; Calabrese and Di Dio, Reference Calabrese and Di Dio2009), when marine conditions were restored in the Mediterranean Sea; upward, they pass into slope and shelf facies associations and then through a regressive trend to the middle Pleistocene continental alluvial deposits, which represent the final retreat of the sea in this area and the establishment of a continental environment with vertebrate faunas and freshwater mollusks (Cigala Fulgosi, Reference Cigala Fulgosi1976; Pelosio and Raffi, Reference Pelosio and Raffi1977; Ciangherotti et al., Reference Ciangherotti, Crispino and Esu1997). Detailed studies carried out in recent years through the integration of surface and subsurface data have resulted in a comprehensive stratigraphic and evolutionary model of the Castell’Arquato basin during the Pliocene and the early Pleistocene (Roveri et al., Reference Roveri, Visentin, Argnani, Knezaurek, Lottaroli, Rossi, Taviani, Trincardi and Vigliotti1998; Monegatti et al., Reference Monegatti, Raffi, Roveri and Taviani2001; Roveri and Taviani, Reference Roveri and Taviani2003). Insights into the paleogeography of the Po basin at the first major regression of the coastline, a consequence of the lowstand during the late early Pleistocene climate turnover (EPT), have been recently provided in figure 8 of Monesi et al. (Reference Monesi, Muttoni, Scardia, Felletti, Bona, Sala, Tremolada, Francou and Raineri2016).
The studied succession crops out along the Arda River and extends downstream of the bridge located at the entrance of the town of Castell’Arquato (northern Italy; Fig. 1B and C); the marine part, which is the subject of the present study, is 237m thick and bounded at the top (44°52'9.95''N, 9°53'1.35''E) by continental conglomerates (Fig. 2). The lowermost portion of the section is cut by a fault (Fig. 1B and 2), causing the repetition of the first 36 m of the succession (base at 44°51′18.52″N, 9°52′26.7″E); the succession described here begins stratigraphically above the fault. The Arda River marine succession has a Calabrian (early Pleistocene) age, ranging from ~1.8 to 1.2 Ma, based on magnetostratigraphy (Monesi et al., Reference Monesi, Muttoni, Scardia, Felletti, Bona, Sala, Tremolada, Francou and Raineri2016) and calcareous nannofossil and foraminifera biostratigraphy, which allowed identification of three nannofossil (CNPL7, CNPL8, and CNPL9) and one foraminiferal (Globigerina cariacoensis) biozones (Crippa et al., Reference Crippa, Angiolini, Bottini, Erba, Felletti, Frigerio and Hennissen2016).

Figure 2 (color online) Detailed stratigraphic log of the Arda River section. Facies codes are listed in Table 1.
MATERIALS AND METHODS
Sedimentology
The Arda River section has been measured bed-by-bed at 1 cm resolution. Bed thickness measurements were performed mainly with a Jacob’s staff (e.g., a 1.5-m-high rod equipped with a clinometer and a flat sighting disc on top). The log was measured, recording the internal subdivisions of the individual beds or “depositional intervals” (i.e., depositional divisions or bed intervals in the sense of Ghibaudo [Reference Ghibaudo1992]). The thickness, grain size, presence of erosion surfaces, mud clasts, and structure of every internal division of the beds were recorded separately for each bed in addition to the total bed thickness. The grain size was measured using a grain-size comparator chart. In order to account for amalgamation of beds, partially amalgamated beds were measured as individual layers. This was possible through detecting the subtle grain-size breaks that are associated with amalgamation surfaces. To make the measurement of the section reproducible and available also for other analyses, labeled nails were fixed every meter from the base to the top of the section, according to the attitude of the bedding.
Body fossils
Fossils were collected bed by bed, from a total of 144 beds over the entire studied section; for each of the targeted beds, two rectangles (50 cm wide and 10 cm tall) were delimitated and all exposed fossils sampled. Fossil specimens (mainly mollusks) were then washed and cleaned from the encasing sediment using an air drill, in the case of hard sediment, or a scalpel, in the case of soft sediment, and a unique ID was assigned to each of them; they were identified at generic and/or specific level (where feasible) based on relevant literature (e.g., Williams et al., Reference Williams, Brunton, Carlson, Alvarez, Ansell, Baker and Bassett2000, Reference Williams, Brunton, Carlson, Alvarez, Ansell, Baker and Bassett2006; Ceregato et al., Reference Ceregato, Raffi and Scarponi2007; Crippa and Raineri, Reference Crippa and Raineri2015) and subsequently counted (see Supplementary Information A1 for a detailed explanation). In addition, to aid environmental interpretation of fossil assemblages, it was observed if the specimens (bivalves and brachiopods) were articulated or disarticulated and each specimen retrieved was carefully inspected, looking for the presence of the following taphonomic features: (1) roundness vs. sharpness of fragments; (2) corrasion, due to the combined effect of abrasion and dissolution (Brett and Baird, Reference Brett and Baird1986); (3) external and/or internal bioerosion, produced by predators, necrophages, or the presence of domichnia; (4) internal and/or external encrustations caused by episkeletobionts (sensu Taylor and Wilson, Reference Taylor and Wilson2003); (5) ornamentation, which is usually fragile and can be lost during post-mortem processes; and (6) original color and pattern of the shell/fragment surface (Supplementary Information A2 and Supplementary Table A1). Based on the analysis of these features, we identified the associations defined by Brenchley and Harper (Reference Brenchley and Harper1998): (1) life assemblages, (2) neighborhood assemblages, and (3) transported assemblages.
A qualitative paleoecological analysis was then performed, in which the assemblages of the Arda River section were tentatively compared to the recent Mediterranean biocenoses. As the majority of the retrieved taxa is represented by living species, the fossil associations recovered were grouped in biofacies and attributed to the present day depositional environments based on the occurrence of characteristic taxa described by Pérès and Picard (Reference Pérès and Picard1964). The abbreviation used are: SFBC, biocenosis of fine-grained well sorted sands; SFS, biocenosis of shallow water fine-grained sands; DC, biocenosis of coastal detritic bottoms; and VTC, biocenosis of terrigenous mud.
Trace fossils
Sedimentology and body fossil paleontology have been integrated with ichnological analysis, using the workflow for integrated facies analysis (McIlroy, Reference McIlroy2008). Because of the predominantly vertical outcrops and the high bioturbation intensity, the ichnofabric approach has been used. The ichnofabric analysis method considers the overall texture of a bioturbated sediment and, as such, it is the ichnological equivalent of facies analysis (Taylor et al., Reference Taylor, Goldring and Gowland2003; McIlroy, Reference McIlroy2004, Reference McIlroy2008).
Data collection consisted of recording the ichnofabric attributes of the Arda River section in the field at regularly spaced intervals (“samples”). Each of the studied samples was approximately 25 cm thick and the spacing between successive samples was 1 m. The recorded ichnofabric attributes are: (1) primary sedimentology (Taylor et al., Reference Taylor, Goldring and Gowland2003); (2) degree of bioturbation, quantified by the ichnofabric index (ii) methodology (Bottjer and Droser, Reference Bottjer and Droser1991); and (3) components of the ichnofabric, including either distinct trace fossils or biodeformational structures with indistinct outlines (Schäfer, Reference Schäfer1956; Wetzel and Uchman, Reference Wetzel and Uchman1998; Taylor et al., Reference Taylor, Goldring and Gowland2003). Relative abundance, burrow size, tiering, trace fossil frequency, and distribution at the sample scale have also been observed (Bromley, Reference Bromley1996; Taylor et al., Reference Taylor, Goldring and Gowland2003; Gingras et al., Reference Gingras, MacEachern and Dashtgard2011).
Visual analysis of each sample included observation of (1) the weathered surface of the outcrop; (2) the fresh surface of the outcrop, exposed with a trowel (a minimum of three fresh surfaces of about 25×25 cm have been observed); and (3) the enhanced fresh surface, obtained by dropping water on the fresh surface to enhance color contrast and to differentiate weathered traces from the surrounding sediment.
Each sample has been attributed to an ichnofabric class that has been distinguished on the basis of the degree of bioturbation, bioturbation distribution (Gingras et al., Reference Gingras, MacEachern and Dashtgard2011), diversity (i.e., the number of ichnotaxa present; Bromley, Reference Bromley1996), and components of the ichnofabric.
RESULTS AND INTERPRETATIONS
Facies analysis
Facies analysis was carried out on marine sediments deposited during phases of advance of fan deltas when Apennine tectonic uplift renewed sediment dispersal and provided the basin with a steeper margin. Following the genetic classification scheme proposed by Zavala et al. (Reference Zavala, Arcuri, Di Meglio, Gamero Diaz and Contreras2011) for flood-generated delta-front lobes, the deposits of the Arda section (Fig. 2) have been grouped in three main facies categories related to the three main processes that characterize sustained hyperpycnal discharges (i.e., hyperpycnal flows; Mutti et al., Reference Mutti, Tinterri, Di Biase, Fava, Mavilla, Angella and Calabrese2000; Tinterri, Reference Tinterri2007) in marine settings: (1) bed load (Facies B: bed-load related sedimentary facies); (2) turbulent suspension (Facies S: suspended-load-related sedimentary facies); and (3) lofting (Facies L: lofting-related sedimentary facies). Facies codes and relative descriptions are shown in Table 1.
Table 1 Facies classification scheme utilized for the Arda section following the genetic classification proposed by Zavala et al. (Reference Zavala, Arcuri, Di Meglio, Gamero Diaz and Contreras2011). All these facies categories are genetically related and occupy a definite position within the hyperpycnal system. The B facies is diagnostic of proximal areas and progressively disappears as the flow enters the area of lobe deposition. The S facies is the consequence of the loss of flow capacity and is typical of the medium to distal parts of the system. The L facies results from the flow inversion, which is diagnostic of flow-margin areas (both down the depositional axis and laterally along the axis).

Facies related to bed-load processes (Facies category B)
Facies category B (Fig. 3) is composed of massive (GmE; Fig. 3A) and cross-stratified (or crudely stratified) conglomerates (Gp; Fig. 3D) with abundant coarse- to fine-grained sandstone matrix (matrix supported). Large clasts in this facies appear to float in a medium- to coarse-grained sandstone matrix. Individual sets of cross bedding commonly show thicknesses between 0.1 and 0.5 m. The foreset inclination in general does not exceed 15°. Bounding surfaces between bedsets can be erosional. Lag deposits (Lag; Fig. 3E and F) represented by gravel carpets with bioclastic and sandy (very coarse) matrix are frequent. This facies category also includes mud clast-supported conglomerate (GmM; clay-chips; Fig. 3A–C). Mudstone intraclast diameter ranges from 0.5 to 20 cm and their shapes range accordingly from rounded sub-spherical to rounded tabular.

Figure 3 (color online) (A–F) Facies related to bed-load processes (Facies B). This category includes different coarse-grained deposits related to shear/drag forces exerted by the overpassing long-lived turbulent (hyperpycnal) flow over coarse-grained material lying on the flow bottom. See Table 1 for facies codes and relative description. Corresponding metric intervals on the stratigraphic section (Fig.2): (A) 179.10 m; (B) 114 m; (C) 94.10 m; (D) 28.20 m; (E) 224.80 m; and (F) 131.90 m.
Interpretation
This facies category includes different coarse-grained deposits related to shear/drag forces exerted by the overpassing long-lived turbulent (hyperpycnal) flow over coarse-grained materials lying on the flow bottom (Fig. 3). High-density flows triggered by river floods can mix and deposit skeletal remains from different shallow-water communities. Regardless, accumulations of shells are rare features in this bed-load related sedimentary facies.
Facies related to the collapse of suspended load (facies category S)
Facies category S (Fig. 4) are mostly fine-grained sandstone strata ranging from lamina-sets a few millimeters thick, to beds and bedsets several tens of decimeters thick, with massive stratification (Sm; Fig. 3E and F); horizontal (Sh; Fig. 4A and D) or hummocky cross stratification (HCS; Fig. 4E); tabular and oblique cross stratification (Sp, Sx; Fig. 4B); and small-scale cross lamination (Sr, St; Fig. 4A and C). Many beds are sharp-based and fine upward, with structures ranging from horizontal or large-scale wavy lamination to small-scale cross lamination (wavy, sigmoidal, and/or climbing ripple structures). Small floating clay chips are common and are dispersed within the sandstone body or grouped toward the top.

Figure 4 (color online) (A–F) Facies related to the collapse of suspended load (Facies S). This category mostly includes fine-grained sediments transported as suspended load, forming thick and commonly complex intervals that can be massive or display traction plus fallout sedimentary structures. See Table 1 for facies codes and relative description. Corresponding metric intervals on the stratigraphic section (Fig.2): (A) 177.20 m; (B) 134 m; (C) 193.80 m; (D) 230.60 m; (E) 184.60 m; and (F) 194.20 m.
Accumulations of shells are common features in the sandstone intervals, where they form thick, sharp-based, sometimes normally graded, lags at the base of massive (Sm; Fig. 3E and F) or laminated strata (Sp, Sx, Sr; Fig. 4A–C). Shell-bed geometry ranges from tabular to lenticular; shells are always closely packed, mostly concave-down, and sometimes imbricated. In many cases, tabular, sharp-based shell beds (10–50 cm thick and broadly lenticular) are found at the base of horizontal or hummocky cross-stratified (HCS) beds. Carbonaceous remains and wood fragments (commonly leaves with exceptional preservation) are also common within massive sands. Sedimentary structures are rarely disrupted by bioturbation, but bedtops may be 100% bioturbated. Flasers and massive mudstone beds (HeB), a few millimeters to several centimeters thick, are often intercalated with the sandstones.
Interpretation
This facies is mostly composed of fine-grained sediments transported as suspended load, forming thick and commonly complex intervals that can be massive or display traction plus fallout sedimentary structures.
Facies related to flow lofting (facies category L)
Facies category L (Fig. 5) is characterized by thin couplets of massive to laminated siltstones and mudstones (Fm; Fig. 5A). The individual levels commonly display a variable thickness from a few mm up to 100 cm. Marine bivalves in life position are present in the lower part of the stratigraphic section.

Figure 5 (color online) (A) Facies related to flow lofting (Facies L). It is characterized by thin couplets of massive to laminated siltstones and mudstones (Fm), transported by the hyperpycnal flow, which accumulated by normal settling when the flow completely stopped (107.40 m). (B) Cemented levels, rich in carbonate (CCB; 130.20 m). See Table 1 for facies codes and relative description. (C) Erosional bedset-bounding surfaces (114 m). (D) Rapid accumulation results in the common occurrence of water-escape features such as load cast and flame structures (184.60 m). (E and F) Biocalcarenitic body (46 m). It forms prograding wedges typically displaying intense bioturbation. Foresets and bottomsets are characterized by dense accumulations of reworked shells.
Interpretation
This facies is composed of the finest materials transported by the hyperpycnal flow, which accumulated by normal settling when the flow completely stopped.
Facies association
On the basis of high-resolution stratigraphic framework, the marine sedimentary succession up to 217 m is characterized by at least six (labeled I–VI in Fig. 2), 5- to 25-m-thick, fining upward cycles deposited by high-density turbidite flows (hyperpycnal flow, sensu Mutti et al., Reference Mutti, Tinterri, Di Biase, Fava, Mavilla, Angella and Calabrese2000) that grade into hemipelagic siltstones and mudstones (Fig. 2). These cycles are often very complex, showing internal erosional surfaces and gradual facies recurrences.
Each cycle usually starts with massive to cross-bedded conglomerates (GmE, GmM, Gp, or Lag; Table 1) with abundant bioclastic and sandy matrix. Intraclasts and accumulations of shallow-water skeletal remains are also present. Bedset-bounding surfaces can be erosional (Fig. 5C). Above follow sandstones that are either massive or with horizontal, hummocky, tabular, or oblique cross stratification (Sp, Sx, HCS, St, Sr, Sh, or Sm; Table 1). Sharp-based, normally graded, tabular to lenticular lags of concave-down shells may occur at the base of the strata. Carbonaceous remains and wood fragments are also common within massive sands. Flasers, wavy and lenticular bedding (Heb; Table 1), from a few millimeters to several centimeters thick, are often intercalated with these sandstones. Finally, above the sandstones follow massive to laminated siltstones and mudstones arranged in thin couplets (Fm; Table 1), ranging in thickness from a few mm up to 1 m and accumulated by normal settling when the flow completely stopped.
Each cycle shows a complicated internal arrangement. The vertical and lateral facies anisotropy and the relatively rapid accumulation result in the common occurrence of water-escape features such as load cast and flame structures (Fig. 5D). Field observations suggest a close association of this facies with channel fill deposits.
A biocalcarenite body occurs (Fig. 5E and F) 45 m from the base of the succession. It forms basinward-prograding wedges composed of alternating well- or poorly cemented layers with dense accumulations of reworked shells. It typically displays a tripartite geometry, whose topset horizontal strata are intensely bioturbated, and may contain abundant articulated bivalves and fragmented calcareous algae, while foresets and bottomsets are characterized by dense accumulations of reworked shells.
From 130 to 132 m, very fine-grained limestones form lenticular or pinching and swelling beds up to 0.25 m thick (Fig. 5B). The beds have sharp bases and tops and may be laminated. The thin section analysis reveals that these beds are predominately composed of a fine-grained calcareous matrix including calcareous microspheroids and organic matter.
From 217 to 237 m, the sequence is characterized by coarse-grained sands and pebbly sandstones with low-angle cross-stratification (Sx, Sh, St, Sm, HCS, and Heb; Table 1). Large clasts appear floating in a medium- to coarse-grained sandstone matrix. Individual sets of cross-beds (Sx, St) commonly show thicknesses between 0.3 and 1 m and asymptotic relationships with top and base. The foreset inclinations do not usually exceed 20°. This facies association is attributed to littoral (transitional) environments.
From 237 to 300 m, the sequence comprises continental sediments arranged in four main cycles, each characterized by massive or crudely stratified, partially cemented, fluvial gravel beds from 1 to 4 m thick (GmE, Gp; Fig. 2, Table 1), passing rapidly upward to sands, silts, and muds packed in beds from few meters up to 15 m thick. In situ root systems and tree trunks, together with CaCO3 nodules and typical terrestrial gastropods (e.g., Pomatias elegans, Carychium tridentatum, and Retinella [Retinella] olivetorum) are abundant in fine-grained beds, suggesting continental swamp environments.
Interpretation
Deposits of the Arda section display a complicated vertical arrangement of different lithofacies reflecting cyclic depositional changes between suspended-load and bed-load-dominated facies associated with different velocity and fallout rates. These cyclical and gradual changes between different facies are the result of near-continuous deposition from a quasi-steady turbulent flow (Zavala et al., Reference Zavala, Arcuri, Di Meglio, Gamero Diaz and Contreras2011). Distinctive features observed in the studied deposits are: (1) the sharp based and normally graded beds containing HCS; (2) gradual and sharp facies transitions without a definite and predictable internal arrangement; (3) the abundant rip-up mudstone clasts and shells; (4) internal and laterally discontinuous erosional surfaces; (5) scarce burrows; and (6) a basal coarsening-upward interval (Mutti et al., Reference Mutti, Tinterri, Di Biase, Fava, Mavilla, Angella and Calabrese2000; Zavala et al., Reference Zavala, Ponce, Drittanti, Arcuri, Freije and Asensio2006, Reference Zavala, Arcuri, Di Meglio, Gamero Diaz and Contreras2011). The studied deposits evolve laterally into packages of lofting rhythmites. These features are most likely related to bed-load processes developed at the base of a hyperpycnal flow (i.e., long-lived turbulent flow) and tend to dominate the proximal to medial parts of a river-delta system.
The biocalcarenite body occurring 45 m from the base of the succession shows an internal geometry suggesting that biocalcarenitic deposits are formed during periods of decreased input of fine-grained terrigenous sediments (or sediment starved conditions) whose fossiliferous content indicates high-energy levels in the shelf environments. Different physical conditions can be assumed for their formation, such as reduced terrigenous input or strong bottom reworking by currents. Massari and Chiocci (Reference Massari and Chiocci2006) describe the formation of very similar Pliocene-Quaternary basinward-prograding biocalcarenite wedges (detached from the shore and below the storm-wave base) along the submerged margins of the Mediterranean area by means of processes of sediment reworking from a nearshore by pass zone and of storm-driven down-welling flow. The cyclical nature of these biocalcarenites, observed in the Castell’Arquato basin (Stirone section, Cau et al., Reference Cau, Taviani, Manzi and Roveri2013, Reference Cau, Roveri and Taviani2017), has been hypothesized to be orbitally controlled by obliquity and/or precession cyclicity.
According to Mutti et al. (Reference Mutti, Tinterri, Benevelli, Di Biase and Cavanna2003), the Arda River succession can be interpreted as a flood-dominated, fan-delta system accumulated in roughly tabular lobes extending from alluvial conglomerates to shelfal siltstone and mudstone. The associate deposits (hyperpycnites; Mulder et al., Reference Mulder, Syvitski, Migeon, Faugéres and Savoye2003) are closely related to direct fluvial discharge. Observed facies associations clearly show as flood-generated dense flows enter seawaters as catastrophic and inertia-dominated relatively unconfined flows. Coarser materials tend to accumulate at the front of the flow, giving way to a horizontally negative grain-size gradient. Sedimentation occurs mainly in a mouth bar (characterized by sigmoidal bedding) and in associated flood-generated delta-front sandstone lobes.
This study suggests that the Arda River succession corresponds to the subaqueous extension of a fluvial system. It originated when the river in flood directly discharged a sustained (Carruba et al., Reference Carruba, Casnedi and Felletti2004; Felletti et al., Reference Felletti, Carruba and Casnedi2009) and relatively denser turbulent mixture of fresh water and sediments (hyperpycnal flows; Bates, Reference Bates1953) into the receiving standing body of water. This system extended for kilometers away from the river mouth and developed a predictable path of genetically related facies (Browne and Naish, Reference Browne and Naish2003; Mancini et al., Reference Mancini, Moscatelli, Stigliano, Cavinato, Marini, Pagliaroli and Simionato2013; Marini et al., Reference Marini, Milli, Rossi, De Tomasi, Meda and Lisi2013; Milli et al., Reference Milli, Mancini, Moscatelli, Stigliano, Marini and Cavinato2016; Bruno et al., Reference Bruno, Amorosi, Severi and Costagli2017) during its travel basinward.
Body Fossils
The fossil associations of the Arda River succession contain a very diversified fauna composed mainly of several species of bivalves and gastropods; brachiopods, corals, serpulids, echinoderms, scaphopods, and barnacles also occur. The associations have been grouped into 11 biofacies, based on the presence of key species, taphonomic evaluation (Supplementary Information A2 and Supplementary Table A1), and (paleo)ecology of the fossils recovered (Fig. 6A–F, 7A–F, 8A–N).

Figure 6 (color online) Fossiliferous beds of the Arda succession. (A) Articulated specimens of Glycymeris inflata (42 m, Biofacies 1, Cycle 0). (B) Section of an articulated specimen of Pinna sp. (54.20 m, Biofacies 10, Cycle I). (C) Articulated specimen of Pelecyora brocchii in life position (57.35 m, Biofacies 2, Cycle I). (D) Echinoid specimen (58.35 m, Biofacies 2, Cycle I). (E) Shell accumulation mainly containing specimens of Spisula subtruncata (68.53 m, Biofacies 4, Cycle I). (F) Life assemblage of articulated specimens of Glycymeris insubrica (133.90 m, Biofacies 9, Cycle IV).

Figure 7 (color online) Fossiliferous beds of the Arda succession. (A) Bed with several specimens of Aequipecten opercularis (134.35 m, Biofacies 9, Cycle IV). (B) Articulated specimen of Arctica islandica in life position and lacking internal sediment filling (224.30 m, Biofacies 6, Cycle VII). (C) Specimens of Chamelea gallina with empty valves in life position (234 m, Biofacies 5, Cycle VI). (D) Accumulation bed with mainly disarticulated bivalves (234.40 m, Biofacies 5, Cycle VII). (E) Articulated specimens of Glycymeris insubrica (235.70 m, Biofacies 5, Cycle VII). (F) Accumulation bed with articulated specimens of Glycymeris insubrica, representing the most abundant species at the top of the section (236.80 m, Biofacies 5, Cycle VII).
Biofacies 1
This biofacies occurs in fine-grained massive siltstones and sandstones (Facies L) at the base of the section (37.05–43.25 m). It is composed mainly of seminfaunal/infaunal organisms and by cemented/byssate epifaunal taxa; many rheophilous taxa (e.g., Astarte fusca, Glycymeris inflata, Glycymeris glycymeris, and Clausinella fasciata) are present (Fig. 6A, 8A, C, D). The taphonomic preservation is generally good (Supplementary Information A2 and Supplementary Table A1).

Figure 8 Taphonomic conditions of the body fossils; two views are shown for each specimen: external or internal for bivalves, abapertural or apertural for gastropods. A) Glycymeris inflata, left valve; note the encrustation made by bryozoans and serpulids (ACG29bis-3, Biofacies 1, Cycle 0); B) Pitar rudis, right valve; note the bioerosion hole reaching the internal part of the valve (ACG106, Biofacies 7, Cycle IV); C) Aequipecten opercularis, right valve; the valve is encrusted by serpulids only in the external part (ACG27bis-6, Biofacies 1, Cycle 0); D) Naticarius stercusmuscarum, showing preserved original color pattern (ACG29bis-20, Biofacies 1, Cycle 0); E) Peronidia albicans, right valve; the finer ornamentation and the color pattern are preserved (ACG53-4, Biofacies 4, Cycle I); F) Flexopecten glaber, left valve; the finer ornamentation is preserved (ACG80-4, Biofacies 4, Cycle II); G) Acanthocardia tuberculata, right valve; note the attempt of a bioeroding organism to perforate the shell (ACG94, Biofacies 9, Cycle IV); H) Aequipecten opercularis, right valve; the finer ornamentation is well preserved (ACG104-2, Biofacies 7, Cycle IV); I) Dosinia lupinus, right valve; note the original color pattern (ACG235, Biofacies 10, Cycle VI); J) Aequipecten opercularis, left valve; the shell is encrusted by bryozoans both in the interior and exterior (ACG238, Biofacies 10, Cycle VI); K) Glycymeris insubrica, left valve; the original color pattern is preserved (ACG197-5, Biofacies 10, Cycle V); L) Flexopecten glaber, right valve; the bioerosion does not perforate the entire thickness of the shell (ACG250, Biofacies 5, Cycle VII); M) Dosinia lupinus, right valve; hole made by bioeroding organisms (ACG201, Biofacies 10, Cycle V); N) Callista chione, right valve; note the preservation of the glossy periostracum (ACG197, Biofacies 10, Cycle V).
Interpretation
The occurrence of disarticulated rheophilous shelf related taxa indicates neighborhood assemblages of a high-energy environment winnowed by currents in an offshore transition setting. The negligible presence of shoreface key taxa (Chamelea gallina, Spisula subtruncata, and Acanthocardia tuberculata) showing high taphonomic degradation suggests a transport from shallower settings.
Biofacies 2
This biofacies occurs in fine-grained massive silty to muddy lithologies (Facies L) below and above the biocalcarenitic body (44.90 m; 47.35–49 m; 56.35–58.35 m); it is mainly represented by few specimens of seminfaunal/infaunal species typical of muddy-detritic and muddy shelf substrates (e.g., Venus nux, Turritella tricarinata pliorecens, Naticarius stercusmuscarum, and Pelecyora brocchii), showing excellent preservation (Fig. 6C, D 8E, F, Supplementary Information A2 and Supplementary Table A1). Corals, echinoids, brachiopods, and non-rheophilous mollusks are also present.
Interpretation
The sparse presence of V. nux and P. brocchii along with echinoids, brachiopods, and corals suggest a low-energy lower offshore transition setting characterized by normal marine salinity and oxygen conditions.
Biofacies 3
This biofacies occurs in biocalcarenite (45.65–46.05 m; Facies S) and is represented by sparse and poorly preserved valves of epifaunal mollusks: Aequipecten opercularis, Aequipecten scabrella, and Ostrea edulis (Supplementary Information A2 and Supplementary Table A1).
Interpretation
The sparse mollusk content associated with poor taphonomic preservation within coarse-grained deposits allows only a generic interpretation of a transported assemblage from relatively high-energy settings.
Biofacies 4
This biofacies occurs in fine-grained massive siltstones (59–70.02 m; 106.50–111.60 m; Facies L). Several key infaunal, shoreface to offshore transition, well-preserved taxa typical of muddy sands are found, specifically Glycymeris insubrica, A. tuberculata, C. gallina, S. subtruncata, and Neverita josephinia (Fig. 6E, 8E, F; Supplementary Information A2 and Supplementary Table A1).
Interpretation
The ecological and taphonomic signatures of this biofacies suggest life and neighborhood assemblages of shallow marine, high-energy offshore transition environments (Facies L); the faunal composition can be compared to the recent Mediterranean biocenosis of SFBC (Pérès and Picard, Reference Pérès and Picard1964).
Biofacies 5
This biofacies occurs in an alternation of fine-grained sandy to silty and muddy lithologies (217.90–223.20 m; 230.80–237 m; Facies S and B). It contains mainly semiinfaunal/infaunal taxa living in shallow water muddy sands (e.g., G. insubrica, A. tuberculata, C. gallina, Ensis ensis, Loripes orbiculatus, Cylichna cylindracea, Atlantella pulchella, and N. josephinia), together with species of upper shoreface (Donax spp.) and of wave-protected environments (Lucinella divaricate; Fig. 7C–F; 8L). Toward the top, G. insubrica becomes the most abundant species (Fig. 7E and F), with numerous articulated specimens found in life position in muddy beds; trunks and plant remains are also found. All the specimens are well preserved (Supplementary Information A2 and Supplementary Table A1).
Interpretation
Biofacies 5 indicates life and neighborhood assemblages of upper shoreface setting, due to the presence of key taxa Ensis ensis and Donax spp.; the faunal composition is comparable to the SFBC and SFS biocenoses of the recent Mediterranean Sea (Pérès and Picard, Reference Pérès and Picard1964). Seminfaunal/infaunal taxa are dominant, suggesting a high-energy shallow water environment, which may prevent the colonization by epifaunal species; the presence of species living in wave-protected environments, however, suggests a more heterogeneous substrate with quieter areas. In addition, toward the top of the section, the disappearance of stenohaline species and the increase of euryhaline taxa, suggests settings affected by river discharge. Indeed, G. insubrica, which can also thrive in low salinity settings (e.g., Malatesta, Reference Malatesta1974; Raineri, Reference Raineri2007; Crnčević et al., Reference Crnčević, Balić and Pećarević2013), becomes the dominant species in the upper part of the section. Biofacies 5 differs from Biofacies 4 by the presence of shallower water taxa (e.g., Donax spp.), which are absent in Biofacies 4.
Biofacies 6
This biofacies is found in fine-grained massive clayey horizon (224.20–224.30 m; Facies L), hosting several articulated specimens of Arctica islandica in life position and lacking sediment filling inside the valves (Fig. 7B), associated with the shallow water S. subtruncata, (average preferred depth=6.60 m, standard deviation =7.3 m; Wittmer et al., Reference Wittmer, Dexter, Scarponi, Amorosi and Kowalewski2014) both showing an excellent preservation (Supplementary Information A2 and Supplementary Table A1).
Interpretation
The co-occurrence of lower shoreface S. subtruncata and of the “northern guest” A. islandica suggests a lower shoreface/offshore transition setting.
Biofacies 7
This biofacies occurs in fine-grained massive silts and clays (Facies L; 146.55–167.90 m, 196–207 m). Seminfaunal/infaunal taxa living in muddy to silty lithologies are dominant. V. nux is the most abundant species in this interval, together with Turritella tricarinata pliorecens, Glossus humanus, Aporrhais pespelecani, Acanthocardia paucicostata, and Saccella commutata, all showing an excellent preservation (Fig. 8B, H; A2 and Table A1 in the Supplementary Information A2 and Supplementary Table A1).
Interpretation
The abundance of V. nux suggests a low-energy offshore transition setting that can be compared to the recent VTC biocenosis of the Mediterranean Sea (Pérès and Picard, Reference Pérès and Picard1964). According to Taviani et al. (Reference Taviani, Roveri, Impiccini and Vigliotti1997) and Dominici (Reference Dominici2001), the Venus nux assemblage lived at water depths of 20–40 m.
Biofacies 8
This biofacies (80.30–91.40 m; 101–104.10 m; 122.90–125.50 m; Facies L) comprises well-preserved epifaunal species of mainly muddy/sandy-detritic settings (e.g., Pecten jacobaeus, Aequipecten opercularis, Flexopecten flexuosus, and Pitar rudis), occurring together with mud-loving infaunal species (as V. nux and T. tricarinata pliorecens) where the mud content increases (Supplementary Information A2 and Supplementary Table A1).
Interpretation
This biofacies is characterized by many key species (e.g., P. rudis, F. flexuosus, and P. jacobaeus) belonging nowadays to the recent DC biocenosis (Pérès and Picard, Reference Pérès and Picard1964) together with VTC species where the mud content increases, all indicating life or neighborhood assemblages of offshore transition environments.
Biofacies 9
This biofacies is found in an alternation of fossil rich fine-grained sands/silts and mud barren of fossils (127.95–146.45 m; Facies S and B). The fauna consists of well preserved seminfaunal/infaunal species together with few epifaunal ones; taxa of shallow water muddy sands are abundant (e.g., G. insubrica, C. gallina, A. tuberculata, E. ensis, and Tritia mutabilis) together with few sandy/muddy-detritic species (A. opercularis, Laevicardium oblongum, and P. rudis; Fig. 6F, 7A, 8G, Supplementary Information A1 and Supplementary Table A1). Ditrupa sp. horizons are occasionally retrieved (e.g., ACG95; Supplementary Table A1).
Interpretation
This biofacies suggests neighborhood assemblages of shoreface settings, which thanks to the presence of G. insubrica, C. gallina, A. tuberculata, E. ensis, and T. mutabilis can be compared to SFBC biocenosis of the recent Mediterranean Sea (Pérès and Picard, Reference Pérès and Picard1964), although a few DC taxa (e.g., L. oblongum and P. rudis) are also found. Biofacies 9 is similar to Biofacies 5, although very shallow water taxa have not been identified here. The occasional presence of monotaxic beds of Ditrupa sp., usually thriving in turbid waters conditions (Dominici, Reference Dominici2001), suggests unstable and loose substrates.
Biofacies 10
This biofacies occurs in several sandstones beds of the succession within Facies S and B (54–54.20 m; 92.50–98.30 m; 170–194.10 m; 208.40–210.40 m) and is characterized by containing ecologically mixed and generally poorly preserved taxa (Fig. 8I, K, M, M; Supplementary Information A2 and Supplementary Table A1), often associated with clay chips and vegetal debris; an exception is given by well preserved, articulated specimens of Pinna sp. and infaunal echinoids. Seminfaunal/infaunal and epifaunal species of shelf muddy/sandy-detritic settings (e.g., L. oblongum, Timoclea ovata, P. rudis, and P. jacobaeus) are associated to shallower water species (e.g., A. pulchella, C. gallina, G. insubrica, and S. subtruncata) and mud loving taxa (e.g., Nucula placentina, T. tricarinata pliorecens, and V. nux).

Figure 9 (color online) Ichnofabric group 1. (A) Ophiomorpha ichnofabric. (B) Close-up of A, showing a specimen of Ophiomorpha, parallel lamination, and concave-up shells. (C) Ophiomorpha with a robust constructional lining, indicating high-energy conditions. (D) Macaronichnus ichnofabric. (E) Macaronichnus ichnofabric cross-cut by “lined light filled burrows” (Ophiomorpha?). (F) Intensely bioturbated Macaronichnus ichnofabric with a significant contribution from other undetermined ichnotaxa.

Figure 10 (color online) Ichnofabric group 2. (A) Few sharp burrows-smooth burrows ichnofabric. (B) Homogeneous suite represented by biogenic structures with poorly defined walls bioturbating a mud layer. (C) Sharp burrows suite represented by a sharp-walled trace that bioturbated a mud layer. (D) Sharp burrows-smooth burrows ichnofabric. (E) Close-up of D showing several “sharp-walled traces”. (F) Schaubcylindrichnus? (morphotype A). (G) Scolicid-sharp burrows ichnofabric. (H) Close-up of G, showing a scolicid and abundant vegetal debris.

Figure 11 (color online) Ichnofabric group 3. (A) Lockeia ichnofabric represented by numerous specimens of Lockeia; bedding plane view. (B) Ophiomorpha preserved in cemented layers. (C) Thalassinoides ichnofabric dominated by horizontal burrows filled by bioclastic sands. (D) “Columnar burrow set”. (E) Coarse-fill burrows ichnofabric overlain by sand-mud couplets pertaining to the ichnofabric group 2.
Interpretation
This biofacies, characterized by an ecologically mixed poorly preserved fauna of shoreface to offshore transition settings, represents mainly transported assemblages finally buried in an offshore transition setting as testified by articulated Pinna sp. and infaunal echinoids in life position (Fig. 6B); this suggests a high-energy setting, also indicated by the facies analysis (Facies S and B).
Biofacies 11
This biofacies groups species poorly preserved and ecologically mixed (e.g., A. tuberculata, A. opercularis, and V. nux) found within conglomerate beds (217.20 m; 223.80 m; Facies B; Supplementary Information A2 and Table A1).
Interpretation
The high taphonomic degradation along the different ecology of the species recovered reflects transport/reworking in a high-energy, coarse-grained, shallow marine settings.
Trace Fossils
The samples of the Arda River section have been attributed to 15 ichnofabric classes, that are grouped in four ichnofabric groups based on bioturbation intensity, distribution, and diversity (Table 2). Ichnofabric groups and classes were named according to the dominant feature and traces. Ichnotaxa have been identified at the ichnogenus level and open nomenclature has been used for difficultly identifiable traces. Readers are directed to Supplementary Table A2 for details on ichnotaxa.
Table 2 Ichnofabrics of the Arda River. Ichnotaxa are described in Supplementary Table A2. The degree of bioturbation is quantified by the ichnofabric index (ii) (Bottjer and Droser, Reference Bottjer and Droser1991). Firm substrates indicate stiffgrounds (Wetzel and Uchman, Reference Wetzel and Uchman1998) and firmgrounds (Fürsich, Reference Fürsich1978; Bromley, Reference Bromley1996). Diversity refers to the typical number of distinct burrows per sampling unit. All ichnofabrics indicate oxic settings.

Ichnofabric group 1 - low-moderate bioturbation, homogeneous distribution of traces, low diversity
The ichnofabric classes of group 1 typically present low to moderate bioturbation intensity, homogeneous distribution of traces at the scale of the sampling unit and low diversity of traces:
(1) Unbioturbated ichnofabric. Unbioturbated conglomerates (Facies GmE, Gp);
(2) Low bioturbation ichnofabric. Unbioturbated or sparsely bioturbated (ii 1-2 Palaeophycus. Cryptobioturbation locally present;
(3) Skolithos ichnofabric. Skolithos occurring in massive sands (Facies Sm);
(4) Ophiomorpha ichnofabric (Fig. 9A–C). Ophiomorpha preserved as full-reliefs in planar- or cross-laminated sands (Facies Sh);
(5) Macaronichnus ichnofabric (Fig. 9D–F). Macaronichnus preserved in faintly laminated sands with rare shell debris (Facies Sm).
Interpretation.
The low to moderate bioturbation intensity is interpreted as the result of a stress factor that prevented total bioturbation of the sediment (Bromley, Reference Bromley1996; Taylor et al., Reference Taylor, Goldring and Gowland2003). The homogeneous distribution of traces indicates that the stress factors were persistent, at least at the scale of the observation unit (Gingras et al., Reference Gingras, MacEachern and Dashtgard2011). Specifically, the ichnofabric classes are interpreted as follows:
(1) Unbioturbated ichnofabric. The lack of bioturbation suggests the original lack of endobenthic activity or the non-preservation of biogenic structures (Bromley, Reference Bromley1996). Because this ichnofabric is characterized by bed load related facies, it is likely that physical stress, represented by high hydrodynamics and shifting substrates, prevented endobenthic colonization of these units.
(2) Low bioturbation ichnofabric. This ichnofabric reflects the work of trophic generalists (the producers of Planolites and Palaeophycus; see Gingras et al., Reference Gingras, MacEachern and Dashtgard2011). Brackish setting is suggested by low ichnodiversity, simple structures produced by trophic generalists, monospecific associations, and small size (Pemberton et al., Reference Pemberton, Spila, Pulham, Saunders, MacEachern, Robbins and Sinclair2001; Buatois et al., Reference Buatois, Gingras, MacEachern, Mágano, Zonneveld, Pemberton, Netto and Martin2005; Hauck et al., Reference Hauck, Dashtgard, Pemberton and Gingras2009; Buatois and Mángano, Reference Buatois and Mángano2011). These features are consistent with a foreshore to middle shoreface environment.
(3) Skolithos ichnofabric. Based on the distribution of both animal and plant Skolithos (Bromley, Reference Bromley1996; Gregory et al., Reference Gregory, Campbell, Zuraida and Martin2006; Knaust, Reference Knaust2017), this ichnofabric is interpreted to reflect marine (foreshore to upper shoreface) or, at least, marine-influenced (backshore) settings. A more precise environmental interpretation of this ichnofabric is difficult, also because plant ichnology is an understudied field (Baucon et al., Reference Baucon, Bordy, Brustur, Buatois, De, Duffin and Felletti2012).
(4) Ophiomorpha ichnofabric. This ichnofabric represents the work of a deep-tier community of trophic generalists (the producers of Ophiomorpha). The constructional lining of Ophiomorpha is a strategy to cope with high-energy and shifting substrates (Frey et al., Reference Frey, Howard and Pryor1978; Coelho and Rodrigues, Reference Coelho and Rodrigues2001; Pemberton et al., Reference Pemberton, Spila, Pulham, Saunders, MacEachern, Robbins and Sinclair2001; Taylor et al., Reference Taylor, Goldring and Gowland2003; Buatois and Mángano, Reference Buatois and Mángano2011; Gingras et al., Reference Gingras, MacEachern and Dashtgard2011). These features are consistent with a high-energy foreshore to shoreface environments (see Pemberton et al., Reference Pemberton, Spila, Pulham, Saunders, MacEachern, Robbins and Sinclair2001; Baucon et al., Reference Baucon, Ronchi, Felletti and Neto de Carvalho2014; Leaman et al., Reference Leaman, Mcilroy, Herringshaw, Boyd and Callow2015). Based on the paleoclimatic significance of Ophiomorpha (Goldring et al., Reference Goldring, Cadée, D’Alessandro, de Gibert, Jenkins and Pollard2004, Reference Goldring, Cadée and Pollard2007), this ichnofabric is regarded as an indicator of warm water.
(5) Macaronichnus ichnofabric. This ichnofabric represents the work of a deep-tier community of selective deposit feeders, well-adapted to soft substrates with very high hydrodynamics at the water-sediment interface. These environmental parameters are compatible to foreshores and shorefaces, as also suggested by the environmental preferences of Macaronichnus (high-energy foreshores and shallow shorefaces: Clifton and Thompson, Reference Clifton and Thompson1978; Pemberton et al., Reference Pemberton, Spila, Pulham, Saunders, MacEachern, Robbins and Sinclair2001; Savrda and Uddin, Reference Savrda and Uddin2005; Seike et al., Reference Seike, Yanagishima, Nara and Sasaki2011; Pearson et al., Reference Pearson, Gabriela Mángano, Buatois, Casadío and Raising2013). Macaronichnus has been proposed as an indicator of temperate to cold waters (Quiroz et al., Reference Quiroz, Buatois, Mangano, Jaramillo and Santiago2010).
Ichnofabric group 2 - low-moderate bioturbation, heterogeneous distribution of traces, moderate diversity
The ichnofabric classes of group 2 are characterized by regular heterogeneous distribution of traces. Traces are preserved in heterolithic facies (Fig. 10A) consisting of alternating sand and mud layers (Facies HeB). Mud layers commonly present biogenic structures with poorly defined wall (e.g., Planolites and mantle and swirl structures; homogeneous suite; Fig. 10B) and “sharp walled burrows” (sharp burrows suite; Fig. 10C–E). Sand layers are typically bioturbated by lined burrows (e.g., morphotype A of Schaubcylindrichnus?; Fig. 9F) and/or smooth-walled traces (e.g., Scolicia; Fig. 10G–H). This ichnofabric group comprises three ichnofabric classes, distinguished on the basis of the paucity of “sharp walled burrows” (few sharp burrows, smooth burrows ichnofabric), the dominance of scolicids (sharp burrows, scolicids ichnofabric), and the abundance of “sharp walled burrows” (sharp burrows, smooth burrows ichnofabric).
Interpretation
The regular heterogeneous distribution of trace fossils indicates regular variability in the physicochemical conditions and iterative colonization events (Gingras et al., Reference Gingras, MacEachern and Dashtgard2011). Based on the hypothesis that burrow boundaries store information about the sediment consistency (Uchman and Wetzel, Reference Uchman and Wetzel2011), these events are interpreted as follows:
(1) Colonization of soupground to softground mud. Traces of the homogeneous suite represent the work of organisms “swimming” in soupgrounds (mantle and swirl traces) or deposit-feeding in firmer substrates (Planolites; Lobza and Schieber, Reference Lobza and Schieber1999). Settling of hypopycnal plumes or lofting of hyperpycnal flows are interpreted to be the major depositional processes because of sedimentological evidence and the ichnological similarity with other hyperpycnites (Bhattacharya and MacEachern, Reference Bhattacharya and MacEachern2009);
(2) Dewatering and colonization of firmground mud. The unlined, passively filled burrows of the sharp burrows suite suggest that the sediment became firm enough to avoid collapse of unlined burrows (see Fürsich, Reference Fürsich1978; Bromley, Reference Bromley1996; Uchman and Wetzel, Reference Uchman and Wetzel2011);
(3) Erosion and event deposition. The passive fill of the sharp burrows suite suggests that, after colonization of firmground muds, an abrupt depositional event brought sand to the seafloor. Hyperpycnal flow is interpreted to be the major depositional process (see Bhattacharya and MacEachern, Reference Bhattacharya and MacEachern2009).
(4) Colonization of looseground sand. The smooth burrows suite represents the community that colonized sand brought to the seafloor by event deposits (“post-depositional suite”; Książkiewicz, Reference Książkiewicz1954; Seilacher, Reference Seilacher1962; Uchman and Wetzel, Reference Uchman and Wetzel2011). Lining indicates that he substrate was soft and unconsolidated (Bromley, Reference Bromley1996).
For these reasons, the ichnofabric classes of this group are interpreted to reflect bioturbation of muddy seafloors during low-energy conditions and colonization of sandy event (hyperpycnal) deposits. Based on bioturbation intensity, ichnodiversity, and tiering complexity, the ichnofabric classes of this group are interpreted to reflect a stress gradient, including persistently stressed marine environments (few sharp burrows, smooth burrows ichnofabric), temporarily stressed environments (sharp burrows, scolicids ichnofabric) and stable environments (sharp burrows, smooth burrows ichnofabric). Based on the paleoclimatic significance of Scolicia (Goldring et al., Reference Goldring, Cadée, D’Alessandro, de Gibert, Jenkins and Pollard2004, Reference Goldring, Cadée and Pollard2007), the sharp burrows-scolicids ichnofabric is interpreted to represent temperate to warm waters. It should be also noted that Scolicia is associated with normal marine salinity (Buatois and Mángano, Reference Buatois and Mángano2011), well-oxygenated porewaters (Löwemark et al., Reference Löwemark, Lin, Chen, Yang, Beier, Werner, Lee, Song and Kao2006; de Gibert and Goldring, Reference de Gibert and Goldring2008; Uchman and Wetzel, Reference Uchman and Wetzel2011), restricted competition by organisms of deeper-burrowing tiers (Fu and Werner, Reference Fu and Werner2000), at times being correlated with bottom currents and high sedimentation rates (Fu and Werner, Reference Fu and Werner2000; Löwemark et al., Reference Löwemark, Lin, Chen, Yang, Beier, Werner, Lee, Song and Kao2006; Wetzel et al., Reference Wetzel, Tjallingii and Wiesner2011).
Ichnofabric group 3 - moderate-high bioturbation, homogeneous distribution of traces, low diversity
Ichnofabric group 3 includes a very heterogeneous set of ichnofabric classes with sharp-walled traces and/or passively filled burrows:
(1) Lockeia ichnofabric (Fig. 11A and B). Cemented carbonatic beds (Facies CCB) with no distinct burrows, or predominantly monogeneric (e.g., Lockeia, Ophiomorpha, and Diplocraterion) assemblages.
(2) Thalassinoides ichnofabric (Fig. 11 C, D). Horizontal, Thalassinoides bioturbating, plurimetrical layers of bioclastic sands (Facies Sp). “Y-shaped burrows” and bioerosion structures on shells (e.g., Entobia) are also present.
(3) Coarse-fill burrows ichnofabric (Fig. 11 E). Irregular mottles and circular sharp-walled burrows (Thalassinoides?) filled with coarse-grained sand or fine-grained conglomerate.
Interpretation.
Low diversity and homogeneous distribution of traces suggest persistently stressed conditions or preservation of elite trace fossils by upward migration of deep tiers (Bromley, Reference Bromley1996):
(1) Lockeia ichnofabric. Cementation is a post-colonization feature for the Ophiomorpha-dominated assemblages because a loose substrate is required by its tracemakers to manipulate the characteristic pellets of the burrow lining. Other assemblages show some of the characteristics of the substrate-controlled Glossifungites ichnofacies (i.e., presence of sharp-walled, unlined dwelling burrows of suspension feeders; dominance of robust, vertical to subvertical, simple, and spreite U-shaped burrows; low ichnodiversity; and high abundance; Buatois and Mángano, Reference Buatois and Mángano2011). They could represent substrates that became stiff before the colonization by shallow-tier, suspension-feeding organisms. Presence of food in suspension and lack of deep-tier deposit feeders suggests an energetic environment, possibly a high-energy foreshore.
(2) Thalassinoides ichnofabric. This ichnofabric occurs in plurimetrical units bioturbated by Thalassinoides, implying that it results from the upward migration of a deep tier. This feature and the passive bioclastic fill suggest that this ichnofabric is the result of repeated cycles of colonization, passive infilling, and deposition. The tubular tempestite model, consisting of the repetitive excavation and storm infilling of burrow networks, could explain this pattern (Tedesco and Wanless, Reference Tedesco and Wanless1991).
(3) Coarse-fill burrows ichnofabric. Sharp walls and passive fill indicate that biogenic structures have been emplaced in firmground muds and maintained as open burrows (MacEachern et al., Reference MacEachern, Pemberton, Gingras and Bann2007, Reference MacEachern, Bann, Gingras, Zonneveld, Dashtgard and Pemberton2012; Buatois and Mángano, Reference Buatois and Mángano2011). Because the burrow fill differs from surrounding and overlying sediments, this ichnofabric class represents a trace-fossil omission suite that preserves a high-energy event that would otherwise have passed unnoticed (see MacEachern et al., Reference MacEachern, Bann, Gingras, Zonneveld, Dashtgard and Pemberton2012). These features are consistent with an environment dominated by sediment bypass, such as the margins of a submarine canyon, that is a typical depositional setting of the Glossifungites ichnofacies (Buatois and Mángano, Reference Buatois and Mángano2011).
Ichnofabric group 4 - high bioturbation, homogeneous distribution of traces, moderate to high diversity
The ichnofabric classes of group 4 are typically characterized by intense bioturbation:
(1) Scolicids ichnofabric (Fig. 12A–C). Scolicids (Scolicia, Bichordites) bioturbating sands (Facies Sm), silts and sandy muds (Facies Fm);
(2) Palaeophycus ichnofabric (Fig. 12D–F). Numerous distinct burrows (e.g., Planolites, Palaeophycus, and Schaubcylindrichnus – morphotype A) bioturbating sands, silts, and sandy muds (Facies Sm, Fm);
(3) High bioturbation ichnofabric (Fig. 12G–L). Homogeneous or mottled muds and silts (Facies Fm). Distinct burrows are not always present.

Figure 12 (color online) (A) Scolicids ichnofabric overlying the sand/mud couplets typical of ichnofabric group 2. (B) Close-up of A, showing a specimen of Scolicia reworking a mud layer. Palaeophycus and Planolites are present in the sand layer, whereas “sharp-walled traces” bioturbates the mud layer. (C) Scolicid. (D) Palaeophycus ichnofabric with a large specimen of Teichichnus (morphotype A) and Palaeophycus. (E) Palaeophycus. (F) Rosselia? (G) High bioturbation ichnofabric. (H) Close-up of G, showing a probable specimen of Asterosoma. (I) High bioturbation ichnofabric with a distinct Teichichnus (morphotype B). (L) High bioturbation ichnofabric with distinct lined vertical burrows (Schaubcylindrichnus?)
Interpretation
High bioturbation intensity and homogeneous bioturbation are interpreted to reflect slow sedimentation, stable, well oxygenated physicochemical conditions (Taylor et al., Reference Taylor, Goldring and Gowland2003; Gingras et al., Reference Gingras, MacEachern and Dashtgard2011; Uchman and Wetzel, Reference Uchman and Wetzel2011). The ichnofabric classes of this group have been interpreted as follows:
(1) Scolicids ichnofabric. The abundance in scolicids suggests oxic porewater with normal marine salinities (de Gibert and Goldring, Reference de Gibert and Goldring2008; Buatois and Mángano, Reference Buatois and Mángano2011); the possible influence of bottom currents (Löwemark et al., Reference Löwemark, Lin, Chen, Yang, Beier, Werner, Lee, Song and Kao2006; Wetzel et al., Reference Wetzel, Tjallingii and Wiesner2011); restricted competition by organisms of deeper burrowing tiers (Fu and Werner, Reference Fu and Werner2000); and a good quantity and quality of food (Wetzel, Reference Wetzel2010). These environmental features are consistent with an upper shoreface to offshore depositional setting.
(2) Palaeophycus ichnofabric. This ichnofabric class presents similar features with respect to the previously discussed ichnofabric class, but the higher bioturbation intensity and diversity suggest a less-stressed environment. This scenario suggests an offshore depositional environment influenced by hyperpycnal flows.
(3) High bioturbation ichnofabric. With respect to the other ichnofabrics of the same group, this ichnofabric presents the highest bioturbation intensity, probably indicating higher bioturbation rates, lower sedimentation rates, and a higher availability of food (see Wetzel and Uchman, Reference Wetzel and Uchman1998). These features are interpreted to represent an oxic offshore environment.
DISCUSSION
Paleoenvironment of the Arda section
In this section, the seven fining-upward cycles (Fig. 2) have been interpreted in terms of paleoenvironments, integrating the results of sedimentology, body fossil paleontology, and ichnology (Fig. 13).

Figure 13 (color online) Environmental evolution of the Arda Section. A simplified stratigraphic log (sed. log) with the age of the section, based on calcareous nannofossil (CNZ) and foraminifera (FZ) biostratigraphy, is illustrated (Crippa et al., Reference Crippa, Angiolini, Bottini, Erba, Felletti, Frigerio and Hennissen2016). For each tool used (Sed, sedimentology; Body, body fossils; Trace, trace fossils) it was analyzed: Water depth: 1, foreshore (region between high tide water level and the low tide water level); 2, shoreface (low tide water level-fair weather wave base); 3, offshore transition and deeper (wave base-shelf edge break; Nichols, Reference Nichols2009). In the modern Adriatic Sea, these regions are respectively at depths of <0 m, 0–10 m, >10 m. Energy: 1, low (nutrients in deposition); 2, high and fluctuating (hyperpycnal flows); 3, high (nutrients in suspension). Sedimentation rate: 1, low; 2, high. Oxygenation: 1, disoxic (2–0.2 ml O2/l H2O); 2, oxic (8.0–2.0 ml O2/l H2O; Tyson and Pearson, Reference Tyson and Pearson1991; Buatois and Mangano, 2011, p.104). Salinity: 1, brackish (0.5–30‰); 2, seawater (30–40‰; Buatois and Mangano, 2011, p. 107). Water temperature: 1, cold; 2, temperate; 3, warm. For body fossils, water temperature was based on oxygen isotope composition of bivalve shells, considering cold >2.5‰; temperate, 0–2.5‰; warm, <0 ‰ (data from Crippa et al., Reference Crippa, Angiolini, Bottini, Erba, Felletti, Frigerio and Hennissen2016). For trace fossils, climate is defined as following: cold (climate at modern artic to temperate latitudes), temperate (climate at modern temperate to tropical and subtropical latitudes), and warm (climate at modern tropical and subtropical latitudes). Arctic latitudes are between 66° and 90°, temperate latitudes are between 35° and 66°, tropical and subtropical latitudes are between 0° and 35° (Goldring et al., Reference Goldring, Cadée, D’Alessandro, de Gibert, Jenkins and Pollard2004, Reference Goldring, Cadée and Pollard2007).
Cycle 0
Cycle 0 does not constitute a full cycle, as it lacks the complete facies sequence characterizing Cycles I–VII (see Facies Association, above). The presence of brachiopods, corals, and echinoids (Biofacies 3; 45.65–46.05 m) as well as the prevalence of high-bioturbation ichnofabric in this cycle would suggest an offshore transition environment characterized by low hydrodynamic energy and low sedimentation rate. Oscillations to high-energy foreshore to shoreface settings with high sedimentation rate do also occur, however, as testified by the presence of rheophilous mollusks in Biofacies 1 (37.05–43.25 m). In addition, the presence (45.20–46.60 m) of a characteristic body of cemented biocalcarenite with abundant burrows (Thalassinoides ichnofabric) is noteworthy. Different physical conditions can be assumed for its formation, such as reduced fine-grained terrigenous input or strong bottom reworking by currents. The upward reduction of winnowing events restores the initial low-energy conditions characterized by deposition of finer-grained sediments. Here, body fossils show, besides a diagenetic dissolution of aragonitic shells, a high degree of degradation (Biofacies 2; 44.90 m, 47.35–49 m), suggesting a high-energy setting winnowed by currents. In this cycle, oxygen isotope values of bivalve shells (Crippa et al., Reference Crippa, Angiolini, Bottini, Erba, Felletti, Frigerio and Hennissen2016) point to temperate-cold conditions, related to a mid-shelf environment (depth=~50 m).
Cycle I
Cycle I is interpreted to reflect environmental conditions similar to those of the previous cycle, i.e., prevailing offshore transition conditions. Specifically, with the exception of a thin sandstone bed in the basal part, most of Cycle I is represented by monotonous, fine-grained to laminated siltstones (Facies L) deposited by suspension settling from decelerating hyperpycnal flows. Body and trace fossils record a change in the environment; the lower part of the cycle accounts for lower shoreface to offshore transition settings (Biofacies 2 [56.35–58.35 m] and high-bioturbation ichnofabric), whereas the middle part records a temporary shift to shallower and higher-energy foreshore to shoreface settings (Biofacies 4 [59–70.02 m] and presence of the Macaronichnus and scolicids ichnofabrics). The topmost part testifies to a return to a deeper water setting (Biofacies 8 [80.30–91.40 m] and high-bioturbation ichnofabric). Although only a few paleotemperature data are available from body fossils of this cycle, these suggest a temperate-cold water environment. Such interpretation is consistent with the presence of Macaronichnus, a typical temperate- to cold-water indicator (Quiroz et al., Reference Quiroz, Buatois, Mangano, Jaramillo and Santiago2010), and Scolicia, which, though not exclusive, is common in temperate waters (Goldring et al., Reference Goldring, Cadée, D’Alessandro, de Gibert, Jenkins and Pollard2004, Reference Goldring, Cadée and Pollard2007).
Cycle II
Cycle II mainly documents offshore transition environments recording a cooling event. The basal part of the cycle documents a shoreface environment characterized by high to high-fluctuating energy and high sedimentation rate. This cycle includes coarse-grained deposits related to shear/drag forces exerted by the overpassing long-lived turbulent (hyperpycnal) flow over coarse-grained materials lying on the flow bottom. High-density flows triggered by river floods can mix and deposit skeletal remains from different shallow-water communities. The poor preservation of body fossils in Biofacies 10 (92.50–98.30 m), the abrupt changes in ichnofabric, the presence of mud clasts and of reworked vegetal debris, besides field observations, all indicate the presence in this interval (91.40–98 m) of a channel cutting obliquely (south-southwest to north-northeast) across the main succession and discharging fresh water and sediments. Aside from this interval, sedimentology and trace fossils document offshore transition environments, whereas body fossils record a shallowing upward trend, passing from an offshore transition environment characterized by low-energy and low sedimentation rate (Biofacies 8; 101–104.10 m) to a shoreface setting with higher energy (Biofacies 4; 106.50–111.60 m). From a paleoclimatic point of view, the first occurrence of the “northern guest” Arctica islandica (103.70 m) and the abundant presence of Macaronichnus (94 m) mark the beginning of a climatic cooling in the area, which is further supported by bivalve shell oxygen isotope composition (Crippa et al., Reference Crippa, Angiolini, Bottini, Erba, Felletti, Frigerio and Hennissen2016).
Cycles III–VI
Cycles III to VI show a more regular organization: each cycle records a deepening upward trend (from foreshore-shoreface to offshore transition settings) and a parallel decrease in hydrodynamic energy and sedimentation rate. Each cycle frequently has an erosive base and starts with conglomerates or rip-up mud clasts (Facies B), followed above by either massive or stratified sandstones (Facies S). Here, low bioturbation ichnofabric indicates brackish conditions, possibly caused by direct fluvial discharge of fresh water and sediments by hyperpycnal flows. This is also supported by the presence of Biofacies 10 (170–194.10 m; 208.40–210.40 m) in Cycles V and VI; this biofacies contains an ecologically mixed fauna and specimens showing poor preservation, both indicating a high-energy environment affected by high-density flows triggered by river floods that mix skeletal remains from different environments. In Cycles III–VI, transported body fossil assemblages and evidence of density flows become more frequent; this means more river discharge and thus more terrigenous input into the Paleo-Adriatic basin. The increase in the tectonic uplift and erosion of the Apennines after 1.80 Ma (e.g., Amorosi et al., Reference Amorosi, Farina, Severi, Preti, Caporale and Di Dio1996; Bartolini et al., Reference Bartolini, Caputo and Pieri1996; Argnani et al., Reference Argnani, Bernini, Di Dio, Papani and Rogledi1997, Reference Argnani, Barbacini, Bernini, Camurri, Ghielmi, Papani, Rizzini, Rogledi and Torelli2003; Dominici, Reference Dominici2001, Reference Dominici2004), the proximity to the coast, and possibly climatic deterioration (e.g., increased precipitation and/or increased ice melting during summer and more ice accumulation during winter) may account for the observed increase of hyperpycnal flows in these cycles. The top of each cycle records fully marine conditions with typical faunal associations/ichnofabrics of low-energy settings (Biofacies 7; 146.55–167.90 m, 196–207 m; high bioturbation ichnofabric), reflecting the normal settling when the flow completely stops or sedimentation in a distal portion of the delta system.
Cycle V is particularly rich in scolicid-dominated ichnofabrics. The sedimentological evidence for hyperpycnal flows may indicate that Scolicia-dominated ichnofabrics are a proxy for extrabasinal turbidites, sensu Zavala and Arcuri (Reference Zavala and Arcuri2016), such as those deposited by hyperpycnal flows. This hypothesis is plausible, as Scolicia is correlated with the abundance of food (Wetzel, Reference Wetzel2010) and extrabasinal turbidites are rich in phytodetritus because they originated from the continent (Zavala and Arcuri, Reference Zavala and Arcuri2016). By contrast, intrabasinal turbidites originated within the marine basin and are therefore less rich in phytodetritus (Zavala and Arcuri, Reference Zavala and Arcuri2016). Further case studies are required to test this hypothesis.
Paleoclimatic indicators mainly suggest temperate water, although oxygen isotopes from bivalve shells and evidence from trace fossils (Macaronichnus) indicate lower water temperatures in a few levels, confirming the change towards cooler climates, affecting the Paleo-Adriatic after the arrival of the “northern guests.” In Cycle III, trace fossils record high-frequency climate fluctuations; abundant Ophiomorpha, an ichnological indicator of warm climate, are present at little stratigraphic distance from Macaronichnus-dominated horizons.
Cycle VII
Cycle VII represents the last marine cycle of the Arda succession before the establishment of a continental environment with freshwater mollusks and vertebrate faunas (Bona and Sala, Reference Bona and Sala2016; Monesi et al., Reference Monesi, Muttoni, Scardia, Felletti, Bona, Sala, Tremolada, Francou and Raineri2016); it documents a foreshore to upper shoreface environment characterized by high hydrodynamic energy and high sedimentation rate, due to discharge by fluvial floods. This cycle is characterized by the dominance of ichnofabrics related to brackish water (low bioturbation ichnofabric, few sharp burrows-smooth burrows ichnofabrics). Increased freshwater input, and thus dilution of salinity, is also indicated by the presence of Biofacies 5 (230.80–237 m), recording the only occurrence of Glycymeris insubrica, a species that tolerates salinity variations (e.g., Malatesta, Reference Malatesta1974; Lozano Francisco et al., Reference Lozano Francisco, Vera Pelaez and Guerra Merchan1993; Raineri, Reference Raineri2007; Crnčević et al., Reference Crnčević, Balić and Pećarević2013). At the top of the succession, Crippa et al. (Reference Crippa, Angiolini, Bottini, Erba, Felletti, Frigerio and Hennissen2016) observed the presence of abundant brackish-water benthic foraminifera and low oxygen isotope ratios in bivalve shells, indicating salinity reduction due to freshwater river discharge. All this evidence, together with the presence of frequent bed-load deposits (Facies B), indicates a foreshore to upper shoreface environment for this part of the section.
Regional significance of the Arda section
The Arda sedimentary succession represents a valuable case study, as it offers the rare opportunity to study depositional dynamics through phases of strong natural climate change within a tectonically active setting. The stratigraphic and paleontological investigation presented for the Arda section highlights the importance of integrated studies to disentangle the effects of climate and tectonic processes acting to structure sedimentary successions during the early Pleistocene. In this respect, the overall regressive trend of the marine part of Arda section, consisting of offshore/prodelta (Cycles 0–II) passing to prodelta/delta front (Cycles III–IV) and to intertidal/coastal (Cycle VII) stacked successions, can be directly related to deformation phases of the local fronts of Apennine mountains; this is represented by the change in the dip of the bedding from 20° in the stratigraphically lower part of the section up to <5° in the upper part. While tectonic and climatic forcings are acting simultaneously, regional studies (e.g., Gunderson et al., Reference Gunderson, Pazzaglia, Picotti, Anastasio, Kodama, Rittenour and Frankel2014) indicate that the early Pleistocene (~1.60–1.10 Ma) structuring of this sector of the northern Apennines has been characterized by unsteady but rapid and intense deformation. This specified interval of time encompasses the entire marine sedimentation in the Arda section (see Geological Setting; Crippa et al., Reference Crippa, Angiolini, Bottini, Erba, Felletti, Frigerio and Hennissen2016). In addition, overall regressive trends were already documented for few other successions outcropping along the northeastern margin of the Apennines, such as the Enza and Stirone River sections (e.g., Pelosio and Raffi, Reference Pelosio and Raffi1977; Dominici, Reference Dominici2001; Gunderson et al., Reference Gunderson, Pazzaglia, Picotti, Anastasio, Kodama, Rittenour and Frankel2014).
The cyclothemic nature of the sedimentary succession retains an expression of climatic changes that occurred during the early Pleistocene and was modulated by the morphology of the basin (see Dominici, Reference Dominici2001). Specifically, cyclic stacking patterns of fan-delta forestepping/backstepping episodes described here were produced primarily by the onset and disappearance of local climatic conditions favoring the development of catastrophic flooding through time (see other geologic examples in Weltje and de Boer, Reference Weltje and de Boer1993; Mutti et al., Reference Mutti, Davoli, Tinterri and Zavala1996). Our results correlate well with regional changes at higher temporal frequencies, recording, besides the Apennine uplift, the same evolutionary history of coeval successions in the Castell’Arquato basin and the Paleo-Adriatic region (e.g., Stirone and Enza River sections; Papani and Pelosio, Reference Papani and Pelosio1962; Dominici, Reference Dominici2001, Reference Dominici2004; Gunderson et al., Reference Gunderson, Pazzaglia, Picotti, Anastasio, Kodama, Rittenour and Frankel2014). The analyses of the biota and of their traces record not only local but also global climate changes. The occurrence of the “northern guest” bivalve A. islandica, of Macaronichnus trace fossil, and of the “northern guests” foraminifera Hyalinea balthica and Neogloboquadrina pachyderma left coiling (Crippa et al., Reference Crippa, Angiolini, Bottini, Erba, Felletti, Frigerio and Hennissen2016) in the section, testify to climatic deterioration, i.e., cooling, which represents an expression of the climatic changes occurring during the early Pleistocene, leading to the onset and establishment of Middle and Upper Pleistocene continental glaciations.
The multidisciplinary method: advantages and disadvantages
The methodological significance of this study is to provide a multidisciplinary approach to paleoenvironmental reconstructions. Ecosystem evolution is affected by many different factors; instead of just looking at one factor, we followed an integrated approach for the understanding of past environments, integrating three different tools (sedimentology and body and trace fossil paleontology). The practical application of a multidisciplinary approach in paleoenvironmental reconstructions revealed some methodological advantages and disadvantages. One of the main advantages in applying this method is the possibility to investigate the ecosystems in detail from different points of view, obtaining a more complete and reliable picture of past environments. The use of a single tool, besides giving a one-sided perspective, may be affected by biases specific to the tool itself, which can be avoided or compensated for when using two or more tools. In employing different tools, however, data may also disagree. Disagreements are generally due to different sampling strategies or to problems intrinsic to the tools themselves, e.g., the lack of continuity in the recorded data along the succession and thus a lower resolution scale of analysis, which does not allow the identification of small variations. In the case of the Arda River section, data from sedimentology and body fossils have shown a lower resolution in the scale of analysis compared to trace fossils. For practical reasons, sedimentology, body fossil paleontology, and ichnology involve different sampling schemes, characterized by different resolutions, which also affect the results of the analysis. In some cases, this has led to possible misinterpretations and disagreements between the different tools used; on the other hand, the higher resolution shown by trace fossils has allowed identification of changes at a finer scale of analysis, highlighting also the smallest environmental variation. Generally, we noted that these three different approaches complement one another quite well and compensate for their respective defects, giving strength and robustness to the obtained results, besides a more complete and exhaustive picture of past ecosystems.
The geological record provides a number of different settings and locations within different time intervals, where this multidisciplinary approach can be applied. Elements required are well-exposed, continuous, and age-constrained sedimentary successions rich in fossils (micro-, macro-, and/or trace fossils) and two (although three or more are preferred) different tools on which to base the paleoenvironmental reconstruction. The use of a multidisciplinary approach would greatly improve the resolution of past environment reconstructions and should be applied more frequently for these purposes, taking advantages of the numerous opportunities that the geological record provides.
CONCLUSION
The detailed multidisciplinary study presented here (sedimentology and body and trace fossils) has proved to be a powerful tool for resolving paleoenvironmental dynamics recorded in sedimentary succession from collisional margins during time intervals of climate change. The paleoenvironmental evolution of the marine Arda River section has been interpreted taking into account the interplay between tectonics and climatic factors. Based upon sedimentology, body fossils, and trace fossils, it corresponds to a subaqueous extension of a fluvial system, originated in a tectonically active setting during phases of advance of fan deltas affected by high-density flows triggered by river floods, which are an expression of early Pleistocene climate changes. It documents a fully marine and well-oxygenated environment, bounded at the top by continental conglomerates indicating a major sea-level drop and the establishment of a continental environment; indeed, a general regressive trend is observed through the section, passing from a prodelta (Cycles 0–II) to a delta front (Cycles III–IV) and an intertidal zone (Cycle VII) setting. Lower order transgressive and regressive cycles with shifts from lower foreshore-shoreface to offshore transition environments have been identified, with supposed water depths ranging between 5 and 50 m. The hydrodynamic energy and the sedimentation rate are not constant through the section, but they are influenced by hyperpycnal flows which caused an increase in terrigenous input linked to fluvial floods, whose sediments are mainly supplied by an increase in the Apennine uplift and erosion, especially after 1.80 Ma (e.g., Amorosi et al., Reference Amorosi, Farina, Severi, Preti, Caporale and Di Dio1996; Bartolini et al., Reference Bartolini, Caputo and Pieri1996; Argnani et al., Reference Argnani, Bernini, Di Dio, Papani and Rogledi1997, Reference Argnani, Barbacini, Bernini, Camurri, Ghielmi, Papani, Rizzini, Rogledi and Torelli2003; Dominici, Reference Dominici2001, Reference Dominici2004). The regressive trend and the climatic deterioration (i.e., cooling) recorded through the section are an expression of the climatic changes occurring during the early Pleistocene; our results correspond well with other studies of both global and local climatic/tectonic changes, recording the same evolutionary history of coeval successions in the Castell’Arquato basin and the Paleo-Adriatic region.
The lower Pleistocene Arda River marine succession represents only one of the numerous case studies provided by the geological record where a multidisciplinary approach can be applied to interpret past complex ecosystems in the frame of climate change and tectonic activity. A multi-approach analysis based on the integration of sedimentological data and body and trace fossils would greatly improve the resolution of paleoenvironmental reconstructions and should also be extended to other geological sites.
ACKNOWLEDGMENTS
G. Crippa was supported by 2011 Italian Ministry PRIN Project to E. Erba. A. Baucon was supported by the ROSAE project (www.rosaeproject.org). We warmly thank the editors, S. Schneider, and an anonymous reviewer for their constructive comments that improved the paper. L. Angiolini and M. Marini are thanked for constructive discussions. M. Marini is also warmly thanked for checking the English manuscript.
Supplementary material
To view supplementary material for this article, please visit https://doi.org/10.1017/qua.2018.10