I. INTRODUCTION AND BACKGROUND
The ubiquity of metal organic frameworks (MOFs) and coordination polymers (Tranchemontagne et al., Reference Tranchemontagne, Mendoza-Cortes, O'Keeffe and Yaghi2009) in the literature reflects their versatility and utility in applications such as selective gas sorption (Rouquerol et al., Reference Rouquerol, Avnir, Fairbridge, Everett, Haynes, Pernicone, Ramsay, Sing and Unger1994). Notwithstanding our sophisticated understanding of the synthesis and chemistry of MOFs (Furukawa et al., Reference Furukawa, Kim, Ockwig, O'Keeffe and Yaghi2008; Long and Yaghi, Reference Long and Yaghi2009), tailoring a synthesis to produce a specific MOF optimized for a particular gas separation is not yet possible. The mainstay of solid-state materials discovery remains intuition-guided and this is necessarily a slow, iterative process. Two strategies can be employed to make the process more efficient: computational screening and in situ experimental screening.
Computational screening uses searchable databases, such as those of the Materials Project (Jain et al., Reference Jain, Ong, Hautier, Chen, Richards, Dacek, Cholia, Gunter, Skinner, Ceder and Persson2013) for the ab initio prediction of the properties (Ceder and Persson, Reference Ceder and Persson2015). For example, high-throughput computational screening of databases of hypothetical and experimentally realized MOFs and other microporous compounds, recently identified SBMOF-1 (Stony Brook metal organic framework-1) as the most selective for xenon separation from radioactive gas streams (Simon et al., Reference Simon, Mercado, Schnell, Smit and Haranczyk2015; Banerjee et al., Reference Banerjee, Simon, Plonka, Motkuri, Liu, Chen, Smit, Parise, Haranczyk and Thallapally2016a). This prediction was confirmed experimentally: SBMOF-1 exhibits high xenon adsorption capacity and Xe/Kr selectivity under conditions of concentration and humidity pertinent to nuclear fuel reprocessing (Banerjee et al., Reference Banerjee, Simon, Plonka, Motkuri, Liu, Chen, Smit, Parise, Haranczyk and Thallapally2016a). In principle, any separation can be treated similarly, with the caveat that a still undiscovered microporous material, not in the database, may have better performance.
In situ experimental screening is used to quickly identify desirable properties of de novo materials, produced in a program of exploratory synthesis and not available in structural databases. Below we describe an experimental set-up to rank order the suitability of novel MOFs for gas separations. The goal within one sample loading is to establish novelty, stability, microporosity, and relative selectivity under variable humidity conditions (Plonka et al., Reference Plonka, Banerjee, Woerner, Zhang, Nijem, Chabal, Li and Parise2013b).
The experimental screening approach grew out of a desire to rapidly evaluate MOFs produced from linkers that contained structural features deemed desirable in separations of CO2 from humid gas streams (Banerjee et al., Reference Banerjee, Zhang, Plonka, Li and Parise2012; Plonka et al., Reference Plonka, Banerjee, Woerner, Zhang, Nijem, Chabal, Li and Parise2013b). Theoretical work identified interactions between CO2 molecules and the π-clouds of aromatic linkers (Tsuzuki and Fujii, Reference Tsuzuki and Fujii2008; Banerjee et al., Reference Banerjee, Zhang, Plonka, Li and Parise2012) as an alternative to sorption on under-coordinated metal sites in zeolites and MOFs. Water effectively competes with CO2 at open metal sites and impedes the performance of such frameworks in commercial humid flue gas (Liu et al., Reference Liu, Wang, Benin, Jakubczak, Willis and LeVan2010; Kizzie et al., Reference Kizzie, Wong-Foy and Matzger2011; Sumida et al., Reference Sumida, Rogow, Mason, McDonald, Bloch, Herm, Bae and Long2012). In the search for compounds that selectively sorb gases from humid gas streams, several linkers and metal combinations were tried and sdb (sulfonyldibenzoate) in combination with Ca (Banerjee et al., Reference Banerjee, Zhang, Plonka, Li and Parise2012; Plonka et al., Reference Plonka, Banerjee, Woerner, Zhang, Nijem, Chabal, Li and Parise2013b) and Cd (Plonka et al., Reference Plonka, Banerjee, Woerner, Zhang, Li and Parise2013a) produced compounds that selectively sorb CO2 (Figure 1). The structural origin for the selectivity was traced to a key interaction between two phenyl rings of the sdb linker and the molecular quadrupole of CO2. This result prompted the search for other microporous networks containing this structural feature. It was during the search for MOFs selective for CO2 removal that it was realized X-ray diffraction – differential scanning calorimetry (XRD-DSC) is a very effective experimental screening tool (Plonka et al., Reference Plonka, Banerjee, Woerner, Zhang, Nijem, Chabal, Li and Parise2013b). When combined with a gas/humidity handling system (Woerner et al., Reference Woerner, Plonka, Chen, Banerjee, Thallapally and Parise2016), XRD-DSC efficiently rank orders novel materials according to their selectivity toward a particular gas.

Figure 1. (Color online) Portion of the structure of Casdb (SBMOF-1): CO2 adduct, showing the relationship between the CO2 molecule occupying the biphenyl “π-pocket” site referred to in the text. Yellow spheres represent S, red O, grey C, and blue Ca.
The need to rapidly evaluate candidate phases, which might be useful for targeted, selective gas separations discovered in a program of MOF synthesis, motivates the work described in this paper. XRD data of native and activated MOFs, and coordination polymers, are used post-synthesis to rapidly determine the likelihood that a material is stable and potentially microporous. By combining simultaneous XRD-DSC with a gas manifold and vacuum swing techniques, one can rank order the selectivity of candidates toward particular gases according to their enthalpies of adsorption. In this paper, we describe an apparatus capable of providing simultaneous XRD and thermal response (DSC) while varying and measuring temperature, gas pressure (from vacuum to 1 atm), and relative humidity (RH). The versatility of this apparatus and the accuracy of the heats of adsorption obtained from it are demonstrated in the results of four case studies: (1) screening coordination polymers produced from a program of synthetic work designed to discover novel materials with structural features discovered during studies of (2) the so-called “pi-pocket” responsible for the humidity independence of gas sorption, especially CO2 sorption, on SBMOF-1. (3) Simultaneous XRD-DSC facilitates studies of the consequences for thermal response upon structural changes occurring in MOFs during gas sorption–desorption cycles. For example, using data collected during several vacuum swing cycles exposing ZIF-7 (zeolitic imidazolate framework-7) to gas pressures up to 1 atm, and at dry and 75% RH conditions, we show the presence of water vapor reduces the measured enthalpy of adsorption and shifts the onset of the previously reported “gate opening” of the ZIF-7 pore by +0.1 atm. (4) The accuracy of the heats of absorption derived from DSC measurements, determined by comparison with results derived from measurements of isotherms, demonstrates the reliability of the XRD-DSC approach for rapid screening of materials for selective gas sorption.
II. EXPERIMENTAL
A. Synthesis of powders
All powders were synthesized under solvothermal conditions using 23 ml Teflon lined stainless steel Parr autoclaves. A typical synthesis for SBMOF-1 (Casdb) involved dissolving a mixture of calcium chloride (0.07 g) and 4,4′-sulfonyldibenzoic acid (sdb, 0.19 g, Figure 2) in 10 g of ethanol with stirring for 2 h to achieve homogeneity. The resultant solution was heated at 180 °C for 5 days. The product was recovered by filtration, washed with ethanol, and air-dried. Similarly, SBMOF-2 (Catcpb) was synthesized from a mixture of 0.25 mmoles of CaCl2 (0.027 g) and 0.05 mmoles (0.03 g) of 1,2,4,5-Tetrakis(4-carboxyphenyl)benzene (tcpb, C34H22O8, Figure 2) dissolved in 12 g of ethanol and stirred for 2 h to achieve homogeneity. The resultant solution was heated at 373 K for 3 days. Solids were obtained from filtration, washed with ethanol, and air-dried.

Figure 2. Some of the linkers used include ctpb, 1,2,4,5-Tetrakis(4-carboxyphenyl)benzene, sdb, 4,4′-sulfonyldibenzoic acid, and sbp, 4′,4″-sulfonylbis([1,1′-biphenyl]-4-carboxylic acid), which was produced in house from the sdb precursor.
For the synthesis of ZIF-7, a solid mixture of zinc nitrate tetrahydrate [Zn(NO3)2·4H2O] (0.045 g) and benzimidazole (0.070 g) was dissolved in 5 ml of DMF and stirred for 2 h to achieve homogeneity. The resultant solution was heated for 3 days at 100 °C and the product was collected by filtration, washed using DMF, and allowed to air-dry.
Inspired by observations that a key structural feature, the V-shaped “pi-pockets” formed by biphenyl rings (Figure 1), provides an efficient binding site for selective capture of CO2, even at high RH, we embarked upon a series of exploratory syntheses to uncover other frameworks with these properties. Apart from commercially available V-shaped dicarboxylate linkers, such as ctpb and sdb shown in Figure 2, we also designed and synthesized a V-shaped linker, L1 = 4′,4″-sulfonylbis([1,1′-biphenyl]-4-carboxylic acid) = sbp (Figure 2). Precursors, 4,4′-sulfonylbis(iodobenzene) and 4-iodobenzenesulfonic acid, were synthesized according to recipes in the literature (Gu et al., Reference Gu, Deng and Yao2007). The purity of the product was checked with 1H NMR and mass spectroscopy. Synthesis of linker L1: To a 500 ml flask equipped with a stir bar were added 4-carboxyphenylboronic acid (3.82 g, 23 mmol), 4,4′-sulfonylbis(iodobenzene) (3.58 g, 10 mmol), Pd(PPh3)4 (0.80 g, 0.69 mmol), aq. Na2CO3 (2 M, 50 ml), 1,4-dioxane (50 ml, degassed), and anhydrous ethanol (100 ml). The mixture was heated to reflux under Ar for 24 h. After cooling, the mixture was filtered and the filtrate was washed with water and THF. The solution was then acidified with HCl, and a white precipitate formed. The mixture was filtered and the solid was washed with water. 1H NMR (400 MHz, DMSO-d 6) ∂ 8.09 (d, J = 12 Hz, 4H), 7.95–8.11 (m, 8H), 7.80 (d, J = 12 Hz, 4H) 13C NMR (100 MHz, DMSO-d6) ∂ 166.9, 144.1, 142.3, 140.4, 130.8, 130.0, 128.4, 128.2, 127.8. ESI-MS (neg mode): m/z [M-H] 257.1.
Linker L2 was synthesized by the method described above, using 4-iodobenzenesulfonic acid in place of 4,4′-sulfonylbis(iodobenzene). 1H NMR (400 MHz, DMSO-d 6) ∂ 13.00 (s, 1 H), 8.00 (d, J = 12 Hz, 2 H), 7.81 (d, J = 12 Hz, 2 H), 7.70 (s, 4H). ESI-MS (neg mode): m/z 277.0.
Several metal nitrates were combined with L1 and L2 to form novel materials. For example, a zinc framework designated ZnL1 was synthesized from a mixture of Zn(NO3)2·6H2O [0.13 g, 0.4 mmol] and L1 [0.02 g, 0.04 mmol] dissolved in 4 ml of DMSO and 2 ml of EtOH and stirred for 2 h to achieve homogeneity. The resultant solution was heated for 5 days at 125 °C, and the product was collected by filtration as brown prismatic crystals, which were washed using ethanol and allowed to air-dry. Further details of the characterization of these materials are presented below.
B. The XRD-DSC experiment
The XRD-DSC system assembled at Stony Brook (Woerner et al., Reference Woerner, Plonka, Chen, Banerjee, Thallapally and Parise2016) is based on a Rigaku Ultima IV diffractometer (CuKα radiation), a Rigaku XRD-DSC stage and a Rigaku HUM-1 humidity generator. The Rigaku components were optimized for adsorption studies with the addition of a ULVAC vacuum pump (5 × 10−4 Torr), a vacuum manifold, and a custom-built humid atmosphere swing chamber (HASC, Figure 3). Additionally, an upstream gas manifold can be introduced before the humidity generator for selecting the desired gases.

Figure 3. (Color online) (a) Photo of complete XRD-DSC system. (b) XRD-DSC stage with sealing cap. (c) XRD-DSC attachment without cap showing the Al2O3 standard and sample powder on aluminum pans. (d) Schematic of entire XRD-DSC system. (e) Schematic of humid atmosphere swing chamber. The base of the chamber is designed like a syringe with a triple O-ring seal so the volume of the chamber can be changed without atmospheric contamination; after (Woerner et al., Reference Woerner, Plonka, Chen, Banerjee, Thallapally and Parise2016).
The desired gas flows from the selection manifold through a flow meter and into the humidity generator where mass flow controllers balance the mixing of wet and dry gas streams (Figure 3). The humidified gas mixture is then directed into the HASC where the RH of the gas stream can stabilize based on a feedback interaction between the humidity probe and generator and can be exhausted through a valve until the atmosphere has a stable flow at the desired RH (Figure 3). Once the atmosphere has stabilized at the desired humidity, it is directed to the XRD-DSC stage through a needle valve. In addition to atmosphere swings, the chamber allows for vacuum-humid atmosphere swings as the input from the generator can be closed off (Banerjee et al., Reference Banerjee, Wang, Plonka, Emge, Parise and Li2016b). Thus, when the valve is opened between the XRD-DSC stage (at vacuum) and the chamber, the syringe base of the HASC moves because of the pressure differential and delivers the humid atmosphere to the sample in the XRD-DSC stage.
When the atmosphere is changed from vacuum to gas, an exothermic effect is observed on the DSC curve. The area of the peak corresponds to the heat released from gas adsorption, and it allows for direct measurements of the enthalpy (ΔH; kJ molMOF−1), which in turn can be used to calculate isosteric heat of adsorption (Q st; kJ molGAS−1) through the relation ΔH = Q st × n i, where n i corresponds to the moles of the adsorbed gas and is determined with gas isotherms measurements.
Vacuum-swing experiments can be performed with different pressure steps. In a typical experiment, 1 atm of gas is introduced in one step so the differential enthalpy between the empty pore and the gas-loaded MOF can be determined at ambient conditions (1 atm gas, RT). In the case of flexible compounds, it is beneficial to increase the gas pressure in multiple steps, allowing reconstruction of the enthalpy of the gas adsorption before and after a phase transition. The interpretation of the PXRD data collected at different pressure steps also shows the structural changes accompanying the phase transition.
In a second type of XRD-DSC experiment, the atmosphere of the sample is changed between different gases. For example, cycling between CO2/N2 gases allows qualitative characterization of the CO2/N2 selectivity of MOFs in dry and humid conditions. Gas displacements that are exothermic indicate a preference for the displacing gas, and those that are endothermic indicate a preference of the framework for the gas being displaced. The area under the exothermic peak corresponds to a difference between CO2 adsorption and N2 adsorption enthalpy. Through the introduction of humidity in the gas flow also facilitates determination of how effectively CO2 competes with water at the strong adsorption sites.
The set-up shown in Figure 3 can be configured for a wide variety of experiments including: (1) routine XRD observations of materials for determination of novelty; (2) estimation of structural changes, including collapse, upon activation, which involves removal of solvent from native materials either under vacuum or any desired atmosphere, up to 350 °C; (3) cycling of temperature, RH, vacuum, and gas atmospheres to gauge the reversibility of sorption (Figure 4). The cycling of atmospheres can be performed by either vacuum or atmosphere swing. The vacuum swing mode performs vacuum to atmosphere swings up to 1 atm of pressure. This can mimic adsorption/desorption isotherms with non-corrosive gases at fixed humidity up to 90% RH. In atmosphere swing mode, flowing atmospheres can be exchanged to evaluate adsorption competition between gases/adsorbates at fixed humidity up to 90% RH.

Figure 4. (Color online) Gas swing experiment (Figure 3) for CO2 loading in SBMOF-1 (Casdb) framework (Figure 1). Left: XRD patterns (background subtracted) at 2% relative humidity showing the change in relative intensities under N2 – CO2 atmosphere swings, confirming that CO2 is sorbing inside the channels. Right: strong exothermic and endothermic effects for experiment performed in 2% relative humidity (red) and 75% relative humidity (blue) at 298 K. Adapted from Plonka et al., Reference Plonka, Banerjee, Woerner, Zhang, Nijem, Chabal, Li and Parise2013b.
For the measurements, the powdered sample and DSC standard are loaded in separate aluminum pans (~10 mg each) on the XRD-DSC stage (Figure 3). Ideally, the stage is aligned so that the incident X-ray beam is only focused on the sample with the diffractometer in Bragg–Brentano geometry. Parallel beam geometry could also be used if the sample surface is uneven or exceedingly rough. If the sample and DSC standard pans are set too close or are over filled with powder, the XRD patterns may be contaminated by reflections from the DSC standard. The 2θ coverage is limited to 5° ≤ 2θ ≤ 40° by the size of the aluminum-coated kapton® polyimide windows on the XRD-DSC stage cap. However, this low-angle range is ideal for investigating microporous materials since it is most sensitive to changes in occupancy of extra-framework pore site by guest molecule sorption and desorption.
III. RESULTS AND DISCUSSION
A. Characterization of gas binding in SBMOF-1 using vacuum-atmosphere swing
Insights into the structure and thermal consequences of the competitive binding of gases at an unusual bi-phenyl site (Figure 1) in SBMOF-1 were provided by single-crystal diffraction in combination with XRD-DSC. The XRD-DSC signatures for CO2 adsorption at 2 and 75% RH during a gas swing adsorption experiment are shown in Figure 4. During the experiment, the atmosphere was alternated between flowing N2 and CO2 in several cycles allowing the system to equilibrate after each change. The XRD results (Figure 4) show that the crystallinity and the performance of CO2 adsorption is not diminished over at least 5 cycles (12 h) in either dry (2% RH) or humid conditions (75% RH). Strong exothermic effects, observed after changing the atmosphere from N2 to CO2, in both dry and humid conditions, indicate that the CO2 competes very effectively with water for adsorption at the phenyl pockets (Figure 1).
In the XRD patterns, the low-angle peaks of 10ī, 002, and 101 reproducibly decrease in comparison to the rest of the pattern suggesting that CO2 occupies space within the pore, consistent with the proposed CO2 adsorption mechanism (Figure 4). Vacuum swing DSC measurements were used to determine the differential enthalpy of adsorption, which was 10.221(81) and 0.779(26) kJ mol−1 for CO2 and N2, respectively. These values can be compared with those derived from measurements of the isotherms (Banerjee et al., Reference Banerjee, Zhang, Plonka, Li and Parise2012) that yield calculated enthalpies of adsorption of 10.56 and 0.811 kJ mol−1 for CO2 and N2, respectively. The good agreement confirms the value of vacuum swing DSC measurements as a screening technique.
B. Structure-thermal response to variable pressure vacuum-atmosphere swing
Guest-induced gate-opening transitions (Aguado et al., Reference Aguado, Bergeret, Titus, Moizan, Nieto-Draghi, Bats and Farrusseng2011; Banerjee et al., Reference Banerjee, Wang, Plonka, Emge, Parise and Li2016b) in MOFs are a sub-set of more general phenomena in microporous materials (Parise et al., Reference Parise, Corbin and Abrams1995; Lee et al., Reference Lee, Carr and Parise1998) involving adjustments to the pore geometry in response to interactions between adsorbate-framework and/or adsorbate-extra-framework species. Although the framework response typically results in volume expansion, and an abrupt increase in the adsorbate uptake, volume contraction upon adsorption has also been reported (Lee et al., Reference Lee, Vogt, Hriljac, Parise, Hanson and Kim2002). XRD-DSC (Woerner et al., Reference Woerner, Plonka, Chen, Banerjee, Thallapally and Parise2016) operating in vacuum-variable pressure mode is a particularly useful tool to rapidly evaluate the contributions of humidity and gas pressure to gate-opening structural transitions.
Using XRD-DSC techniques, we mimicked isotherm experiments at room temperature under both dry conditions and a CO2 atmosphere with 75% RH. We measured the DSC signal and collected XRD patterns while dosing 0.1 atm CO2 at intervals of 20 min: going from vacuum to 1 atm and back to vacuum (Figure 5). ZIF-7 has a sodalite cage structure and upon absorption of CO2 undergoes a gate-opening transition during adsorption with a hysteresis during desorption (Figure 5). The effect of temperature on the CO2 adsorption of ZIF-7 is well known, as the onset pressure of the gate opening and degree of hysteresis both increase with temperature (Zhao et al., Reference Zhao, Lampronti, Lloyd, Suard and Redfern2014). However, the effect of water vapor is revealed in a series of XRD-DSC measurements (Figure 5). The presence of water vapor not only reduces the measured enthalpy and therefore capacity of ZIF-7 (85.2% of dry), it shifts the onset of the gate opening and closing by +0.1 atm (Figure 5).

Figure 5. (a) (Color online) The 2D powder XRD patterns of ZIF-7 during the 0.1 atm titration of CO2 under dry and (b) 75% RH conditions as a function of pressure (right). The pressure of CO2 atmosphere on the sample during the XRD collection increases at 0.1 atm intervals from vacuum until 1 atm is reached, at which point pressure decreases in 0.1 atm intervals to vacuum. Abrupt shifts are noted in the vicinity of 0.4 atm, with differences between dry and 75% RH runs. (c) The DSC signal from dosing 11 mg of ZIF-7 with CO2 at 0.1 atm intervals (every 20 min) going from vacuum to 1 atm, and back down to vacuum, all under dry conditions. (d) Cumulative enthalpy plot of CO2 adsorption – desorption under dry and 75% RH conditions. Closed symbols are from enthalpy measurements during adsorption, and open symbols are from desorption enthalpy measurements. Adapted from previously published work (Woerner et al., Reference Woerner, Plonka, Chen, Banerjee, Thallapally and Parise2016).
C. Evaluation of novel MOFs
The search for novel MOFs with the biphenyl moiety (Figure 1) and their preliminary evaluation proceeded according to Figure 6. Powder XRD confirmed the ZnL1 compound obtained as described in the synthesis section above was novel and that upon activation in air at 280 °C the structure undergoes drastic changes in the powder XRD (Figure 7) indicative of structural changes. Large changes in the XRD of MOFs during activation do not necessarily indicate loss of 3D-connectivity, and only careful structure determination can distinguish structural collapse from structural flexibility. Subsequent structure determination (Figure 7) confirmed the 2D nature of ZnL1, consistent with the changes observed with XRD-DSC, which suggested the structure of the coordination polymer ZnL1 does not possess 3D-connectivity. The asymmetric unit of ZnL1 contains two zinc centers, with Zn1 and Zn2 octahedrally and tetrahedrally coordinated, respectively. Both Zn centers are coordinated by both carboxylates from L1 and by water molecules; loss of the later during activation probably leads to structural collapse.
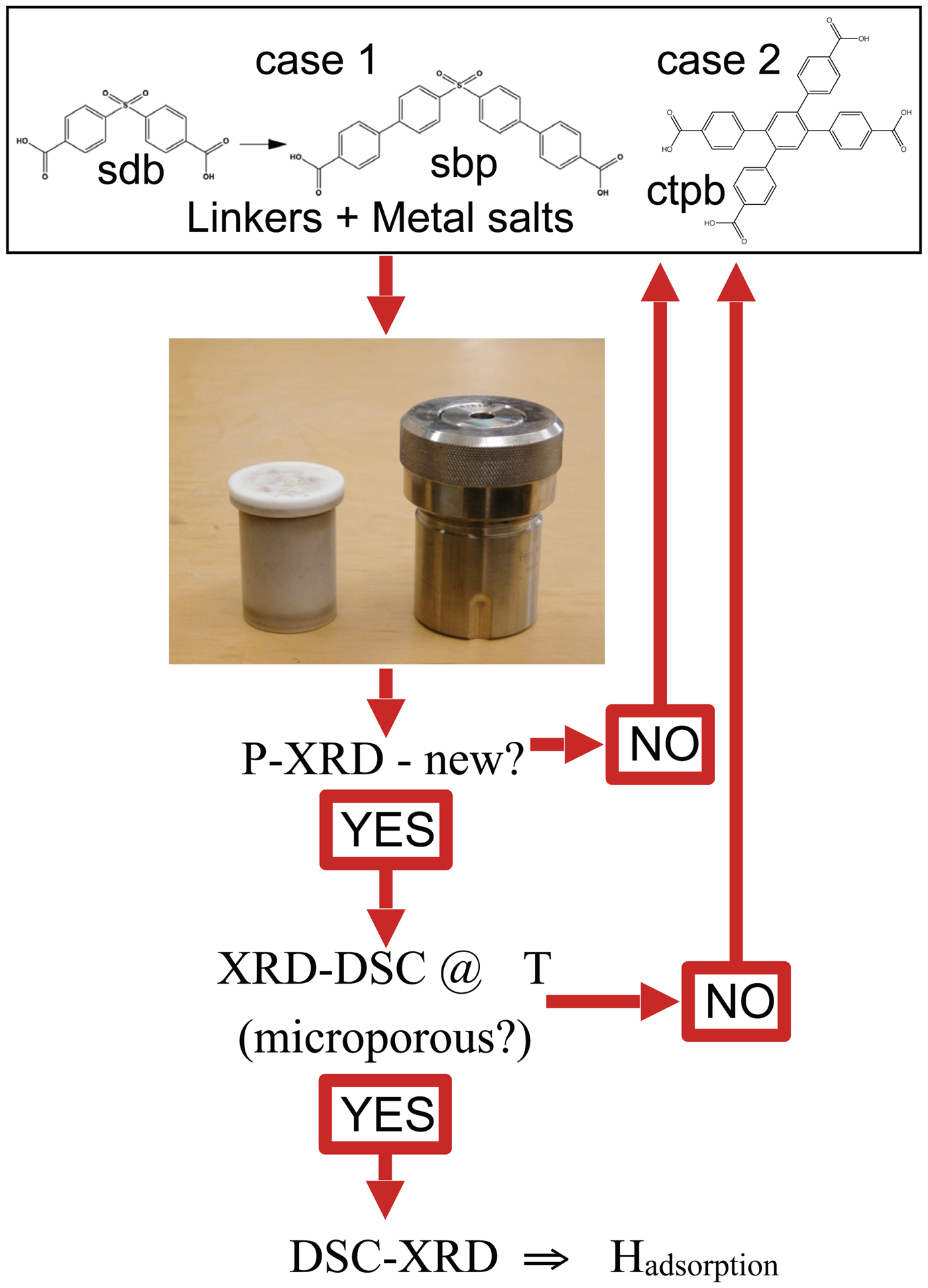
Figure 6. (Color online) Schematic of how DSC-XRD is integrated into a program of exploratory synthesis, in this case to discover new microporous MOFs capable of selective sorption of gases from humid gas streams, based on the observation that a biphenyl “π-pocket” provides a binding site (Figure 1) that promotes such selectivity.

Figure 7. (Color online) PXRD patterns of ZnL1 with stoichiometry Zn3(L1)2; L1 = 4′,4″-sulfonylbis([1,1′-biphenyl]-4-carboxylic acid); C2/m; a = 23.731(4) Å, b = 20.428(4) Å, c = 6.2272(5) Å, β = 95.619(3)° in as-synthesized form (bold black line) and of a sample activated at 280 °C (red dashed line). Indexing of the pattern, from the single-crystal structure determination (inset) reveals impurity phase(s) designated Im. The structure of native (unactivated) ZnL1, shown in the inset, contains zinc oxide polyhedral clusters (purple) cross-linked by L1 to form un-connected chains along [001]. Red, black, and yellow spheres represent oxygen, carbon, and sulfur, respectively.
The structure of ZnL1 (Figure 7) clearly contains the “π pocket” structural feature sought, but the procedure outlined in Figure 6 quickly identified this material as unsuitable. Similar procedures were used to synthesize many other new coordination networks and we randomly checked the structures for a few of the novel materials obtained, such as coordination polymers of Cd and Na (CdL1 and NaL2) including their solvent exchanged forms. Characterization of the structural collapse upon activation (Figure 7) for all compounds in this series forestalled attempts to explore gas sorption properties, since structural integrity upon activation is a prerequisite to the microporosity required for selective and reversible sorption of gases. Greater success was achieved from the use of commercially available linker, and this is described below.
D. Rapid evaluation C2Hn sorption properties of SBMOF-2
As described in Cases A and C above, preliminary studies of the humidity dependence of CO2-adsorption in SBMOF-1 prompted a search for microporous MOFs with phenyl moieties that might be capable of selectively absorbing gases in the presence of high RH. In addition to those materials screened in Case C, several novel solids were recovered from reactions that utilized ctpb (Figure 2) as a linker. One recovered from the solvothermal reaction of calcium nitrate and ctpb (Figure 8) was found to be microporous and this material was studied further for its gas sorption properties using XRD-DSC. Since SBMOF-1 was the inspiration for the synthesis of this new material, designated SBMOF-2, we present comparative studies of both. The set-up shown schematically in Figure 3 was coupled to a “corral” of gases that could be mixed in a gas manifold and passed over activated solids; the details of vacuum-swing procedure are described above and in Figure 3. The complete details of the synthesis and optimization of SBMOF-2 is described in the literature (Chen et al., Reference Chen, Plonka, Banerjee, Krishna, Schaef, Ghose, Thallapally and Parise2015).

Figure 8. (Color online) Structure of activated SBMOF-2 (Chen et al., Reference Chen, Plonka, Banerjee, Krishna, Schaef, Ghose, Thallapally and Parise2015) [P1−, unit cell, outlined in blue, with origin in the bottom right-hand corner with y up and z to the left; a = 5.1011(3) Å, b = 10.8715(9) Å, c = 15.2363(8) Å, α = 83.132(5)°, β = 85.982(4)°, γ = 83.032(5)°] showing channels running along the [100] direction. Calcium oxide octahedra are presented as blue polyhedra, and oxygen, hydrogen, and carbon atoms as red, pink, and black spheres, respectively. Except for hydrogen in the OH-moiety, all H atoms are omitted for clarity. The two types of channels, ones containing and others not containing OH, are marked I and II, respectively.
As an example of the versatility of the XRD-DSC set-up (Figure 3), the changes occurring upon activation and predictions of the ability of SBMOF-2 to separate C1- and C4-hydrocarbons were explored (Plonka et al., Reference Plonka, Chen, Wang, Krishna, Dong, Banerjee, Woerner, Han, Li and Parise2016). The results obtained for C1- and C2-hydrocarbons are summarized in Figure 9. In situ PXRD diffraction patterns of SBMOF-2 confirm the adsorption mechanisms determined with the single-crystal diffraction (Plonka et al., Reference Plonka, Chen, Wang, Krishna, Dong, Banerjee, Woerner, Han, Li and Parise2016). The decrease in low-angle peak intensity (Figure 9) with respect to higher angle reflections is consistent with the gas molecules occupying the pore space (Figure 8). Further, in contrast with SBMOF-1, the framework of SBMOF-2 is flexible, as evidenced in the shifting of peak positions in the PXRD patterns [Figure 9(a)] upon sorption of C2-hydrocarbons.

Figure 9. (a) (Color online) In situ PXRD patterns of SBMOF-2:C2Hn collected at 1 atm pressure of C2 hydrocarbons. The bold black patterns represent activated samples. (b) DSC signals measured upon loading activated SBMOF-2 with C1-C2 gases. Adapted from Figures 8 and S18 of previously published work (Plonka et al., Reference Plonka, Chen, Wang, Krishna, Dong, Banerjee, Woerner, Han, Li and Parise2016).
DSC measurements were performed using 9–10.5 mg of the sample in an aluminum crucible, as described in the experimental section above. Vacuum-swing measurements on SBMOF-2 were conducted to determine the differential enthalpy between empty and gas-loaded material for the adsorption of C2-hydrocarbons (ethane, ethylene, and acetylene) at 298 K and 1 atm. After activation at 523 K under vacuum, the XRD-DSC chamber was pressurized to 1 bar of hydrocarbon gas over the course of 15 s. When the DSC signal returned to baseline, after about 10 min, the chamber was evacuated to vacuum over the course of 15 s, which was sufficient to fully remove all sorbed gas; a total of 6–8 cycles of vacuum-swing were completed.
The differential enthalpy of adsorption (ΔH, kJ molMOF−1) and isosteric heat of adsorption values (Q st, kJ molGAS−1) were calculated (Table I) by fitting the gas adsorption isotherms, collected at two different temperatures, to the Langmuir–Freundlich equation, or virial equation (Suh et al., Reference Suh, Park, Prasad and Lim2012) and by direct measurement experimentally with DSC. The values of Q st obtained with DSC and virial methods are in a good agreement and all C2Hn display similar values of Q st (Table I). However, we can see that ethane interacts with the pore surface with the highest energy of the three, in spite of the full saturation of the C–C bond, normally leading to the lower adsorbent–adsorbate energy (Tsuzuki and Fujii, Reference Tsuzuki and Fujii2008) suggesting that it is the size of the molecule and the number of the H-pore surface interactions, rather than the saturation of the C–C bond, that are important in determining the energy of adsorption.
Table I. Comparison of calculated and experimental enthalpies (ΔH) and the heat of adsorption (Q st) of C2Hn in SBMOF-2. Adapted from previously published work (Plonka et al., Reference Plonka, Chen, Wang, Krishna, Dong, Banerjee, Woerner, Han, Li and Parise2016).

IV. CONCLUSION
The utility of the simultaneous measurement of the XRD-DSC system (Figure 3) lies chiefly in the versatility of the technique and as a supplementary screening tool, rather than as a substitute for more precise measurements. Isotherms (Suh et al., Reference Suh, Park, Prasad and Lim2012) breakthrough experiments (Chen et al., Reference Chen, Plonka, Banerjee, Krishna, Schaef, Ghose, Thallapally and Parise2015) and measurements of neutron (Brown et al., Reference Brown, Liu and Neumann2008) and synchrotron-based XRD provide the sorption capacity, selectivity, and sorption mechanisms, respectively. However, measurements of isotherms and breakthrough are time consuming, while data collection time at national facilities is at a premium, and so studies there should be carried out on optimized samples, with the screening of those samples carried out beforehand. The results obtained from the XRD-DSC apparatus described above provides the basis for prioritizing the most promising materials to target, thereby greatly increasing throughput and the likelihood of more rapidly identifying breakthrough materials. In one sample loading: (1) the stability of materials upon activation (solvent removal) can be evaluated as a function of temperature, gas, and humidity and (2) atmosphere–atmosphere and vacuum–atmosphere swings, with or without variable RH, can be used to accurately predict heats of adsorption and selectivity. As resources become scare, new materials with ever better functionality will become necessary, and not just desirable. Simultaneous XRD-DSC is one approach that is part of a broader effort to decrease the discovery-deployment cycle by developing the infrastructure necessary for more rapidly identifying and evaluating functional materials discovered serendipitously, from intuition-based extrapolation from previous results, and de novo by design.
ACKNOWLEDGEMENTS
The XRD-DSC experiments were made possible by equipment acquisition grants from NSF (EAR-0744230) and NASA (MFRP07-0022). NASA continues to support this facility through grant 80NSSC18K0535.