Introduction
Coleoid cephalopods, typified by squids, cuttlefish, and octopuses, are important components of modern marine ecosystems (see, e.g., Clarke Reference Clarke1996). Despite low biological diversity (<1000 species), they occur in nearly all habitats from inshore grass flats and coral reefs to the remotest areas of the open ocean, from surface layers to the bathypelagial and bathyal. Different species exhibit benthic, planktonic, or nektonic lifestyles. Cephalopods generally occupy the same set of ecological niches as teleost fishes, interacting with them as predators, prey, and competitors throughout evolutionary history (Packard Reference Packard1972; Tanner et al. Reference Tanner, Fuchs, Winkelmann, Gilbert, Pankey, Ribeiro, Kocot, Halanych, Oakley, da Fonseca, Pisani and Vinther2017). This led to a kind of “parallel evolution” from heavily armored ancestors with an external shell/skeleton and large eggs to modern fast-swimming teleosts and coleoids with internal skeleton/shell only and a range of reproductive strategies based on small to medium-sized eggs (Laptikhovsky et al. Reference Laptikhovsky, Nikolaeva and Rogov2017). However, the cephalopod Bauplan, that of a mollusk rather than a vertebrate, provided them with a very different set of morphological possibilities to solve particular evolutionary and ecological problems. One of the respective differences between coleoid cephalopods and teleost fishes is the high metabolic and growth rates of the former (Seibel Reference Seibel2007), allowing them to provide a quick population response to environmental changes and to occupy vacant spaces in ecological niches that appeared, either due to climatic shifts or to human activities (primarily fisheries). In recent decades, this has been demonstrated by a recent global increase in cephalopod stocks in very different ecosystems (Doubleday et al. Reference Doubleday, Prowse, Arkhipkin, Pierce, Semmens, Steer, Leporati, Lourenço, Quetglas, Sauer and Gillanders2016).
Fast reproduction and high fluctuation in abundance are the main life-history traits that appeared in modern coleoids as results of long evolutionary history going through many climate changes and mass extinctions. A tool to adapt to these challenges was egg size, which is an integral feature of reproductive strategy as a parameter inversely related to fecundity due to the trade-off between quantity and quality of offspring (e.g., Olofsson et al. Reference Olofsson, Ripa and Jonzén2009).
Measurement of egg size does not pose a problem in recent cephalopods, as ripe ovulated eggs are normally accumulated in the oviducts of squid and cuttlefish or can be collected from freshly laid egg masses of octopods. The size of freshly deposited eggs is nearly the same as at ovulation, as the perivitelline space appearing at fertilization is very small (Fig. 1). During embryonic development, egg size increases drastically, for example, from 1 mm to 2.5 mm in the oegopsid Illex argentinus (Sakai et al. Reference Sakai, Bruneiti, Elena and Sakurai1998), from 2.0–2.5 mm to 4.7–6.2 mm in the myopsid squid Doryteuthis gahi (Arkhipkin et al. Reference Arkhipkin, Laptikhovsky and Middleton2000), and from 3.8 mm to 6–7 mm in the octopus Octopus bimaculatus (Ambrose Reference Ambrose1981). This allows hatching of paralarvae, with a shell size about 10% larger than the length of an unfertilized egg (Laptikhovsky et al. Reference Laptikhovsky, Nikolaeva and Rogov2017), because in a swollen developed egg there is plenty of space for the embryo (Fig. 1). In modern coleoids the hatching mantle length (ML) corresponding to the embryonic shell (ES) length at hatching in most species is about equal to the egg size at fertilization (Laptikhovsky et al. Reference Laptikhovsky, Nikolaeva and Rogov2017).
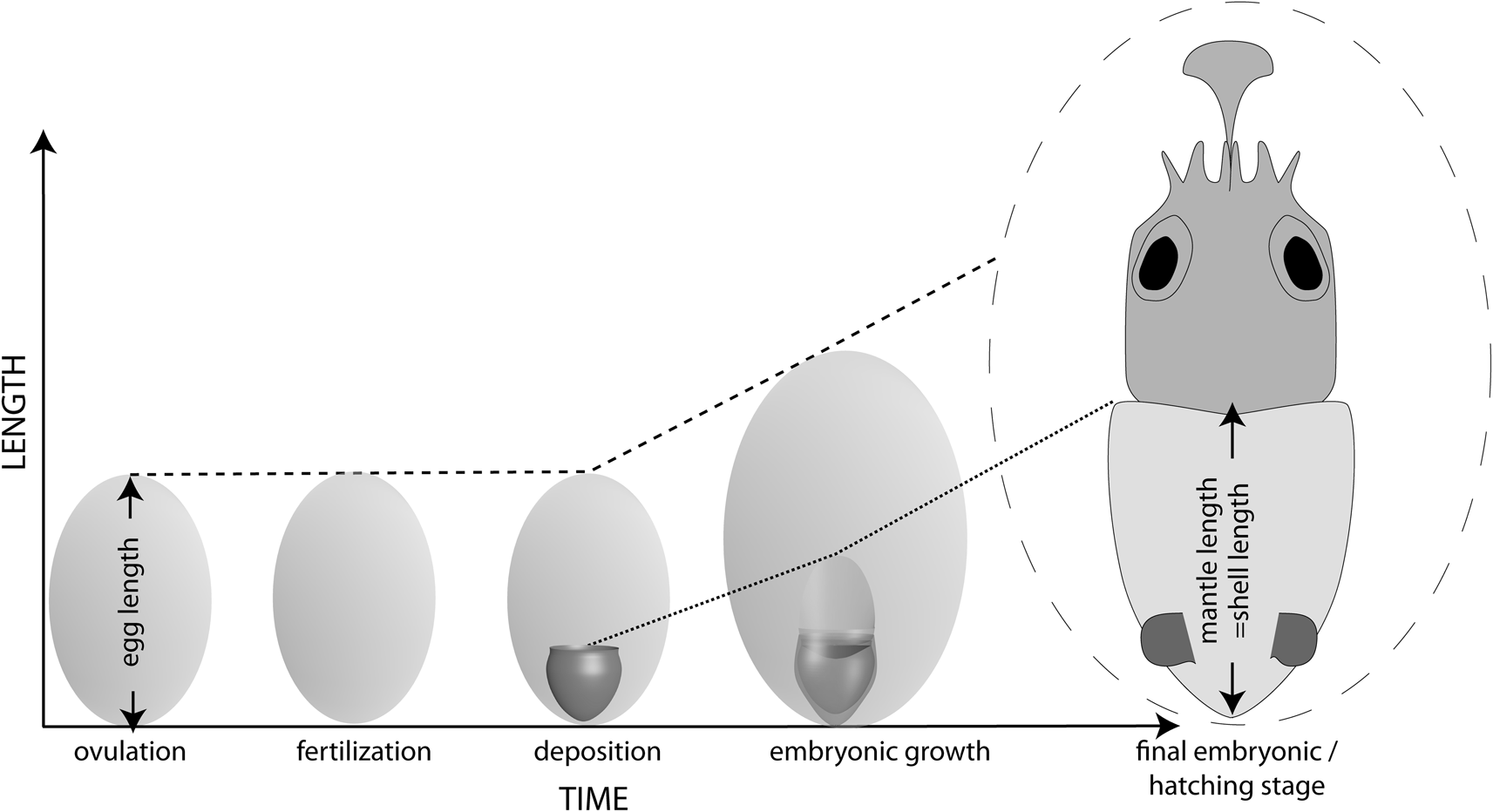
Figure 1. Schematic embryonic development of coleoid cephalopods. Indicated are egg growth and shell–mantle relationships.
Modern coleoids exhibit two types of reproductive strategies with a mean size of planktonic hatchlings (a proxy for egg size) of 2.5 ± 1.5 mm and a mean size of benthic hatchlings of 6.5 ± 4.6 mm (Villanueva et al. Reference Villanueva, Vidal, Fernández-álvarez and Nabhitabhata2016). The overlap between the two distinct strategies is caused by some flexibility in the life cycles, as well as by a bias caused by gigantism/dwarfism that gives giants the opportunity to produce relatively large eggs, whereas dwarfs lack such an opportunity just because of their body size.
Reports on ESs of extinct coleoids are frequent (e.g., Ward and Bandel Reference Ward, Bandel and Boyle1987; Doguzhaeva and Meléndez Reference Doguzhaeva and Meléndez2017; Tajika et al. Reference Tajika, Nützel and Klug2018), but the authors use different criteria for their identification, as coleoid ESs are mostly not clearly defined in contrast to the unambiguous ESs (ammonitellas) of ammonoids (De Baets et al. Reference De Baets, Landman, Tanabe, Klug, Korn, De Baets, Kruta and Mapes2015). Interestingly, the ES of the earliest ammonoids resembles that of coleoids in lacking a clear nepionic constriction (De Baets et al. Reference De Baets, Klug, Korn and Landman2012, Reference De Baets, Klug, Korn, Bartels and Poschmann2013). The ESs of early ammonoids and coleoids accordingly consist of the initial chamber (IC; protoconch in other terminologies) and a number of main chambers, from none to several, the consecutive numbering of which begins from 2 (e.g., IC, second chamber, third, etc.).
It is the purpose of the present contribution to reconsider existing ideas about the definition of the ES in coleoid cephalopods in order to reevaluate and reassess previous approaches and to set the standard for appropriate new measurements. Finally, we will use our results to infer evolutionary trajectories of coleoid reproductive strategies in geologic time to the present.
Material and Methods
Our comparative analysis of early coleoid phragmocones is based on a composite of literature research and new observations. New observations consider the early shells of 15 species (13 extinct/2 Recent) from five different coleoid orders (Aulacoceratida, Belemnitida, Diplobelida, Spirulida, and Sepiida). Stratigraphically, the fossilized specimens come from Triassic, Jurassic, Cretaceous, and Cenozoic deposits from all over the world (Table 1). Recent shells of Spirula spirula and Sepia officinalis come from the Indo-West Pacific and the Mediterranean Sea, respectively. Only one specimen per species was available for examination.
Table 1. Studied coleoid taxa, their systematics, ages, and origins.

The specimens—either internal molds (steinkerns) or longitudinal sections—were measured under a digital microscope and/or under scanning electron microscopy. Measurements were focused on the length of the IC and on the distance between the first eight mineralized septa (note the belemnoid closing membrane is not seen as a septum proper). It is essential to emphasize that only the dorsal septal distance was measured (Figs. 2–4). This practice is less important with orthoconic coleoids (i.e., forms with straight and parallel septa perpendicular to the longitudinal shell axis), but very helpful for comparisons of cyrto- or gyroconic spirulids and sepiids (hence forms with oblique and nonparallel septa) where ventral or lateral measurements are problematic. For comparisons, with early coleoids, we additionally interpolated the septal distances of Carboniferous Mutveiconites milleri based on Figure 1B provided by Doguzhaeva et al. (Reference Doguzhaeva, Mapes and Dunca2006). Finally, we expanded our matrix data with equivalent measures of belemnites Wani et al. (Reference Wani, Tajika, Ikuno and Iwasaki2018) conditionally determined as Passaloteuthis laevigata, Parapassaloteuthis zieteni, and Pseudohastites longiformis (respectively from the Pliensbachian of Germany and France). These identifications should all be considered with reservation.

Figure 2. Initial and subsequent chambers of spirulid and sepiid coleoids (magnification ×40). A, Spirula spirula, Recent, Mozambique; initial chamber (IC) complete; CH2L, length of chamber 2; ch4, chamber 4; ICL, IC length; s1-2, septal 1 and 2; SHL, shell length; dashed lines indicate measured lengths of two- and three-chambered shell. B, Spirula spirula, Recent, Mozambique; opened IC showing caecum and prosiphon. C, Sepia officinalis, Recent, embryonic cuttlebone with a single septum, dotted circle marks the outline of the growth increments–less shell primordium equivalent to the IC; CH1L, length of chamber 1; other abbreviations as in A. D, Sepia officinalis, Recent, longitudinal section of an early cuttlebone with nine septa; CH1H, high of chamber 1. E, Belosaepia sp., Eocene, France, internal cast; CH4L, length of chamber 4. F, Belosaepia sp., Eocene, France, scanning electron microscope image showing the cap-like IC; ch2, chamber 2. Scale bars, 1 mm.

Figure 3. Initial and subsequent chambers of spirulid coleoids (magnification ×40). A, Groenlandibelus rosenkrantzi (holotype), Upper Cretaceous (Maastrichtian), Greenland; longitudinal fracture; sh, sheath; other abbreviations as in Fig. 2. B, Cyrtobelus hornbyense, upper Cretaceous (Campanian), British Columbia, Canada; longitudinal fracture; ma, matrix. C, Beloptera belemnoidea, Eocene, France; internal cast. D, Spirulirostra hoernesi, Miocene, Germany; longitudinal section. Scale bars, 1mm.

Figure 4. Initial and subsequent chambers of belemnoid coleoids (magnification ×40). A, Aulacoceras sulcatum (Aulacoceratida), Upper Triassic (Carnian–Norian), Timor; longitudinal section; pr, primordial rostrum; ro, rostrum; other abbreviations as in Fig. 2. B, Belemnopsis sp. (Belemnitida, Belemnopseina), Middle Jurassic (Bajocian), Normandy, France; longitudinal fracture. C, Holcobelus sp. (Belemnitida, Belemnopseina), Middle Jurassic (Bajocian), Normandy, France; longitudinal fracture. D, Conobelus hayakawai (Diplobelida), upper Cretaceous (Cenomanian), Hokkaido, Japan; longitudinal section. Scale bars, 1 mm.
In coleoids with orthoconic shells, shell lengths at different growth stages can be calculated as the sum of the IC length and the septal distances. However, the total shell length additionally includes the length of the body chamber or proostracum, which left no clearly delimited shell markers. The very early formation of the organic proostracum is confirmed by observations of Bandel et al. (Reference Bandel, Engeser and Reitner1984), Doguzhaeva et al. (Reference Doguzhaeva, Mutvei and Weitschat2003), and Doguzhaeva and Meléndez (Reference Doguzhaeva and Meléndez2017). It is therefore necessary to estimate the total shell length at different growth stages. Our total shell length estimates for two- to seven-chambered shells are based on the assumptions that the tubular body chambers of primitive belemnoids (Mutveiconites and Aulacoceras) as well as the proostracum of belemnitid, diplobelids, and spirulids did not undergo a distinct growth allometry. In belemnitids, the adult proostracum is, where known (e.g., Hibolithes and Acanthoteuthis), roughly as long as the chambered part (Fuchs Reference Fuchs, Arratia, Schultze, Tischlinger and Viohl2015: Fig. 455). In putative diplobelids Clarkeiteuthis and Chondroteuthis, it is slightly longer (approx. 60% of the total shell length) than the phragmocone (Fuchs et al. Reference Fuchs, Donovan and Keupp2013a; Jenny et al. Reference Jenny, Fuchs, Arkhipkin, Hauff and Klug2019). We therefore assumed that the length of early ontogenetic body chambers/proostraca were between 40% and 50% of the total shell length. Unfortunately, we do not know the proostracum length in early spirulids Groenlandibelus (Maastrichtian) and Cyrtobelus (Campanian). On the basis of similar shell morphologies between groenlandibelids and diplobelids (see Fuchs et al. Reference Fuchs, Keupp, Trask and Tanabe2012a,Reference Fuchs, Keupp and Wieseb, Reference Fuchs, Iba, Ifrim, Nishimura, Kennedy, Keupp, Stinnesbeck and Tanabe2013b), we presume the same relative length as in belemnitids and diplobelids. Proostracum length estimates are not necessary in sepiids and Cenozoic spirulids, as their cyrto- and gyroconic shells lack a forward-projecting proostracum (Table 2). Their total shell lengths can therefore be directly measured as the maximum distance between dorsal rim of the final chamber (anteriormost shell extension) and the earliest chambers (posteriormost shell extension) (Fig. 4). Another uncertain variable in our hatching shell length estimation is the very inconsistent length of the primordial rostrum apex. It can occupy between 10–30% of the IC length (see Doguzhaeva et al. Reference Doguzhaeva, Weis, Delsate and Mariotti2014). However, taking into account that the septal part of the ES is generally much longer than the IC in coleoids, ignoring the primordial rostrum is unlikely to invoke an underestimation of the ES of more than 5–10%.
Table 2. Measured and estimated lengths of two- to seven-chambered shells. Measured lengths include only the shell length (SH L); estimated lengths include SH L plus the estimated body chamber (BC) or proostracum (PO) length. Two estimates are based on the assumption that BC/PO lengths occupies 40 or 50% of the total shell length. *Measurements based on Fig. 1B in Doguzhaeva et al. (Reference Doguzhaeva, Mapes and Dunca2006). **Medial measurements taken from Wani et al. (Reference Wani, Tajika, Ikuno and Iwasaki2018).

Known and Unknown Hatching Markers in Cephalopod Shells
Hatching Markers in Ectocochleate Cephalopods
The morphology of ESs of cephalopods with external shells, both extinct and extant (Nautilus) was recently revised (Laptikhovsky et al. Reference Laptikhovsky, Nikolaeva and Rogov2017). The hatching event is often (if not mostly) well defined in their morphology. The nepionic constriction is present in some bactritoids and Nautilida as a wide constriction in the early part of the shell. Another kind of constriction (primary constriction) was found in derived ammonoids and is accompanied by the primary varix, indicating the appearance of the nacreous layer. The septal approximation (decrease in the distance between septa) is known in many nautilids and is commonly used for recognition of the ES. Changes in micro-sculpture are rarely seen in fossil ectocochleates, as this relies on excellent preservation, but they could also be used for this purpose (De Baets et al. Reference De Baets, Landman, Tanabe, Klug, Korn, De Baets, Kruta and Mapes2015; Turek and Manda Reference Turek and Manda2016).
Hatching Markers in Modern Coleoids
The mineralized shells of extant Sepia and Spirula are devoid of any structural feature that allows the end of the embryonic phase to be unambiguously defined, and only egg incubation might provide information on the ES at hatching. Also, the nonmineralized shell remains of squids, the gladii, show no distinct evidence of hatching markers. Among other hard structures, hatching marks are found on statoliths of Decabrachia and on stylets (vestigial shells) of Octobrachia, but they do not provide any evidence of the hatchling size (Arkhipkin et al. Reference Arkhipkin, Bizikov, Doubleday, Laptikhovsky, Lishchenko, Perales-Raya and Hollyman2018).
Spirula spirula (Fig. 2A,B) is one of the few exceptions, where the ramshorn-shaped shell does not occupy the full ML. Isotopic signatures in the shells that possess the IC (Lukeneder et al. Reference Lukeneder, Harzhauser, Müllegger and Piller2008; Warnke et al. Reference Warnke, Oppelt and Hoffmann2010) suggest that hatchlings emerge from the egg with the shell containing two to three chambers (IC = 0.6–0.7 mm), which agrees well with captures of the youngest specimens with 2–3 mm ML and a two-chambered shell (Chun Reference Chun1914; Bruun Reference Bruun1943; Clarke Reference Clarke1970). Similarly, Yamaguchi et al. (Reference Yamaguchi, Kumada, Alfaro and Wani2015) correlated lower rotational angles between septa 1 and 3 with hatching.
The dorsal shield of the sepiid cuttlebone (i.e., Sepia officinalis) includes a posterior growth increment-less structure (Fig. 2C,D). It is only visible in ESs before the secretion of the spine. This shell primordium is disk-shaped, approx. 0.8 mm in diameter and considered to be equivalent to the IC (Bandel and Boletzky Reference Bandel and Boletzky1979). Sepia hatches with seven to eight septa and both ripe egg size and hatchling ES is some 6–8 mm (Boletzky Reference Boletzky and Boyle1983). According to Yamaguchi et al. (Reference Yamaguchi, Kumada, Alfaro and Wani2015), hatching in Sepiella japonica is indicated by distinctly decreased septal distances around chamber 8.
Hatching Markers in Extinct Coleoids
Although innumerable shells of extinct coleoids have been examined so far, we are apparently unable to reliably distinguish between embryonic and post-ES parts. Nevertheless, a range of potential hatching markers has been proposed for at least some species.
IC Equivalent to ES
The absence of an unambiguous border between embryonic and postembryonic stages, with the only constriction being between the IC and the phragmocone (Figs. 2–4), leads to the suggestion that the belemnite ES consisted only of the IC (Wani et al. Reference Wani, Tajika, Ikuno and Iwasaki2018). In the majority of extinct coleoids, the IC is separated from the phragmocone through a constriction with no further constriction to define much larger ES (Figs. 2–4). This main constriction between the IC and the second chamber is said to correspond to the separation of the inner and outer yolk sacs, which happens comparatively early in embryogenesis (Boletzky Reference Boletzky1974; Bandel Reference Bandel1982).
Other Shell Constrictions
The previously mentioned sharp constriction between the initial and following chambers does not exist in most ectocochleate cephalopods. This main constriction is certainly not an equivalent to the nepionic constriction, which marks the end of the embryonic phase in ammonoids and bactritoids as suggested by Wani et al. (Reference Wani, Tajika, Ikuno and Iwasaki2018). If the outer surface of coleoid shells bear any indication of a second (“nepionic”) constriction, this signature would have been in later ontogenetic stages compensated by the deposition of secondary (= rostral) layers on the outer surface of the early shell. In other words, a thin juvenile rostrum would hide a potential constriction. Hence, only longitudinal sections showing the internal side of the shell wall may offer a constriction related to the point of hatching. Hewitt and Jagt (Reference Hewitt and Jagt1999) reported a second constriction in the spirulid-like Cyrtobelus (their Groenlandibelus; see Fuchs et al. Reference Fuchs, Keupp, Trask and Tanabe2012a). However, a reinvestigation of Cyrtobelus by Fuchs et al. (Reference Fuchs, Keupp, Trask and Tanabe2012a) shows that the assumed constriction is caused by an artifact. Finding of the “nepionic” constriction between the initial and subsequent chamber was also claimed for the Eocene sepiid Belosaepia (Meyer Reference Meyer1993). However, Yancey et al. (Reference Yancey, Garvie and Wicksten2010) showed that such a constriction does not exist, which is confirmed by our observations.
Interpolations from Initial Chamber Size
Doguzhaeva et al. (Reference Doguzhaeva, Weis, Delsate and Mariotti2014) concluded that a constriction indicating the point of hatching, as in ammonoids, is absent in coleoid taxa. They suggested that belemnites with an IC of 0.3–1.0 mm (mostly 0.3–0.5 mm) might have both an ES and egg size of 1.5–3 mm. Recently, without documented evidence, it was assumed that Jurassic belemnites hatched with an IC and one or two chambers of the phragmocone (hence two to three septa), a primordial rostrum, and a proostracum, the entire ES being 1.5–2 mm (Doguzhaeva and Meléndez Reference Doguzhaeva and Meléndez2017).
Protoconch–Teleoconch (Architectural) Separation
In belemnoids, the main constriction between the initial and the second chamber is associated with an architectural separation. Therefore, Fuchs (Reference Fuchs2019) recently divided the belemnoid phragmocone into the IC (= protoconch) and the teleoconch, as for other conchiferan mollusks. This separation in belemnoid shell architecture was demonstrated for the first time by Bandel et al. (Reference Bandel, Engeser and Reitner1984). In belemnitids (and aulacoceratids, where known), the wall of the IC tapers out near its constricted aperture and the prismatic mural part of the first (mineralized) septum continues as the conotheca (Doguzhaeva et al. Reference Doguzhaeva, Mutvei and Weitschat2003, Reference Doguzhaeva, Weis, Delsate and Mariotti2014; Fuchs et al. Reference Fuchs, Keupp, Trask and Tanabe2012a; Fuchs Reference Fuchs2019). Fuchs et al. (Reference Fuchs, Keupp and Wiese2012b) observed a similar morphology in the diplobelid Conoteuthis. Hence, the belemnoid (aulacoceratid, belemnitid, and diplobelid) shell is composed of two shell units, the IC and the teleoconch separated from each other by a sharp growth boundary. In other words, the first prismatic septum becomes the prospective conotheca to which all subsequent lamello-fibrillar septa attach. In non-belemnoid (spirulid and sepiid) shells, such a clear partition is absent.
Decreasing Shell Diameter
Bactritoids, direct ancestors of coleoids with a presumed external shell, often but not always possessed a well-defined ES consisting of three growth stages (Mapes Reference Mapes1979), which included the IC (0.3–0.7 to 1.5 mm), the second chamber, and subsequent chambers 3–8 (ES = 1–3 mm). The last embryonic stage is characterized by a decreasing shell diameter, while an increasing shell diameter presumably indicates the hatching stage. A decreasing shell diameter together with septal spacing has also been used to infer embryonic stages of early ammonoid shells where a clear nepionic constriction is missing (De Baets et al. Reference De Baets, Klug, Korn and Landman2012, Reference De Baets, Klug, Korn, Bartels and Poschmann2013, Reference De Baets, Landman, Tanabe, Klug, Korn, De Baets, Kruta and Mapes2015). However, such a shift in shell diameter is unknown in fossil coleoids.
Changes in Shell Ornamentation
Outer shell ornamentation indicative of ontogenetic phases is unknown in fossil coleoids.
Ultrastructural Markers
A sudden shift in the ultrastructural texture of the shell layers, as can be observed in ectocochleates (e.g., appearance of a nacreous layer), has not yet been reported in coleoids. However, none of the workers who have so far studied early ontogenetic shell stages recognized a layer of tabular nacre in the belemnoid conotheca. This suggests that nacre possibly first appeared at multicameral (juvenile) stages, well after hatching. In non-belemnoids, tabular nacre is generally absent.
In belemnites, early septum formation is subject to an ultrastructural shift. The first mineralized septum is prismatic and continuous as the unilayered conotheca (discussed earlier). From the second septum onward, belemnitid septa are composed of lamello-fibrillar nacre. In non-belemnoids, in contrast, there is no interruption between the wall of the IC and the wall of the second chamber (i.e., protoconch–teleoconch separation is absent; see “Protoconch–Teleoconch [Architectural] Separation”), and the first septum is already lamello-fibrillar, as in Sepia and Spirula.
Shell Thickening
Hewitt and Jagt (Reference Hewitt and Jagt1999) found that in a juvenile shell of the belemnite Cylindroteuthis (IC = 0.5 mm), there are a ventral thickening and a dorsal shift in the axis of the belemnite around the eighth calcified septum at a shell length of 1.3 mm. Apart from this assumption, there are no further reports on a shift in shell swellings in fossil coleoids.
Shift in Septal Spacing (= Chamber Length)
A shift in septal spacing is a common hatchling marker in extinct ectocochleate cephalopods (e.g., Landman et al. Reference Landman, Rye and Sheldon1983; Wani and Mapes Reference Wani and Mapes2010; Arai and Wani Reference Arai and Wani2012) as well as extant sepiid coleoids (e.g., Yamaguchi et al. Reference Yamaguchi, Kumada, Alfaro and Wani2015; Wani et al. Reference Wani, Tajika, Ikuno and Iwasaki2018 and references therein). Although Wani et al. (Reference Wani, Tajika, Ikuno and Iwasaki2018) found no significant irregularities in septal spacing in three belemnitid species, earlier workers recorded variable septum densities in various extinct coleoids. For instance, changes in dorsal spacing of the sutures led Hewitt and Jagt (Reference Hewitt and Jagt1999) to assume that Cylindroteuthis (Belemnitida) might have hatched at the septum 2 or 3 stage.
The ES of sepiids (Fig. 2C,D) is unique in many ways. One novelty concerns the extensions of the IC, whose longitudinal axis becomes progressively shorter than the sagittal axis, resulting in an evolution of the IC from a hemisphere (Ceratisepia) via a low cap (Belosaepia; Fig. 2E,F) to a flat disk (Sepia). Also, in contrast to belemnoids and spirulids, the septa became progressively inclined; that is, the ventral septal distance is strongly reduced, whereas the dorsal distance is distinctly extended. The Paleocene Ceratisepia elongata showed a sudden reduction in the spacing of septa 3 and 4 at a shell length varying between 2.8 and 3.7 mm (Meyer [Reference Meyer1993] as interpreted by Hewitt and Jagt [Reference Hewitt and Jagt1999]). These authors also deduced—although without detailed explanation—that the Eocene Belosaepia hatched at ES = 5.3 mm.
Functional Morphology
Bandel et al. (Reference Bandel, Engeser and Reitner1984) reconstructed the point of hatching on the basis of functional morphology. They argued that the belemnite Hibolithes (IC = 0.44 mm) hatched as soon as the IC was sealed by the organic closing membrane and the IC was emptied. The animal consequently hatched with a shell consisting of a single buoyant chamber. Bandel et al. (Reference Bandel, Engeser and Reitner1984) reconstructed an ES of 1.5 mm length.
Onset of Rostrum Formation
The belemnoid primordial rostrum or the spirulid/sepiid sheath covering the IC and the conotheca is certainly a product of embryonic development (Fuchs Reference Fuchs2012). It is known that the deposition of the rostrum proper begins early in the ontogeny of aulacoceratids and belemnitids; its onset might therefore represent one of the first posthatching events. However, we are not presently able to correlate the exact onset of rostrum formation and the number of existing chambers. Apart from this, contemporary coleoid workers generally agree that belemnoids hatched without a rostrum proper (e.g., Bandel et al. Reference Bandel, Engeser and Reitner1984; Doguzhaeva and Meléndez Reference Doguzhaeva and Meléndez2017).
New Observations
A review of previous approaches shows that an unambiguous hatching marker, such as in ectocochleates (e.g., a sudden change in the shell diameter, a modification of the external shell surface ornamentation, or the presence of a distinct nepionic constriction) apparently does not exist in coleoid shells. In our opinion, the most promising character was the septal spacing. However, our approach failed to detect a clearly defined shift in septal distances—apart from irregularly occurring indistinct fluctuations most probably influenced by individual and/or environmental triggers (Table 2, Fig. 5A–C).

Figure 5. Initial chamber lengths and the length of seven to eight subsequent chambers (= septal distances) of belemnoids (A), spirulids (B), and sepiids (C).
With respect to early shell measurements, Groenlandibelus (Fig. 3A), Beloptera (Fig. 3C), and Spirulirostra (Fig. 3D) differ from belemnoids in having a strikingly enlarged IC (compare Figs. 3 and 4 with Fig. 5A,B). The IC in both spirulids and belemnoids is generally longer than many subsequent chambers, which lengthen at a slow rate over ontogeny. This is different in sepiids (Fig. 2C–F), where the chamber length underwent a severe reduction relative to the chamber diameter (see “Shift in Septal Spacing [ = Chamber Length]”), which becomes more and more dominant in the course of sepiid shell evolution.
In Eocene Belosaepia (Figs. 2E,F, and 5C), the length of the first chambers increases from the short bowl-shaped IC until chamber 4; then a decrease begins until chamber 7 before distances increase again. In Sepia (Fig. 2C,D), the “IC” (diameter = about 1 mm) is not equivalent to the IC of Belosaepia (and other phragmocone-bearing cephalopods), because the first septum attaches to the forward growing dorsal shield (rather than to the margins of the primordium), in which the first chamber is incorporated. The first chamber in Sepia is thus longer than the primordium and subsequent chambers, whose length decreases gradually until chamber 7 before an increasing trend begins (Fig. 5C).
Key Architectural Stages, Estimated Shell Lengths, and Their Implications for Hatching Stages
A congruent combination of shell growth modifications present on a wide systematic spectrum allows identification of three different key stages in the early shell morphogenesis of belemnoids and a single key stage in non-belemnoid coleoids. Total shell length estimates for various growth stages are compiled in Table 2 and Figure 6.

Figure 6. Estimated total shell lengths of belemnoids (shaded), spirulids, and sepiids from the IC to chamber 6. Key growth stages of their shells are reconstructed below.
Key Stage 1
Key stage 1 (only belemnoids) terminates with an organic closing membrane sealing the IC. Hatchlings therefore had a buoyant IC, which was externally covered by a still very thin primordial rostrum. At this stage, the hatchling shell was distinctly smaller than 1 mm.
Key Stage 2
Key stage 2 (only belemnoids; Fig. 6) is characterized by the initial formation of the teleoconch. The teleoconch wall consists of the first mineralized septum and its mural part, which continues anteriorly as the prospective shell wall (conotheca). The initial teleoconch can be tubular (Aulacoceratida) or dorsally projected (Belemnitida, Diplobelida). Therefore, key stage 2 includes a single functional (buoyant) chamber (i.e., the IC) and either a tubular body chamber or a dorsally projected proostracum (Fig. 6). This primary shell is enveloped by the primordial rostrum (a secondary shell formation), whose thickened apex slightly extends the total hatching shell length. The length of the prehatching teleoconch is probably not longer than the IC, indicating ESs of a maximum of 1.5 mm length (Fig. 6). The apex of the prismatic teleoconch (hence the first septum proper) almost touches the closing membrane, leaving no space for the “second” chamber, which is therefore usually disregarded as being a functional chamber. Regarding this one-chambered shell stage as the hatching stage would imply that the onset of secreting lamello-fibrillar septa is a posthatching event (see “Key Stage 3”).
The view that belemnoids hatched after formation of the teleoconch (Key Stage 2) is further corroborated by the fact that the longitudinal axis of the IC often differs from the phragmocone's (teleoconch) axis (Bandel et al. Reference Bandel, Engeser and Reitner1984). Though unknown in aulacoceratids, such deviation is to some extent developed in many belemnite taxa and is most striking in the diplobelid Conoteuthis (Fuchs et al. Reference Fuchs, Keupp and Wiese2012b). Wherever the two longitudinal axes deviate from each other, the IC is—as a rule—ventrally inclined. In belemnoids, the deviation concerns only the IC, whereas in spirulids and sepiids, successive chambers are also involved, which initiates coiling of at least the earliest chambers. This unique shift of the longitudinal body axis might be referred to as a shift of the body orientation.
Key Stage 3
Key stage 3 (Fig. 6) is marked by the appearance of lamello-fibrillar septa initially secreted either just before or just after hatching. Assuming belemnoids hatched with a two-chambered shell, their shells were no longer than 2 mm, implicating a small-egg strategy similar to recent oceanic oegopsids (Laptikhovsky Reference Laptikhovsky1999; Hoving et al. Reference Hoving, Laptikhovsky, Lipinski and Jurgens2014; Laptikhovsky et al. Reference Laptikhovsky, Fock, Piatkowski, Schwarz and Hoving2019).
With the exception of Aulacoceras, belemnoid shells with four chambers do not exceed the 2 mm boundary. Our estimation shows that the bulk of belemnoids crosses the 2 mm boundary with five to seven chambers. The eight-chambered shells of Parapassaloteuthis and Pseudohastites are still shorter than 2 mm.
In non-belemnoid spirulids and sepiids, the secretion of lamello-fibrillar septa represents the one and only key stage in their shell morphogenesis. In contrast to belemnoids, the first septum is lamello-fibrillar (rather than prismatic). In Spirula, hatching with a one-chambered shell is conceivable, but the multi-chambered Sepia hatchlings show that lamello-fibrillar septa here unambiguously represent embryonic secretion products and are thus a problematic means for detecting hatching markers (at least in sepiids).
If the secretion of lamello-fibrillar septa represented hatching markers in ancestral spirulids and sepiids, hatching would have occurred with a single functional (buoyant) chamber (i.e., the IC). Belosaepia, Beloptera, Spirula, Cyrtobelus, and Vasseuria—according to our estimates—possessed shells of less than 2 mm, whereas Spirulirostra (2.1 mm) is slightly and Groenlandibelus (approx. 4.28 mm) distinctly above the 2 mm boundary. Belosaepia and Beloptera reach the 2 mm boundary between chambers 2 (one buoyant) and 3 (two buoyant). Estimated shell lengths of Cyrtobelus and Vasseuria with three to seven chambers are similar to those of belemnoids. The measured shell lengths of Spirula show that this enigmatic deep-sea squid crosses the 2 mm boundary between chambers 3 and 4, implicating either hatching with two to three buoyant chambers and the small-egg strategy or hatching with more than three buoyant chambers and the large-egg strategy.
Discussion
As noted earlier, we consider potential hatching markers such as shell thickening or constrictions to be ambiguous, poorly understood, or extremely variable in coleoid cephalopods. The delayed appearance of nacre in the belemnoid conotheca as an ultrastructural marker as well as the onset of rostrum formation are subsequent milestones in the belemnoid ontogeny and thus are not hatching related.
Although the shift in septal spacing between embryonic and postembryonic stages is a reliable hatching marker in Sepia, it is less useful in phragmocone-bearing coleoids, particularly belemnoids and spirulids. Irregularities present in some belemnitids indicate that septal spacing as a hatching signal is not universal, extremely variable, and possibly individually related to both hatching and posthatching environmental factors. The lack of a septum-related hatching signal in belemnoids is congruent with Wani et al. (Reference Wani, Tajika, Ikuno and Iwasaki2018). The supposed absence of a decreasing trend in septal spacing led these authors to assume that belemnitid hatchlings were born with a single buoyant chamber. This idea was previously suggested by Bandel et al. (Reference Bandel, Engeser and Reitner1984). Accordingly, the first mineralized septum (i.e., the rear of the teleoconch) was supposed to be a posthatching development. Hatching at this earliest stage, however, appears unlikely for various reasons: (1) Eggs smaller than 0.6 mm do not occur in modern cephalopods. Acceptance of Bandel's hypothesis would mean that belemnoids generally produced eggs smaller than 0.5 mm, incomparably smaller than any extant or (with very few exceptions) extinct Cephalopoda and similar in size to those of gastropods and bivalves with a true larval stage and metamorphosis. In such a case, the belemnoid ontogeny would be fundamentally different from other coleoids. (2) Owing to the absence of a body chamber/proostracum, there would be no space to securely accommodate the animal's visceral mass. (3) A similarly distinct growth interruption between the IC and subsequent phragmocone (teleoconch) can also be observed in ammonoids and bactritoids (Doguzhaeva Reference Doguzhaeva2002), both of which are closest relatives of Coleoidea among the cephalopods, but in these groups the hatching occurs later, after the formation of the body chamber.
Focus on architectural growth stages shows that the formation of lamello-fibrillar septa represents a universal key stage that closes the second chamber in belemnoids and the IC in spirulids and sepiids. Belemnoids would accordingly have hatched with two buoyant chambers (including the IC). Belemnoid hatchlings with two to three chambers have previously been assumed by Doguzhaeva and Meléndez (Reference Doguzhaeva and Meléndez2017), and we consider this as the most likely scenario.
Nevertheless, our total shell length estimates also show that belemnoid hatchlings with more than two chambers are conceivable. In this case, the belemnoid shell would be devoid of any unambiguous hatching marker. Belemnoid hatchlings with four to five chambers would have followed the small-egg strategy; those with six or more chambers the large-egg strategy.
Setting the first formation of lamello-fibrillar septa as a hatching marker means that spirulids and (early) sepiids hatched with only a single buoyant chamber. This event is congruent with the only (main) shell constriction and the only obvious shift in chamber lengths. Modern Sepia demonstrates that this putative hatching marker is not universal, but the secretion of lamello-fibrillar septa during late embryogenesis in modern cuttlefishes might be seen as embryonization—a process of delayed hatching with the accomplishment within the egg of developmental processes otherwise occurring after hatching (Crowson Reference Crowson1981). It should be noted that hatching in modern cephalopods can occur at different embryonic stages, even within the same population (e.g., Sepia hatches with seven or eight septa). Stressful situations like rapid temperature changes lead to production of earlier but still viable hatchlings. This occurs because of the existence of a so-called phase of hatching competence rather than a morphologically and/or physiologically defined hatching stage that may optimize posthatching survival depending on the environmental situation (Boletzky Reference Boletzky2003). Therefore, it is not surprising to find that the number of septa at hatching varies slightly not only within the order, but even within the species.
How many buoyant chambers did ancestral sepiids have at hatching? Belosaepia exhibits an increasing–decreasing–increasing septal spacing trend. The first shift is at a four-chambered stage (total shell length = 5.32 mm); the second shift at a seven-chambered stage (total shell length = 10.51 mm). Both scenarios imply that Belosaepia produced large eggs like modern Sepia. If Belosaepia hatched after the formation of the first lamello-fibrillar septum, this typical Eocene sepiid produced small eggs (total shell length = 1.47 mm), but if Belosaepia hatched with two buoyant chambers (total shell length = 3.09 mm), these animals already laid large eggs.
The first evidence of a large-egg strategy is provided by Late Cretaceous groenlandibelid spirulids. In Groenlandibelus rosenkrantzi (Maastrichtian), the embryos most probably hatched immediately after the formation of the first septum, because the two-chambered shell was—according to our estimates—longer than 2 mm (total shell length = 2.14 mm). In contrast, the estimated shell lengths of the closely related and slightly older genus Cyrtobelus (Campanian) suggest a small-egg strategy at least until a five-chambered stadium. If these early spirulids hatched in a one-chambered stadium, their total shell length would have been 1.1–1.4 mm.
Eocene Beloptera and Miocene Spirulirostra possibly adopted the large IC together with the large-egg strategy from Groenlandibelus. Both genera hatched according to our estimates in a one- to two-chambered stadium; hence, slightly before or slightly after the formation of the first lamello-fibrillar septum. In Beloptera, this assumption fits well, because the secretion of the first septum coincides with the onset of sheath formation. With respect to the onset of increased growth of its guard-like sheath, Spirulirostra alternatively hatched later between the fifth and the sixth chamber at a shell length of 3.5 mm to 4.0 mm.
Our interpretations presented here suggest that the length of the IC is an adequate, but not the ultimate, tool to deduce the length of the ES. When chambers 2–3 are not exceptionally short, ICs longer than 1 mm point to ESs exceeding the 2 mm boundary, whereas ICs distinctly shorter than 1 mm indicate ESs smaller than 2 mm. It is important to note that the IC length is not applicable to sepiids and forms with ICs shorter than 1 mm but comparatively long subsequent chambers (e.g., Beloptera).
Reconstruction of Coleoid Reproductive Strategies through Time
Paleozoic Coleoids
The oldest unambiguous coleoid with an internal shell and a rostrum comes from the lower Carboniferous (Doguzhaeva et al. Reference Doguzhaeva, Mapes, Mutvei, Tanabe, Shigeta, Sasaki and Hirano2010; Mapes et al. Reference Mapes, Doguzhaeva, Mutvei, Landman and Tanabe2010). Hematites barbarae, which is the earliest known (Serpukhovian) coleoid belonging to the short-lived order Hematitida, had an IC of 0.6 mm and comparatively short subsequent chambers. If Hematites hatched with two buoyant chambers—as assumed for belemnoids—this species probably produced small eggs with planktonic hatchlings (Fig. 7). The Fayetteville Shale in Arkansas contains abundant rostra of this species, but most (if not all of them) were preserved in phosphatic coprolites together with remnants of other cephalopods. Absence of benthic organisms and phosphatizing of coproliths demonstrate the existence of dysoxic/anoxic bottom conditions (Mapes et al. Reference Mapes, Doguzhaeva, Mutvei, Landman and Tanabe2010), which in turn allows the supposition that these earliest coleoids had a nektonic lifestyle well above the bottom and reproduced in the pelagic layers like extant Ommastrephidae.

Figure 7. Stratigraphic occurrences, phylogenetic relationships (after Fuchs Reference Fuchs2019: Fig. 2b) and the evolution of the reproductive strategy in coleoid cephalopods.
The late Carboniferous Mutveiconites (Late Pennsylvanian) lived during the icehouse and intensive glaciation episodes in Russia and Texas (Fig. 7). Mutveiconites had an IC of 0.20–0.30 mm, suggesting that it produced small eggs. This is consistent with the entire length of a juvenile, posthatching Mutveiconites mirandus shell (likely grown after hatching) being only 3 mm (Doguzhaeva Reference Doguzhaeva2002). Specimens of M. mirandus were found in deposits of a lagoon-like shallow sea that also contained many well-preserved fragments of terrestrial plants, indicating nearshore burial. There were no signs of postmortem drift of the juvenile shells (Doguzhaeva Reference Doguzhaeva2002), suggesting a nursery (and possibly spawning grounds)—a small-egg strategy somewhat similar to that of modern Loligo. Adolescent (subadult) shells of M. milleri come from deeper inner-shelf deposits (~25–100 m) with well-oxygenated water and moderately well-oxygenated bottom sediments (Doguzhaeva et al. Reference Doguzhaeva, Mapes and Dunca2006), which might reflect an ontogenetic shift from an inshore hatching area to a more offshore growth and foraging zone, as in modern Loliginidae (see Nesis Reference Nesis2003 and references therein).
Mesozoic Belemnoids
The same small-egg strategy as in Paleozoic coleoids (e.g., Hematitida, Donovaniconida) is recorded in their descendants inhabiting the Triassic seas. The aulacoceratid genera Aulacoceras, Dictyoconites, Metabelemnites, and Atractites (Jeletzky Reference Jeletzky1966; Bandel et al. Reference Bandel, Engeser and Reitner1984; Bandel Reference Bandel1985; Doguzhaeva Reference Doguzhaeva2002; Doguzhaeva et al. Reference Doguzhaeva, Mapes and Dunca2006; Fuchs Reference Fuchs2012) had embryos with IC ~0.3–0.6 mm, suggesting small ESs and thus small eggs (Fig. 7). The Dictyoconites (IC ~0.5 mm) of the Middle Triassic Cassian Formation (Bandel Reference Bandel1985) inhabited “fully marine conditions” above sea-basin clays (Hausmann and Nützel Reference Hausmann and Nützel2014; Nützel and Kaim Reference Nützel and Kaim2014). The Early Triassic genus Metabelemnites (IC ~0.5 mm; Jeletzky Reference Jeletzky1966) was recovered from the Pardonet Formation, a major carbonate reservoir facies at the continental margin of Pangaea (Davies Reference Davies1997). Possibly aulacoceratids changed from their original shallow-water habitats and during their evolution moved into deeper offshore waters, which is understandable, considering the absence of a muscular mantle in these coleoids (Fuchs et al. Reference Fuchs, Iba, Tischlinger, Keupp and Klug2016). Triassic seas were, for the first time in Earth's history, characterized by an extreme diversity of pelagic reptile predators, such as sauropterygians, ichthyosauromorphs, and thalattosuchian crocodylomorphs (Stubbs and Benton Reference Stubbs and Benton2016), just as modern shelves are inhabited by dolphins and seals. This predation pressure might have made survival in these habitats difficult for semi-planktonic coleoids. Absence of strong mantle musculature also indicates that aulacoceratids (like Paleozoic coleoids) did not migrate far for spawning grounds, like modern Loliginidae and Ommastrephidae, and probably reproduced where they lived: in deeper offshore waters (see Nesis Reference Nesis2003 and references therein).
In contrast to aulacoceratids, their Jurassic and Cretaceous descendants (Phragmoteuthida, Belemnitida, and Diplobelida) lost their tubular body chamber, and the free part of the mantle developed into a hypertrophied muscular pump. This locomotory reorganization enabled a powerful jet propulsion and therefore made proostracum-bearing belemnoids less vulnerable to marine predators. More effective swimming abilities also allowed them to migrate, thus providing an opportunity to separate their range into foraging and reproductive areas with relatively large distances in between. The reproductive strategy of these advanced coleoids remained unchanged: all known representatives produced small eggs (Fig. 7). Many studied Jurassic–Cretaceous representatives of the belemnite genera Acrocoelites, Aulacoteuthis, Dactyloteuthis, Dicoelites, Hibolithes, Holcobelus, Lissajousibelus, Pachybelemnopsis, Pachyteuthis, Parapassaloteuthis, Eocylindroteuthis, Somalibelus, Belemnopsis, and Cylindroteuthis were characterized by a maximum size of IC of ~0.3–0.6 mm (Pugaczewska Reference Pugaczewska1961; Jeletzky Reference Jeletzky1966; Kabanov Reference Kabanov1967; Barskov Reference Barskov1973; Bandel et al. Reference Bandel, Engeser and Reitner1984; Mariotti and Pignatti Reference Mariotti and Pignatti1990; Doguzhaeva et al. Reference Doguzhaeva, Mutvei and Weitschat2003, Reference Doguzhaeva, Weis, Delsate and Mariotti2014; Larson Reference Larson2010; Doguzhaeva and Bengtson Reference Doguzhaeva and Bengtson2011; Arkhipkin et al. Reference Arkhipkin, Weis, Mariotti and Shcherbich2015; Weis et al. Reference Weis, Dzyuba, Mariotti and Chesnier2015; Doguzhaeva and Meléndez Reference Doguzhaeva and Meléndez2017; Ippolitov and Desai Reference Ippolitov and Desai2019), so their eggs were small and hatchlings pelagic, as in the modern epipelagic oceanic squid families Ommastrephidae, Enoploteuthidae, and Thysanoteuthidae (Nigmatullin and Laptikhovsky Reference Nigmatullin and Laptikhovsky1994; Nigmatullin and Arkhipkin Reference Nigmatullin, Arkhipkin and Okutani1998; Laptikhovsky Reference Laptikhovsky1999). Modern deep-sea cephalopods generally produce larger eggs, but deep waters were not available to belemnites due to the risk of shell implosion (see Westermann Reference Westermann1973). The general absence of belemnite records in deep-sea deposits corroborate the idea of belemnites being shelf dwellers (e.g., Doyle Reference Doyle1992), so they did not evolve eggs of even a moderate size. The giant megateuthid belemnite genera Eocylindroteuthis and Megateuthis (Pugaczewska Reference Pugaczewska1961; Iba et al. Reference Iba, Sano and Mutterlose2014) had ICs of 0.70–0.77 mm, which indicates a very weak tendency toward egg size increase along with the size of females, which is also common in modern squids. Jeletzky (Reference Jeletzky1966) and Košt’ák and Wiese (Reference Košťák and Wiese2008) reported relatively large ICs (1–1.2 mm) of cold-water polar species like Oxyteuthis cf. pugio (from the Canadian Arctic) (Jeletzky Reference Jeletzky1966) and Praeactinocamax aff. plenus from (Taimyr, northwest Siberia) (Košt’ák and Wiese Reference Košťák and Wiese2008) expectably produced relatively large eggs (IC = 1–1.2 mm). The observation of increased IC sizes (and thus larger eggs) in high-boreal belemnites is not surprising, as the inverse relationship between egg size in marine animals and water temperature is a common phenomenon in marine animals known as the Thorson-Rass rule (e.g., as in most modern cold-water loliginids, such as D. gahi and Loligo forbesi; see Laptikhovsky Reference Laptikhovsky2006 and references therein).
The Diplobelida, a group of belemnoid coleoids with a very slender proostracum, have been considered as stem decabrachians (Fuchs et al. Reference Fuchs, Keupp and Wiese2012b). Initial chambers of the rostrum-less diplobelids Conoteuthis and Tauriconites fall in the same size category as belemnites (Drushchits et al. Reference Drushchits, Kabanov and Nerodenko1984; Fuchs et al. Reference Fuchs, Keupp and Wiese2012b).
Spirulida and Sepiida (Late Cretaceous to Recent)
Late Cretaceous groenlandibelids (Groenlandibelus, Cyrtobelus) have variously been considered as stem decabrachians, stem spirulids, or the root of a clade including spirulids and oegopsids (Strugnell et al. Reference Strugnell, Hall, Vecchione, Fuchs and Allcock2017). Their IC size varies between 0.4 and 1.5 mm and the egg sizes probably varied widely between species. For the first time in the evolutionary history of coleoids, some of them could exhibit a large-egg reproductive strategy, such as the Maastrichtian G. rosenkrantzi with an IC of 1.5 mm and an estimated ES of 3–4.3 mm suggests (Fig. 7).
Cenozoic spirulids (Eocene Belopteridae, Miocene Spirulirostridae) with IC sizes ranging from 0.9 mm to 1.7 mm and estimated ES sizes of strikingly more than 2 mm (see Table 2) apparently maintained the large-egg strategy, though some enigmatic forms (Eocene Vasseuria) likely produced small eggs. Cenozoic spirulids had an epicontinental, demersal lifestyle such as is found in contemporary and modern sepiids (Young et al. Reference Young, Vecchione and Donovan1998), so it is not surprising that some of them produced large eggs and switched to a large-egg reproductive strategy.
The first sepiids (Maastrichtian Ceratisepia vanknippenbergi) had a bowl-shaped IC with a length of just 0.08 mm and a maximum diameter of 0.35 mm, making them among the smallest of all cephalopods. The shell of C. vanknippenbergi was represented by a single post hatchling and was of 3.64 mm in length (Hewitt and Jagt Reference Hewitt and Jagt1999), so the egg size of this species was no more than 3.6 mm, possibly much less, as the shell already bore 14 septal growth increments (Hewitt and Jagt Reference Hewitt and Jagt1999). We suppose that this archaic cuttlefish laid small eggs of 1–1.5 mm, as its ancestors did. Even if this species hatched with seven to eight septa like modern S. officinalis (not three to five as assumed for extinct cuttlefishes), it probably still produced small eggs of <2 mm.
The ICs of Paleocene Aegyptosepia lugeri (Košt’ák et al. Reference Košťák, Jagt, Speijer, Stassen and Steurbaut2013) and Eocene belosaepiids (Belosaepia sepioidea, Belosaepia ungula) are much larger, ~1–1.5 mm in diameter. They probably laid eggs 3–8 mm long, which is consistent with the large-egg strategy observed in modern cuttlefishes.
Conclusions
In total, existing data provide evidence that neither Paleozoic nor Mesozoic coleoids exhibited anything other than the small-egg planktotrophic strategy inherited from their bactritoid ancestors. In the Triassic and Jurassic most of them lived and reproduced in shallow seas that occupied vast areas of continental platforms due to the hot climate and the absence of polar ice caps. Based on this ancestral strategy and ES morphology, it is suggested that in the Early–Middle Jurassic, Belemnitida colonized a broad range of depths along the coastal shelves, expanded their geographic distribution, and increased in diversity. The demise of the remaining belemnoids (Diplobelida and Belemnitida) began at the very end of the Cretaceous, at least partly due to competition with modern octo- and decabrachians (Iba et al. Reference Iba, Mutterlose, Tanabe, Sano, Misaki and Kazunobu2011). The large-egg strategy appeared first in crown decabrachians, at the end of the Cretaceous—at least in Groenlandibelus and possibly in cirrate octopods that are known since the Santonian (Tanabe et al. Reference Tanabe, Trask, Ross and Hikida2008). Similar larger eggs might have also occurred in some Maastrichtian cuttlefishes, though the only known species, C. vanknippenbergi, still exhibited a small-egg strategy. On the other hand, cuttlefishes possibly always laid egg masses on the bottom regardless of the egg size and had nekto-benthic hatchlings that might have avoided mass mortality from the catastrophic events in the surface waters following the K-T impact.
Unfortunately, we have no information about ICs of gladius-bearing octobrachians, which appeared during the Triassic and were common during the Jurassic and Cretaceous until their decline before the K/T boundary. However, even in octopus-like forms like Proteroctopus and Palaeoctopus, the body form included well-developed fins and was reminiscent of modern oceanic squids of the families Onychoteuthidae and Enoploteuthidae, and so is consistent with a pelagic nektonic lifestyle (e.g., Fischer and Riou Reference Fischer and Riou2002; Fuchs et al. Reference Fuchs, Bracchi and Weis2009). Squid-like forms (e.g., Plesioteuthis and Palaeololigo) by their body morphology are reminiscent of modern nektonic shelf-oceanic Ommastrephidae or shelf Loliginidae, respectively. As neither modern pelagic nektonic squids nor epipelagic planktonic octopods exhibit an unambiguous large-egg strategy, production of large eggs by Mesozoic representatives of this group is highly unlikely.
This large-egg strategy helped large-egged coleoids to survive the K-T extinction, as Nautilida did versus Ammonoidea, and to prosper in the Cenozoic seas (Laptikhovsky et al. Reference Laptikhovsky, Rogov, Nikolaeva and Arkhipkin2013). Subsequently, the alternative small-egg strategy might have evolved among diversifying crown octo- and decabrachians quickly and opportunistically in response to environmental pressure preceding important morphological body changes, as exemplified by the sibling octopod species O. bimaculatus (eggs 2.5–4.0 mm) and Octopus bimaculoides (eggs 12–17 mm) (Pickford Reference Pickford1949) with very different egg size but very similar body morphology.
Summary
• An unambiguous, universal hatching marker as in ectocochleates does not exist in endocochleate coleoids. Septal spacing may leave a hatching signal in some extant and extinct sepiids, but this feature is not observable in other fossil groups. It is possibly no coincidence that the secretion of the first lamello-fibrillar septum, which respectively corresponds to a one- and a two-chambered shell in non-belemnoids and belemnoids, represents the only key growth stage that invariably occurs in phragmocone-bearing coleoids.
• According to our shell length estimates (Fig. 6), belemnoid shells reached the 2 mm milestone (boundary between small- and large-egg strategy) with a minimum of three to four chambers (two to three buoyant chambers). While the shells of Late Cretaceous Cyrtobelus are similar in size to belemnoids, most studied Cenozoic spirulids and sepiids possess larger ICs and therefore reach the 2 mm boundary earlier with two to three chambers (one buoyant chamber).
• The current phylogeny of crown coleoids (e.g., Strugnell et al. Reference Strugnell, Hall, Vecchione, Fuchs and Allcock2017; Tanner et al. Reference Tanner, Fuchs, Winkelmann, Gilbert, Pankey, Ribeiro, Kocot, Halanych, Oakley, da Fonseca, Pisani and Vinther2017) suggests that the small-egg strategy is the plesiomorphic reproductive strategy. Therefore, the shift to the large-egg strategy occurred convergently in the Octobrachia and Decabrachia (Fig. 7). To better resolve the current picture in the decabrachian lineage, measurements of more Late Cretaceous ICs are necessary (e.g., Longibelus).
• The earliest coleoids had a nektonic lifestyle, living well above the bottom, and spawned small eggs in the pelagic layers. During the Mesozoic diversification of coleoids (Aulacoceratida, Phragmoteuthida, Belemnitida, Diplobelida), none of the known species belonging to these taxa evolved the large-egg strategy. The large-egg strategy in decabrachian cephalopods is first recorded at the end of Cretaceous period and among crown Sepiida and Spirulida during the Eocene.
Acknowledgments
S.N.’s study was supported by the Program of the Presidium of the Russian Academy of Sciences’ Origin of the Biosphere and Evolution of Geo-Biological Systems project Geobiological Events in the Evolution of the Biota on the Example of Cephalopods and Radiolarians and by a subsidy from the Russian government to support the Program of Competitive Growth of Kazan Federal University among the World's Leading Academic Centers. A.I. and M.R. were supported by a grant from the Russian Foundation for Basic Research (RFBR 18-05-01070). We thank Max Barclay (Natural History Museum, London, UK) for checking the English. We finally thank the two referees for their efforts. They helped to enormously improve the quality of the article.