Introduction
The Ediacaran Period (635–541 Ma) represents a critical interval in the evolution of complex life, bridging the microscopic eukaryotic groups of the Cryogenian (Bosak et al. Reference Bosak, Lahr, Pruss, Macdonald, Gooday, Dalton and Matys2012; Riedman et al. Reference Riedman, Porter, Halverson, Hurtgen and Junium2014) to the eventual radiation of macroscopic animal life that epitomizes the Cambrian explosion (Erwin et al. Reference Erwin, Laflamme, Tweedt, Sperling, Pisani and Peterson2011). Cambrian Lagerstätten such as Chengjiang and the Burgess Shale are pivotal to our understanding of the diversification of phylum-level animal clades, yet they tell us comparatively little about perplexing stem- and crown-group metazoan origins, which sit protractedly in the late Neoproterozoic (Peterson et al. Reference Peterson, Lyons, Nowak, Takacs, Wargo and McPeek2004, Reference Peterson, Cotton, Gehling and Pisani2008; Erwin et al. Reference Erwin, Laflamme, Tweedt, Sperling, Pisani and Peterson2011). Furthermore, Ediacaran-aged fossil assemblages represent the earliest evolution of complex ecological interactions such as bioturbation and predation, often hypothesized to be the requisite biological drivers for the Cambrian explosion of morphological innovation (Stanley Reference Stanley1976; Bengston and Zhao Reference Bengston and Zhao1992; Peterson and Butterfield Reference Peterson and Butterfield2005; Sperling et al. Reference Sperling, Frieder, Raman, Girguis, Levin and Knoll2013).
To address these questions, heightened interest has focused on the intermediary Ediacaran fossil record, dominated by a globally distributed marine assemblage of multicellular organisms colloquially referred to as the “Ediacara biota” (Narbonne Reference Narbonne2005). These macrofossils, restricted in this study to lineages of large, soft-bodied organisms predominantly preserved as casts and molds of Ediacaran age (Laflamme et al. Reference Laflamme, Darroch, Tweedt, Peterson and Erwin2013), first appear after the Gaskiers glaciation (579 Ma; Van Kranendonk et al. Reference Van Kranendonk, Gehling and Shields2008) and remain globally distributed until their abrupt disappearance at the Proterozoic–Cambrian boundary (~541 Ma; Narbonne et al. Reference Narbonne, Saylor and Grotzinger1997; but see Jensen et al. Reference Jensen, Gehling and Droser1998; Hagadorn et al. Reference Hagadorn, Fedo and Waggoner2000). Although the phylogenetic affinities of many of these taxa are contentious (e.g., Glaessner Reference Glaessner1984; Gehling Reference Gehling1991; Seilacher, Reference Seilacher1992; Seilacher et al. Reference Seilacher, Grazhdankin and Legouta2003; Budd and Jensen Reference Budd and Jensen2015), emerging paleontological studies suggest they represent an amalgam of stem- and crown-group metazoans in addition to disparate, higher-order eukaryotic clades with no modern representatives (Xiao and Laflamme Reference Xiao and Laflamme2009; Brasier et al. Reference Brasier, Antcliffe and Liu2012; Laflamme et al. Reference Laflamme, Darroch, Tweedt, Peterson and Erwin2013; Rahman et al. Reference Rahman, Darroch, Racicot and Laflamme2015). Recent molecular clock analyses of early animal divergence estimate the origins of demosponge, cnidarian, and bilaterian crown groups deep within the Cryogenian (Peterson et al. Reference Peterson, Cotton, Gehling and Pisani2008; Erwin et al. Reference Erwin, Laflamme, Tweedt, Sperling, Pisani and Peterson2011), suggesting that the Ediacara biota, irrespective of their uncertain metazoan affinities, were contemporaneous with early metazoans (Fedonkin et al. Reference Fedonkin, Simonetta and Ivantsov2007b) and their associated innovations of skeletonization, predation, and bioturbation (Grotzinger et al. Reference Grotzinger, Watters and Knoll2000; Hua et al. Reference Hua, Pratt and Zhang2003; Liu et al. Reference Liu, McIlroy and Brasier2010; Carbone and Narbonne Reference Carbone and Narbonne2014; Darroch et al. Reference Darroch, Sperling, Boag, Racicot, Mason, Morgan, Tweedt, Myrow, Johnston, Erwin and Laflamme2015). As such, their inclusion within a holistic macroevolutionary and ecological framework is critical to study the development of early animal life.
The challenges of conducting evolutionary studies and identifying associated selective pressures (e.g., ecological, evolutionary, environmental, and subsequent physiological changes) on Ediacaran organisms that lack systematic agreement have long been recognized. In light of this problem, Waggoner (Reference Waggoner1999, Reference Waggoner2003) resolved to test hypotheses that sought to explain the distribution of Ediacaran macrofossils in stratigraphic space and time as a function of biogeographic, ecological, and paleoenvironmental factors. Using a modified cladogram to perform parsimony analysis of endemism (wherein localities replaced taxa and presence/absence of taxa replaced characters), three statistically distinct biotic “assemblages,” termed the Avalon, White Sea, and Nama, occurring in loosely ascending stratigraphic order, were identified. The Avalonian assemblage (579–559 Ma) includes the oldest Ediacaran communities, consisting of Rangeomorph and Arboreomorph taxa in deep-water marginal slope and basinal facies (Darroch et al. Reference Darroch, Laflamme and Clapham2013; Liu et al. Reference Liu, Kenchington and Mitchell2015). These assemblages are often preserved under ash beds in “Conception style” preservation (Narbonne Reference Narbonne2005; Kenchington and Wilby Reference Kenchington and Wilby2014) or on the soles of or intrastratally within contourites and turbidites in deep-water sandstone packages (Narbonne et al. Reference Narbonne, Laflamme, Greentree and Trusler2009, Reference Narbonne, Laflamme, Trusler, Dalrymple and Greentree2014). The White Sea biota (558–550 Ma) represents a diverse grouping dominated by Bilateralomorph, Dickinsoniomorph, and Kimberellomorph taxa typically preserved in shallower prodeltaic shelf settings with pervasive microbial mats, creating “Flinders style” cast-and-mold preservation (Martin et al. Reference Martin, Grazhdankin, Bowring, Evans, Fedonkin and Kirschvink2000; Narbonne Reference Narbonne2005; Gehling and Droser Reference Gehling and Droser2013; Zakrevskaya Reference Zakrevskaya2014). The youngest assemblage, the Nama (549–541 Ma), largely consists of depauperate communities of Erniettomorph and Rangeomorph taxa in shallow shelf–shoreface settings, often preserved as three-dimensional molds preserved within beds of storm-deposited sand and channel-fill deposits (Narbonne et al. Reference Narbonne, Saylor and Grotzinger1997; Narbonne Reference Narbonne2005; Vickers-Rich et al. Reference Vickers-Rich, Ivantsov, Trusler, Narbonne, Hall, Wilson, Greentree, Fedonkin, Elliot, Hoffmann and Schneider2013; Ivantsov et al. Reference Ivantsov, Narbonne, Trusler, Greentree and Vickers-Rich2015; Darroch et al. Reference Darroch, Sperling, Boag, Racicot, Mason, Morgan, Tweedt, Myrow, Johnston, Erwin and Laflamme2015). The Nama assemblage is also unique in hosting the earliest skeletonizing macrofauna, including the globally distributed Cloudina (Warren et al. Reference Warren, Fairchild, Gaucher, Boggianai, Poiré, Anelli and Inchausti2011), in addition to unresolved tubular taxa (e.g., Carbone et al. Reference Carbone, Narbonne, Macdonald and Boag2015).
Tectonic (paleogeography), paleoenvironment (lithology and bathymetry), and temporal (evolutionary succession) factors were identified by Waggoner (Reference Waggoner2003) as underlying controls on the known Ediacaran macrofossil record. However, due to a sparse data set (21 localities, 70 genera) and limited geochronological data, the relative impacts of these factors could not be quantitatively defined. In the subsequent decades, dedicated paleontological work has aimed to identify the controlling factors of these assemblages at an outcrop scale. Recent studies suggest these core assemblages may represent discrete temporal intervals (i.e., evolutionary stages; Xiao and Laflamme Reference Xiao and Laflamme2009; Grazhdankin Reference Grazhdankin2014), environmental partitioning (i.e., an ecological response to bathymetry; Grazhdankin Reference Grazhdankin2004; Gehling and Droser Reference Gehling and Droser2013), taphonomic mega-biases (Narbonne Reference Narbonne2005), or a superposition of both time and ecology (Narbonne et al. Reference Narbonne, Laflamme, Trusler, Dalrymple and Greentree2014). It is important to note that despite the hypothesized impact of these variables on Ediacaran taxonomic diversity, they have so far been demonstrated to share little correlation with overall patterns in morphospace among the Ediacara-type biota (Shen et al. Reference Shen, Dong, Xiao and Kowalewski2008).
Here, we present findings from a new, global-scale meta-analysis of the Ediacaran macrofossil record. Using an updated paleontological database that serves as a platform for robust statistical evaluation, we test: (1) the validity of contemporary Ediacaran biotic assemblages as discrete taxonomic groupings; and, (2) the statistical significance of temporal, paleoenvironmental, and lithological factors that may control the observed distribution of taxa in the Ediacaran fossil record at a global scale. Testing the extent to which the Avalon, White Sea, and Nama assemblages represent successive stages in Ediacaran evolution, or instead environmental or taphonomic heterogeneities, is crucial for the development of a robust biostratigraphy for the Neoproterozoic era. Furthermore, a revised biostratigraphy is critical to permit temporally accurate correlative testing of causal drivers for early metazoan diversification—including ecological (Laflamme et al. Reference Laflamme, Darroch, Tweedt, Peterson and Erwin2013; Darroch et al. Reference Darroch, Sperling, Boag, Racicot, Mason, Morgan, Tweedt, Myrow, Johnston, Erwin and Laflamme2015), developmental (Erwin et al. Reference Erwin, Laflamme, Tweedt, Sperling, Pisani and Peterson2011), and environmental (Sperling et al. Reference Sperling, Frieder, Raman, Girguis, Levin and Knoll2013; Lyons et al. Reference Lyons, Reinhard and Planavsky2014) changes under way in the latest Ediacaran stratigraphy worldwide.
Methods
Paleontological Database Parameters
An Ediacaran paleontological database was constructed as a data matrix with localities being defined on the basis of geographic location (i.e., unique latitude and longitude coordinates). When multiple fossil horizons co-occurred in a single continuous stratigraphic package, additional localities were subdivided based on stratigraphic distribution. Localities were coded for the following “characters” using published primary literature: modern locality coordinates, geological unit of occurrence, geochronological age constraint(s), depositional environment and approximate water depth, fossil-preserving lithology, bedding-plane sedimentary structures, diagenetic minerals and processes associated with preservation, taxonomy (and abundance when present—to generic and/or species level when available), associated bioturbation index and ichnotaxa, and finally, historical literature published on the locality of interest.
From this parent database, we restricted the study to localities with more than one taxonomic occurrence, resulting in a subsidiary binary data matrix of 86 Ediacaran localities containing macrofossils (denoted by locality codes in [brackets] hereafter—see Table 1). Importantly, we follow the interpretation that “holdfasts” or “disks” represent the anchoring structure of a frondose organism. As these cannot yet be correlated with any one specific taxon (Burzynski and Narbonne Reference Burzynski and Narbonne2015; Tarhan et al. Reference Tarhan, Droser, Gehling and Dzaugis2015), we did not include Aspidella Billings, Reference Billings1872, and its associated junior synonyms (see Gehling et al. Reference Gehling, Narbonne and Anderson2000; Supplementary Table S.1). In addition, we did not include the taxonomically invalid ivesheadiomorphs Pseudovendia Boynton and Ford, Reference Boynton and Ford1979, Blackbrookia and Shepshedia Boynton and Ford, Reference Boynton and Ford1995, and Ivesheadia Boynton and Ford, Reference Boynton and Ford1996, as they represent either microbially induced sedimentary structures (MISS) (Laflamme et al. Reference Laflamme, Schiffbauer, Narbonne and Briggs2011b) or taphomorphs of frondose organisms in various states of decomposition prior to preservation (Liu et al. Reference Liu, McIlroy, Antcliffe and Brasier2011). Each locality was then coded for the presence or absence of 124 remaining Ediacara-type biota, macroalgae, and tubular and mineralizing genera. This diversity catalogue was then investigated with the following treatments taken from the database to be tested against this taxonomic distribution to evaluate their validity: (1) temporal binning into the three informally assigned Ediacaran biostratigraphic stages (Avalon, 579–559 Ma; White Sea, 558–550 Ma; and Nama, 549–541 Ma; Narbonne et al. Reference Narbonne, Xiao and Shields2012), (2) paleoenvironmental setting and water depth, and (3) preserving facies (lithology), to visualize trends observed in previous studies at a global scale (Supplementary Tables S.2, S.6, and database references therein; Waggoner Reference Waggoner2003; Grazhdankin Reference Grazhdankin2004, Reference Grazhdankin2014; Narbonne Reference Narbonne2005; Gehling and Droser Reference Gehling and Droser2013).
Table 1 List of Ediacaran locality codes used in NMDS analyses.

Data Analysis
We used nonmetric multidimensional scaling (NMDS) to ordinate the 86 Ediacaran localities in multidimensional space based on taxonomic similarity (Jaccard distance). NMDS was chosen over other computational techniques that rely on a Euclidean (linear) relationship between variables and taxonomic composition (such as principal components analysis) as it: (1) uses rank orders, which can accommodate a variety of nonnumerical data types (e.g., presence/absence of taxa and qualitative geological properties used in this study), and (2) is widely accepted as a standard statistical metric within contemporary ecological and community analysis studies (Clapham Reference Clapham2011, and references therein). NMDS collapses multidimensional ranked data into two-dimensional scatter plots (axes NMDS1 and NMDS2), which allows for visualization and interpretation of trends within large data sets. The calculated stress for this original taxonomic ordination was low (0.071), indicating that the resulting biplot provides an excellent representation of rank orders in reduced dimensions (Clarke Reference Clarke1993). Once a taxonomic ordination using NMDS was generated, we then overlaid convex hulls (polygons) representing sites corresponding to the original three assemblages identified by Waggoner (Reference Waggoner1999, Reference Waggoner2003). We then created a second set of polygons linking sites with similar temporal (i.e., time bin), paleoenvironmental (depositional environment and water depth), and lithological characteristics. The degree to which these polygons overlap provides a visual indication of the extent to which the Waggoner assemblages are controlled by time, paleoenvironment, and lithology. We evaluated the statistical validity of these polygons using two methods. First, function “ordiellipse” draws 95% confidence intervals (CIs) around class centroids as ellipses. If the ellipses do not overlap, they are outside of the assigned level of confidence, and the polygons are therefore considered significantly different (Supplementary Table S.4). Second, we quantified the dissimilarity between polygons in partitioned (i.e., “assemblage,” “lithology,” “time,” etc.) data sets using three beta-diversity metrics: (1) mean Jaccard dissimilarity of all pairwise comparisons between categories, (2) multisite Sorenson’s dissimilarity, and (3) multisite Simpson’s dissimilarity metric using package betapart (Table 2; Baselga and Orme, Reference Baselga and Orme2012). Jaccard dissimilarity is a well-known ecological metric that has frequently been used in paleocological studies to quantify taxonomic dissimilarity (e.g., Hammer and Harper Reference Hammer and Harper2006), while Simpson’s beta represents turnover independent of nestedness (Baselga Reference Baselga2010) and is robust to gradients in richness (Koleff et al. Reference Koleff, Gaston and Lennon2003; Darroch and Wagner Reference Darroch and Wagner2015). All analyses were performed using the open-source statistical software package R, package Vegan, Version 2.3-0 (Oksanen et al. Reference Oksanen, Blanchet, Kindt, Legendre, Minchin, O’Hara, Simpson, Solymos, Henry, Stevens and Wagner2015; R Development Core Team 2015).
Table 2 Convex hulls treated as single “community,” in which presence/absence of each genus is summed from all fossil occurrences into each category. Beta diversity is calculated for overall dissimilarity (0=complete similarity, 1=complete dissimilarity) between categories, using Jaccard pairwise dissimilarity, and Simpson and Sorenson multisite indices.

Retesting the Assemblage Concept
A principal goal of this study was to reassess the validity of three Ediacaran assemblages based on taxonomic distinctness as identified in Waggoner (Reference Waggoner2003). We therefore selected the original 21 localities (and respective assemblage designations) identified in Waggoner (Reference Waggoner2003) and applied these as polygons over the updated taxonomic ordination space. The results show that even after a significantly updated data set (86 localities, 124 genera), the original 21 localities and their resulting three assemblages still provide clear taxonomic separation at CI=95% (Fig. 1, Supplementary Table S.4.A). Furthermore, assemblage polygons produced the greatest dissimilarities in beta-diversity values among all tested variables (Table 2), demonstrating strong separation between assemblages in terms of faunal composition.

Figure 1 Ediacaran taxonomic ordination plot based on the updated data set (86 localities, 124 genera). The original 21 localities identified in Waggoner (Reference Waggoner2003) have been color assigned to their respective “Avalon,” “White Sea,” and “Nama” designations. These have then been coded as polygons to assess the degree to which they remain as discrete taxonomic assemblages against an updated ordination space.
These data reflect several important global trends. First, locality-specific diversity among the original localities demonstrates robust assemblage integrity, as both new taxa and localities have been added in the past ~15 years. New localities such as those from Bonavista Peninsula [BV_...] and Spaniard’s Bay [SB_…], Newfoundland, and Charnwood Forest [Brad] and [NQ_...], UK (Hofmann et al. Reference Hofmann, O’Brien and King2008; Narbonne et al. Reference Narbonne, Laflamme, Greentree and Trusler2009; Wilby et al. Reference Wilby, Carney and Howe2011; Liu et al. Reference Liu, Matthews, Menon, McIlroy and Brasier2014) display a significant degree of faunal exchange with traditional Avalonian localities and cluster in close proximity to the classic surfaces near Mistaken Point, Newfoundland [Shin], [LMP], [D_sur], [E_sur], [G_sur], [BC], [PC], [DC] (Clapham et al. Reference Clapham, Narbonne and Gehling2003), as well as the recently updated Sekwi Brook “June beds” from the Mackenzie Mountains, northwest Canada [SJb_Nor2] (Narbonne et al. Reference Narbonne, Laflamme, Trusler, Dalrymple and Greentree2014). The White Sea assemblage shows similar intra-assemblage separation, with classic localities from Nilpena, South Australia [Nil_...] (Gehling and Droser Reference Gehling and Droser2013), Arkhangelsk region, Russia [Zg_...], [Sol_V], [KR], [Suz_...], [Ong], [LR_...] (Martin et al. Reference Martin, Grazhdankin, Bowring, Evans, Fedonkin and Kirschvink2000), Sekwi Brook, northwest Canada [SBf_Nor1] (Carbone et al. Reference Carbone, Narbonne, Macdonald and Boag2015), and Finnmark, Norway [DP_...] (Crimes and McIlroy Reference Crimes and McIlroy1999), forming the vertices of the White Sea polygon. These contain other known White Sea localities such as the Podolia, Ukraine [Mog_PUK] (Sokolov and Fedonkin Reference Sokolov and Fedonkin1990), Olenek Uplift, Siberia [Kh_khat] (Grazhdankin et al. Reference Grazhdankin, Balthasar, Nagovitsin and Kochnev2008), and newly represented localities such as the Himalayan Krol Belt, India [Nain] (Shanker and Mathur Reference Shanker and Mathur1992), and upper Miette Group from Mt. Fitzwilliam in the Canadian Rockies [Fitz] (Hofmann et al. Reference Hofmann, Mountjoy and Teitz1991).
The Nama assemblage encompasses both terminal Ediacaran localities in Namibia, Africa [Nam_...], the southwestern United States [Dea_V3], [Mont_...], North Carolina, U.S.A. [Car_SB], and the Shibantan Member, China [Muz2Sh] (Narbonne et al. Reference Narbonne, Saylor and Grotzinger1997; Hagadorn and Waggoner Reference Hagadorn and Waggoner2000; Weaver et al. Reference Weaver, McMenamin and Tacker2006; Chen et al. Reference Chen, Zhou, Xiao, Wang, Guan, Hua and Yuan2014), in addition to globally distributed occurrences of biomineralizing taxa such as Cloudina in Namibia [Nam_drie], the southwestern United States [Dea_V3], [Mont_…], the Canadian Rockies [Sali1], Oman [Omn], Brazil [MatoGDS], China [Lijian], and Paraguay [Loc…] (Grotzinger et al. Reference Grotzinger, Watters and Knoll2000; Hagadorn and Waggoner Reference Hagadorn and Waggoner2000; Hofmann and Mountjoy Reference Hofmann and Mountjoy2001; Amthor et al. Reference Amthor, Grotzinger, Schröder, Bowring, Ramezani, Martin and Matter2003; Hua et al. Reference Hua, Pratt and Zhang2003; Babcock et al. Reference Babcock, Grunow, Sadowski and Leslie2005; Warren et al. Reference Warren, Fairchild, Gaucher, Boggianai, Poiré, Anelli and Inchausti2011).
Despite the observed taxonomic integrity of Waggoner’s (Reference Waggoner2003) assemblages, there are several isolated Ediacaran macrofossil localities that do not fit into these statistically defined assemblages. For example, macroscopic algal communities preserved as carbonaceous compressions in black shales (“Miaohe” [Miah1] and “Wenghui” [Jiang] biotas: Hubei [Xiao et al. Reference Xiao, Yuan, Steiner and Knoll2002] and Guizhou provinces [Zhao et al. Reference Zhao, Chen, Peng, Yu, He, Wang, Yang, Wang and Zhang2004]) do not place within any of our traditional Ediacaran biotic assemblages (Fig. 1). We do not interpret this lack of taxonomic overlap as merely a taphonomic bias due to nonmoldic preservation. Macroscopic annulated tubular taxa such as Gaojiashania Chen et al., Reference Chen, Sun and Hua2002 and Shaanxilithes Chen et al. Reference Chen, Chen and Lao1975; Xing et al. Reference Xing, Ding, Luo, He and Wang1984, are also preserved as carbonaceous compressions found in the Gaojiashan Member, Dengying Formation, Shaanxi Province [Gaoj] (Cai et al. Reference Cai, Schiffbauer, Hua and Xiao2012). These localities display a similarly disparate relationship as macroscopic algal communities when compared with the traditional Ediacaran biotic assemblages. When observed globally, tubular taxa appear to heavily skew a locality’s distribution away from traditional assemblages, even if they co-occur with known Ediacara biota. This is the case with recent finds in the Zaris Subbasin, Namibia [Nam_ZR] (Shaanxilithes; S. A. F. Darroch, T. H. Boag, R. A. Racicot, S. Tweedt, S. J. Mason, D. H. Erwin, and M. Laflamme, unpublished data), and to a lesser extent the Blueflower Formation of northwest Canada [SBf_Nor1] (Sekwitubulus annulatus, Annulatubus flexuosus, Carbone et al. Reference Carbone, Narbonne, Macdonald and Boag2015). It is therefore clear that both annulated tubular taxa and macroscopic algal communities currently display highly dissimilar taxonomic association to the classic Ediacara-type assemblages as identified by Waggoner (Reference Waggoner2003).
As an alternative method for assemblage visualization, we also performed a hierarchical cluster analysis of our updated data as a second test of assemblage validity. Average-linkage clustering (Kulczynski similarity) using the function hclust was performed on the diversity matrix in the R package Vegan (Oksanen 2015), resulting in a cluster dendrogram that provided good agreement with our NMDS ordination plot. Cophenetic correlation for the dendrogram, which measures the similarity between original locality diversity dissimilarity and dissimilarities estimated by the dendrogram tree, was high (0.8418), indicating this analysis is a reliable alternative method for interpretation of faunal assemblages. Only five localities ([Nil_mf], [Fitz], [SBf_Nor1], [SecA_CC], [Ferry]) changed assemblage designation between the NMDS method and cluster analyses (Fig. 2; see Supplementary Fig. S.3 for alternative clustering method).

Figure 2 Average-linkage hierarchical cluster dendrogram (Kulczynski similarity) of 86 Ediacaran localities generated from the binary faunal catalogue. Assemblage rectangles (Nama, Avalon, Algae, White Sea) were plotted using function rect.hclust, which cuts the dendrogram into n closest clusters.
Our updated taxonomic ordination and cluster analysis supports the original conclusion that the localities set out in Waggoner (Reference Waggoner2003) provide fairly robust approximations of taxonomic diversity within the current Ediacaran macrofossil record and appear to remain as coherent, distinct faunal associations. However, emerging heterogeneities exist and will likely grow as worker effort increases diversity in novel localities (Supplementary Fig. S.3). As such, these data reinforce previous studies, which have locally identified the nonrandom distribution of Ediacaran taxa (Grazhdankin Reference Grazhdankin2004; Gehling and Droser Reference Gehling and Droser2013). The need to statistically test the underlying factors controlling Ediacaran macrofossil distribution is therefore imperative moving forward, as they are likely to inform as to the underlying mechanisms that produce both traditional and novel biotic assemblages.
Taphonomic Biases—Preserving Lithology
Decoupling Preserving Lithology with Moldic Preservation
Taphonomic biases can influence any quantitative paleontological study; this concern is heightened when dealing with exceptional soft-tissue preservation (Briggs Reference Briggs2003). Several taphonomic windows were open during the Ediacaran Period (Kenchington and Wilby Reference Kenchington and Wilby2014), preserving macrofossils in a range of sedimentological facies and depositional environments, albeit in highly disproportionate regularity. We aimed to resolve whether any large-scale lithological barriers are present in order to (1) assess the impact on the overall fidelity of the taxonomic record and (2) constrain any potential lithological dependencies guiding the known Ediacaran taphonomic windows.
Due to a combination of unique paleoenvironmental conditions, microbially induced moldic “death masks” of soft tissues in siliciclastic sandstone and siltstone dominate the Ediacaran fossil record (Gehling Reference Gehling1999; Gehling et al. Reference Gehling, Droser, Jensen and Runnegar2005). Moldic preservation occurs through a complex interplay between two processes: (1) mold formation initiates as labile tissues decay rapidly and are infilled with sediment; and (2) sulfate-reducing bacteria then exploit this decaying organic material, converting sulfate (SO4 2-) to hydrogen sulfide (H2S), which combines with iron in the sediment to precipitate early diagenetic minerals such as pyrite (FeS2). Ultimately, these molds are believed to be the product of multiple depositional factors at the time of burial, including presence of clay minerals, microbial mat prevalence, and ensuing chemistry of pore waters (Narbonne Reference Narbonne2005; Callow and Brasier Reference Callow and Brasier2009; Darroch et al. Reference Darroch, Laflamme, Schiffbauer and Briggs2012, Kenchington and Wilby Reference Kenchington and Wilby2014). In light of this complexity, we instead tested the degree to which overall preserving lithology affects this taphonomic character in order to inform potential outcrop-scale biases in collection methods.
Database results reinforce a clear ubiquity of moldic preservation across Ediacaran genera; convex hulls for each lithology show limited separation toward any of the traditional assemblages, perhaps with the exception of the Avalonian biota, which are tightly correlated with Conception-style preservation in ash (Fig. 3, Supplementary Fig. S.4.C). This inference is strengthened by the results of beta-diversity analyses, which show that lithology accounts for the second-lowest dissimilarity values among tested variables after temporal binning (Table 2). Although moldic preservation may appear to create a taphonomic filter for epifaunal taxa (Kenchington and Wilby Reference Kenchington and Wilby2014), with the exception of the unique Conception-type, moldic preservational styles are observed in a wide range of paleoenvironments (shallow shoreface to deep-water slope; see Bouougri et al. Reference Bouougri, Porada, Weber and Reitner2011; Narbonne et al. Reference Narbonne, Laflamme, Trusler, Dalrymple and Greentree2014). Furthermore, moldic preservation appears to be largely decoupled from any strictly lithological controls, such as carbonate facies (Grazhdankin et al. Reference Grazhdankin, Balthasar, Nagovitsin and Kochnev2008; Chen et al. Reference Chen, Zhou, Xiao, Wang, Guan, Hua and Yuan2014; Fig. 3, [Kh_khat] and [Muz2Sh]). This can make the identification of any major taxonomic–taphonomic moldic biases beyond in vivo position relative to the sediment–water interface difficult.

Figure 3 Distribution of macrofossil-preserving lithologies at each locality, overlain as polygons across our updated taxonomic ordination space. Siliciclastic coarse-grained sandstone (Coarse sandstn.), fine-grained siltstone (Mixed siltstn.), and limestones (Limestone) appear broadly distributed across taxa, while siliciclastic and carbonate shale/mudstones (Shale/mudstn.) and ash (Ash) show greater taxonomic restriction.
There are several implications of these results. First, Ediacaran taxonomy does not appear to suffer from any litho-preservational barriers among taxa preserved via moldic impressions in siltstone–sandstone grained siliciclastic settings. As discussed, this trend extends into the heavily undersampled carbonate facies (e.g., Chen et al. Reference Chen, Zhou, Xiao, Wang, Guan, Hua and Yuan2014). Second, from a worker effort or “search image” perspective, further explorative work for new Ediacaran localities should remain focused on identifying heterolithic sedimentary packages with sedimentological and structural properties suitable for moldic preservation: abundant MISS, cleavage planes that align with original bedding surfaces, and well-defined bedding soles, which are often formed via contourite and/or turbidity currents (Sperling et al. Reference Sperling, Carbone, Strauss, Johnston, Narbonne and Macdonald2015), and not limited to just siliciclastic sandstone and siltstone lithofacies.
Finally, these data may point to the importance of microbial mats, and not necessarily lithology, in the overall mold-forming process. It is well documented that Ediacaran seafloors were marked by global evidence for extensive microbial mats and absence of deep bioturbation (Mángano and Buatois Reference Mángano and Buatois2014; Davies et al. Reference Davies, Liu, Gibling and Miller2016). These mats would therefore have supported large populations of decay bacteria and created an effective seal over newly buried organisms. This seal would have largely restricted the flux of an oxygenated water column into dysoxic–anoxic pore waters beneath the sediment–water interface, producing geochemical conditions that would heavily favor early diagenetic precipitation of preserving minerals such as pyrite (see above; Darroch et al. Reference Darroch, Laflamme, Schiffbauer and Briggs2012). In addition, scavenging and macrophagy are conspicuously absent until the latest Ediacaran, creating ecosystems with minimal biological biostratinomic destruction (Kenchington and Wilby Reference Kenchington and Wilby2014). These conditions have led several authors to suggest the overall fidelity of Ediacaran soft-tissue preservation was vastly superior to that of the Phanerozoic (Brasier et al. Reference Brasier, Antcliffe and Callow2010). As such, exceptional moldic preservation in the Ediacaran should permit a robust representation of true biological patterns with relatively few taphonomic biases in comparison with Phanerozoic settings. Furthermore, as microbial mats appear ubiquitous in their temporal, geographic, and bathymetric distribution in the Ediacaran prior to large-scale metazoan bioturbation, the spatial/temporal partitioning of mats as a potential taphonomic bias in the Ediacaran appears largely decoupled from the other factors tested in this study (Laflamme et al. Reference Laflamme, Schiffbauer and Narbonne2011a, Reference Laflamme, Darroch, Tweedt, Peterson and Erwin2013).
Despite the pervasiveness of the moldic taphonomic window in the Ediacaran, it is important to note that moldic preservation may exert a bias against epifaunal taxa that extend into the water column (Kenchington and Wilby Reference Kenchington and Wilby2014). Consequently, much of the Ediacaran fossil record is dominated by taxa that are proximal to the sediment–water interface, such as the discoidal taxon Aspidella (Laflamme et al. Reference Laflamme, Schiffbauer, Narbonne and Briggs2011b), regarded to be the anchoring holdfasts for fronds (Gehling et al. Reference Gehling, Narbonne and Anderson2000). Rarely are both holdfast and frond preserved on the same bedding surface (e.g., Laflamme et al. Reference Laflamme, Narbonne and Anderson2004; Narbonne et al. Reference Narbonne, Laflamme, Trusler, Dalrymple and Greentree2014: Fig. 4.4). Rather, fronds appear either without associated holdfast in instances where the disk remained buried within the sediment both in vivo and during preservation (Laflamme et al. Reference Laflamme, Narbonne, Greentree and Anderson2007, Reference Laflamme, Schiffbauer, Narbonne and Briggs2011b), or more commonly, the frond itself will be absent, leaving only bedding surfaces covered in holdfasts in varying styles of preservation (Tarhan et al. Reference Tarhan, Droser and Gehling2010; Kenchington and Wilby Reference Kenchington and Wilby2014). The ubiquity of holdfasts and comparative rarity of preserved fronds is a critical unresolved taphonomic bias present in the current Ediacaran record (Laflamme et al. Reference Laflamme, Schiffbauer, Narbonne and Briggs2011b). As holdfasts cannot be rigorously correlated with specific frondose taxa (Burzynski and Narbonne Reference Burzynski and Narbonne2015), this reinforces that both the diversity and stratigraphic range of frondose taxa are likely highly underrepresented in localities with dense holdfast assemblages (Darroch et al. Reference Darroch, Sperling, Boag, Racicot, Mason, Morgan, Tweedt, Myrow, Johnston, Erwin and Laflamme2015).

Figure 4 Localities that have been geochronologically constrained are binned into Avalon, White Sea, and Nama temporal intervals. These have then been expressed as polygons on top of our taxonomic ordination space to assess the degree to which these suggested biostratigraphic stages represent discrete taxonomic intervals.
Sampling Intensity and Lithology Limit Carbonaceous Compressions
Although the mid-late Ediacaran macrofossil record is dominated by moldic soft-tissue preservation, in several cases carbonaceous compressions have been recovered. The most well-known instances of this occur in several Chinese localities, including the Gaojiashan Lagerstatte, Shaanxi Province (Cai et al. Reference Cai, Schiffbauer, Hua and Xiao2012), and the Miaohe and Wenghui biotas in the Hubei and Guizhou provinces, respectively (Xiao et al. Reference Xiao, Yuan, Steiner and Knoll2002; Zhao et al. Reference Zhao, Chen, Peng, Yu, He, Wang, Yang, Wang and Zhang2004) (Fig. 3: [Gaoj], [Miah1], [Jiang]). Carbonaceous compressions, formed via kerogenization (polymerization of organic molecules), are best known from the iconic Cambrian Burgess Shale; hence they are often referred to as Burgess Shale–type (BST) preservation (Orr Reference Orr2014). Kerogenization is essential to BST preservation; however, it can be accompanied by two other mineralization processes: authigenic pyritization and aluminosilicification, which can co-occur as a range of taphonomic end members that together contribute to the BST taphonomic mode (Cai et al. Reference Cai, Schiffbauer, Hua and Xiao2012; Schiffbauer et al. Reference Schiffbauer, Xiao, Cai, Wallace, Hua, Hunter, Xu, Peng and Kaufman2014).
Database analysis has shown significant undersampling of fossiliferous shale lithofacies, identifying only 6 BST candidates from our 86 Ediacaran localities and >45 geological units: [Ong], [MatoGDS], [Jiang], [Gaoj], [Kh_khat],and [Miah1] (Fig. 3). These deposits all form in low-energy, deep-water distal offshore shelves or restricted basinal lagoons. Perhaps the most uncertain feature of Ediacaran BST deposits is the apparent exclusion of frondose taxa from this preservational window (Steiner and Reitner Reference Steiner and Reitner2001; Grazhdankin et al. Reference Grazhdankin, Balthasar, Nagovitsin and Kochnev2008). The Khatyspyt Formation in Siberia [Kh_khat] is interpreted to be a distal, low-energy carbonate ramp, below storm-weather wave base (SWWB). Although it contains exquisitely preserved Rangeomorphs such as Charnia Ford, Reference Ford1958, occurring as molds in authigenic carbonate cementation, these same frondose taxa are relegated to “phantoms” within interbedding carbonaceous compression windows (Grazhdankin et al. Reference Grazhdankin, Balthasar, Nagovitsin and Kochnev2008: Fig. 3a–h). New Ediacaran localities with BST-type preservation may therefore help to confirm the presence/absence of certain Ediacaran taxa from this mode of preservation, thus resolving issues associated with taphonomic effect versus ecological biofacies (Zhu et al. Reference Zhu, Gehling, Xiao, Zhao and Droser2008; Gehling and Droser Reference Gehling and Droser2013; Kenchington and Wilby Reference Kenchington and Wilby2014; Carbone et al. Reference Carbone, Narbonne, Macdonald and Boag2015).
Temporal Distribution of Ediacara Biota
Chronostratigraphic Overlap of the Avalonian and White Sea Assemblages
Evolutionary succession has often been suggested as an important factor in the distribution of taxa in the Ediacaran macrofossil record. However, the ability to test the biotic assemblages as discrete temporal intervals has been limited by a lack of high-quality geochronology. We use a collection of updated publication data to reanalyze Waggoner’s core assemblage concept as temporally distinct by binning Ediacaran localities into the traditionally accepted biostratigraphic intervals for the Avalon (579–559 Ma), White Sea (558–550 Ma), and Nama (549–541 Ma) (Narbonne et al. Reference Narbonne, Xiao and Shields2012; see Supplementary Table S.5). If the hypothesis that the three assemblages represent a response to a temporal control on the distribution is correct, then temporal binning should also display a clear polygonal separation similar to the initial taxonomic separation (Fig. 1). Instead, NMDS ordination plots of time-binned localities reveal that temporal overlap is present between both the Avalon–White Sea and White Sea–Nama assemblages. These results agree with the current, albeit limited geochronological record (Fig. 4, discussed below), and are supported by beta-diversity (dissimilarity) metrics showing that temporal binning produces the lowest diversity dissimilarity when compared with all other variables being tested (Table 2). However, upon further statistical analyses, the data produce contradictory results. When confidence ellipses were drawn around the class centroids for each time bin, they did not overlap, suggesting the assemblages are in fact temporally distinct at CI=95% (Supplementary Fig. S.4.D). These inconsistent results may reflect differences in the relative sample size of tested variables: abundant diversity data yield higher degrees of confidence and therefore a narrower overall confidence interval, while the limited number of geochronological dates requires significantly wider confidence intervals (see Supplementary Table S.5). At present, both NMDS and beta-diversity data support previous local-scale observations of contemporaneous but unrelated taxa partitioning into different depositional environments (Grazhdankin Reference Grazhdankin2004; Wilby et al. Reference Wilby, Carney and Howe2011; Gehling and Droser Reference Gehling and Droser2013; Noble et al. Reference Noble, Condon, Carney, Wilby, Pharaoh and Ford2014). Meanwhile, confidence testing reinforces the need for more critical age constraints of fossiliferous Ediacaran strata that may bridge traditional assemblage time intervals.
Recently updated geochronology of the Charnian Supergroup of Charnwood Forest, England, has placed much of the classic Avalonian assemblage into a clearer biostratigraphic context. For instance, a date of 561.85±0.34 Ma (Noble et al. Reference Noble, Condon, Carney, Wilby, Pharaoh and Ford2014) now provides a maximum age for the Bradgate Park Formation (Fig. 4: [NQ_D], [NQ_A], [NQ_B]). Additionally, although no dates were recovered from the stratigraphically highest fossiliferous surfaces, which preserve the greatest taxonomic diversity, they lie ~200 m below the Hanging Rocks Formation, which has been dated 556.6±6.4 Ma. This effectively constrains the age of the Avalonian frondose taxa in the Bradgate Park Formation to ca. 561–557 Ma (Noble et al. Reference Noble, Condon, Carney, Wilby, Pharaoh and Ford2014). Taking uncertainty into account, the localities at Bradgate Park appear likely to overlap temporally with the lowermost fossiliferous strata in the White Sea region in Russia. The prodeltaic and outer-shelf succession of the Lyamsta Formation is constrained by a youngest possible age of 558±1 Ma from the overlying Verkhovka Formation (Grazhdankin Reference Grazhdankin2004). It contains a highly disparate population in comparison with deep-water frondose communities at Bradgate Park, including Dickinsonia Wade, Reference Wade1972, Parvancorina Glaessner, Reference Glaessner1958, and sac-like Inaria Gehling, Reference Gehling1988 [LR_pd], [LR_ds] (Grazhdankin Reference Grazhdankin2004). Thus, with current, albeit limited geochronological data, it is apparent that highly diverse, yet taxonomically dissimilar assemblages were likely to have co-occurred at least during the Avalon–White Sea transition. These data lessen the case for evolutionary succession exerting a first-order control on Avalon–White Sea assemblages. However, a lack of precise geochronology highlights the effect that an uneven chronostratigraphic record can have on interpreting trends in both global diversity and evolution. Specifically, data illustrating the relative youth of the uppermost Bradgate Park Formation place heightened emphasis on obtaining similarly accurate age constraints for the Avalon Peninsula Conception Group. While the Conception Group records the oldest occurrence of Ediacaran body fossils (578.8±0.5 Ma; Van Kranendonk et al. Reference Van Kranendonk, Gehling and Shields2008; Schmitz Reference Schmitz2012) from the upper Drook Formation, the stratigraphic range of its Avalonian taxa are still poorly constrained by a maximum age of 565±3 Ma (Benus Reference Benus1988), taken from the upper Mistaken Point Formation. The ~1400 m of overlying fossiliferous strata of the Trepassey and Fermeuse formations on both the Avalon and Bonavista peninsulas of Newfoundland lack any geochronological constraint (Williams and King Reference Williams and King1979; Wood et al. Reference Wood, Dalrymple, Narbonne, Gehling and Clapham2003; Hofmann et al. Reference Hofmann, O’Brien and King2008), and may provide a much younger maximum age for the Avalonian assemblage.
It is also important to evaluate both taphonomic and stratigraphic gaps in the known record when assessing global trends in taxonomic diversity and evolution. Both first and last appearances of specific taxa can be highly concentrated along physical or preservational disconformities that truncate the stratigraphic range of taxa that would otherwise have first appeared or gone extinct in an unrecorded interval of time (Kidwell and Holland Reference Kidwell and Holland2002). A similar effect can be induced by rapid up-section changes in paleoenvironment. These factors are both present in the uppermost stratigraphy of Newfoundland and Charnwood Forest. While fine- to medium-grained deep-water turbiditic facies preserve the fossil communities from the Bradgate Park Formation, the overlying Hanging Rocks Formation is bounded by an erosional surface. It is composed of poorly sorted volcanic epiclastic conglomerates and pyroclastic components, overlain by medium-grained sandstones and tuffaceous siltstones interpreted as turbidity currents carrying reworked detritus from much shallower fluvial or nearshore conditions (McIlroy et al. Reference McIlroy, Brasier and Moseley1998). A similar shift in paleoenvironment occurs within the stratigraphy at Bonavista Peninsula. There, weak turbidity currents with thin interbedded fossil-preserving ash beds of the Fermeuse Formation grade into shallower prodelta and delta-front facies of the Renews Head Formation (Williams and King Reference Williams and King1979; O’Brien and King Reference O’Brien and King2005). These facies shifts may have removed both the environmental conditions for a deep-water Avalonian biotope to exist and the Conception-style taphonomic window that preserves the majority of epifaunal fronds (Hofmann et al. Reference Hofmann, O’Brien and King2008). Factors such as these reinforce the need for caution when interpreting the absence of certain taxa from the current Ediacaran record as a true biological signal (Sperling et al. Reference Sperling, Carbone, Strauss, Johnston, Narbonne and Macdonald2015). Global studies of macroevolutionary patterns must take place within a stratigraphic framework that can statistically account for varying likelihoods of preservation, be it taphonomic, environmental, or a superposition of the two (Holland Reference Holland1995, Reference Holland2003). Given the current uncertainty in correlating the global Ediacaran stratigraphic record, the most rigorous method of assessing global trends in biodiversity should remain dedicated to geochronologically constrained outcrop-scale studies, which can better account for these preservational impacts on observed diversity (Darroch et al. Reference Darroch, Sperling, Boag, Racicot, Mason, Morgan, Tweedt, Myrow, Johnston, Erwin and Laflamme2015).
Assessing Biotic Turnover in the Depauperate Nama
The temporal overlap observed between the White Sea and Nama assemblages is more complex. With the exception of the Khatyspyt Formation in the Olenek Uplift of Siberia (Grazhdankin et al. Reference Grazhdankin, Balthasar, Nagovitsin and Kochnev2008), there is significant taxonomic separation between terminal Ediacaran strata (549–541 Ma) and older White Sea localities (Fig. 4). The deep-water Khatyspyt Formation [Kh_khat] is a unique assemblage in itself, plotting closely with other White Sea localities (Waggoner Reference Waggoner2003; this study), but lacking the iconic Dickinsoniomorph, Bilateralomorph, Kimberellomorph taxa that define the White Sea Region, Russia, and poorly age-constrained 556±24 Ma, Australian assemblages (Preiss Reference Preiss2000). The Khatyspyt consists of the fronds Charnia and Khatyspytia Fedonkin, 1985 (in Fedonkin et al. Reference Fedonkin, Gehling, Grey, Narbonne and Vickers-Rich2007a), numerous Aspidella-type morphs, the serial Palaeopascichnus Palij, Reference Palij1976, and sac-like Inaria preserved in nodular bituminous limestones (Knoll et al. Reference Knoll, Grotzinger, Kaufman and Kolosov1995; Grazhdankin et al Reference Grazhdankin, Balthasar, Nagovitsin and Kochnev2008). Interstratified within these limestones are calcareous mudstones, which preserve an assemblage of carbonaceous compressions of taxa similar to the >551.09±1.02 Ma Miaohe assemblage in the uppermost Doushantuo Formation of south China (Condon et al. Reference Condon, Zhu, Bowring, Wang, Yang and Jin2005; Xiao et al. Reference Xiao, Yuan, Steiner and Knoll2002).
Several authors have referred to the Khatyspyt Formation as “Avalon-type,” based on its relatively deep-water distal carbonate ramp depositional setting, presence of fronds, and absence of typical White Sea biota (Grazhdankin et al. Reference Grazhdankin, Balthasar, Nagovitsin and Kochnev2008; Rogov et al. 2012). Interestingly, our ordination plots do not place the Khatyspyt within the Avalon-type taxonomic designation, and instead agree with the results of Waggoner (Reference Waggoner2003) by placing it squarely within the White Sea assemblage ([Kh_khat] in Figs. 1, 4). From a stratigraphic perspective, a maximum age for the Khatyspyt assemblage is provided by a volcaniclastic breccia unit, which crosscuts the overlying Kessyusa Formation and is dated at 543.9±0.3 Ma (Bowring et al. Reference Bowring, Grotzinger, Isachsen, Knoll, Pelechaty and Kolosov1993; Knoll et al. Reference Knoll, Grotzinger, Kaufman and Kolosov1995). However, the presence of Cambrian shelly tubular taxa Cambrotubulus and Anabarites in the intermediary Turkut Formation (Brasier et al. Reference Brasier, Shields, Kuleshov and Zhegallo1996; Maloof et al. Reference Maloof, Porter, Moore, Dudás, Bowring, Higgins, Fike and Eddy2010) reinforces that the underlying Khatyspyt assemblage remains poorly constrained as an important deep-water end member for the terminal Ediacaran.
Traditional shallower-water Nama-type localities display unusually depauperate populations of taxa and are principally separated into two populations. Limestones and micritic mudstone horizons preserve mineralizing taxa such as Cloudina Pflug, Reference Pflug1972, Namacalathus Grotzinger et al., Reference Grotzinger, Watters and Knoll2000, and Namapoikia Wood et al., Reference Wood, Grotzinger and Dickson2002 [Nam_drie], [Sali_1], [Lijian], [Omn] (Grotzinger et al. Reference Grotzinger, Watters and Knoll2000; Hofmann and Mountjoy Reference Hofmann and Mountjoy2001; Wood et al. Reference Wood, Grotzinger and Dickson2002; Amthor et al. Reference Amthor, Grotzinger, Schröder, Bowring, Ramezani, Martin and Matter2003), often co-occurring with the tubular genus Corumbella Hahn et al., Reference Hahn, Hahn, Leonardos, Pflug and Walde1982 [MatoGDS], [Loc1], [Loc2] (Babcock et al. Reference Babcock, Grunow, Sadowski and Leslie2005; Warren et al. Reference Warren, Fairchild, Gaucher, Boggianai, Poiré, Anelli and Inchausti2011; see Figs. 2, 4). The global distribution of these taxa, coupled with their abundant and consistent preservation in terminal Ediacaran (549–541 Ma) carbonate successions favors this association as an excellent candidate for a globally correlative biozone (Grant Reference Grant1990; Warren et al. Reference Warren, Fairchild, Gaucher, Boggianai, Poiré, Anelli and Inchausti2011). The few soft-bodied Ediacara biota in the Nama assemblage such as Pteridinium Gürich, Reference Gürich1930, Rangea Gürich, Reference Gürich1933, Charniodiscus Ford, Reference Ford1958, Namalia Germs, Reference Germs1968, and Nasepia Germs, Reference Germs1973 (and perhaps Swartpuntia Narbonne et al., Reference Narbonne, Saylor and Grotzinger1997—see Ivantsov and Fedonkin Reference Ivantsov and Fedonkin2002) are in fact also found in assemblages taxonomically assigned to both the White Sea and Avalon (Gehling and Droser Reference Gehling and Droser2013; Narbonne et al. Reference Narbonne, Laflamme, Trusler, Dalrymple and Greentree2014). This is an important detail that suggests the disparate taxonomic ordination visible for the Nama assemblage (Fig. 4: [Nam_hf], [Nam_aa], [Nam_sw], [Car_SB], [DeaV3], [Muz2Sh]) is mostly due to the absence of diverse White Sea clades (Bilateralomorphs, Dickinsoniomorpha, and Triradialomorpha) from the terminal Ediacaran rather than the emergence of novel taxa (such as at [Gaoj] and [Lijian] localities; Fig. 2). The extent to which this represents a genuine biological signal has been explored in recent studies (Gehling and Droser Reference Gehling and Droser2013; Darroch et al. Reference Darroch, Sperling, Boag, Racicot, Mason, Morgan, Tweedt, Myrow, Johnston, Erwin and Laflamme2015), which recognize that the absence of White Sea fauna in less diverse Nama-aged strata of analogous paleoenvironmental and taphonomic conditions may represent a global-scale episode of protracted extinction. If the depauperate Nama assemblages do in fact represent surviving taxa with a shared ecological association with White Sea biota, this hypothesis would also posit that surviving taxa (such as Erniettomorphs and Rangeomorphs) should be composed of ecological generalists or opportunists with broad niche tolerances (see next section; Darroch et al. Reference Darroch, Sperling, Boag, Racicot, Mason, Morgan, Tweedt, Myrow, Johnston, Erwin and Laflamme2015). Such a scenario may also be reflected by the utilized morphospaces of Ediacara-type biota, which remained largely unchanged between the White Sea and Nama after initial expansion observed within the Avalonian assemblage. A lack of significant morphological diversification among Ediacara taxa thereafter may indicate that these organisms could have become developmentally or ecologically entrenched (Shen et al. Reference Shen, Dong, Xiao and Kowalewski2008) and would therefore be gradually outcompeted by skeletonizing, tubular, and trace-making animals that were increasing in both diversity and autecological complexity throughout the terminal Ediacaran.
Paleoenvironmental Distribution of the Ediacara Biota
To link temporal changes in global diversity with responses to ecological disparity in the Ediacaran record, it is critical to identify patterns in paleoenvironmental partitioning among Ediacaran taxa. We investigated the influence of paleoenvironment on our updated taxonomic ordinations and Waggoner’s (Reference Waggoner1999, Reference Waggoner2003) original assemblages by fitting a smoothed contour surface representing the relative water depths of specific localities onto ordination plots using the function ordisurf in the R package Vegan (Figs. 5, 6; Oksanen et al. Reference Oksanen, Blanchet, Kindt, Legendre, Minchin, O’Hara, Simpson, Solymos, Henry, Stevens and Wagner2015). Each of the 86 localities was assigned a value corresponding to relative water depth, using either a combination of sedimentological and lithological properties (see Supplementary Table S.2) or directly from reported interpretations in primary literature. The ordisurf function then aggregates a smooth response gradient of these water-depth values over the limit of the ordination biplot. The degree to which depth contours separate and follow the limits of the assemblages provides another visual indication of correlation between assemblages and paleoenvironments and the relative degree to which specific taxa follow depth gradients.
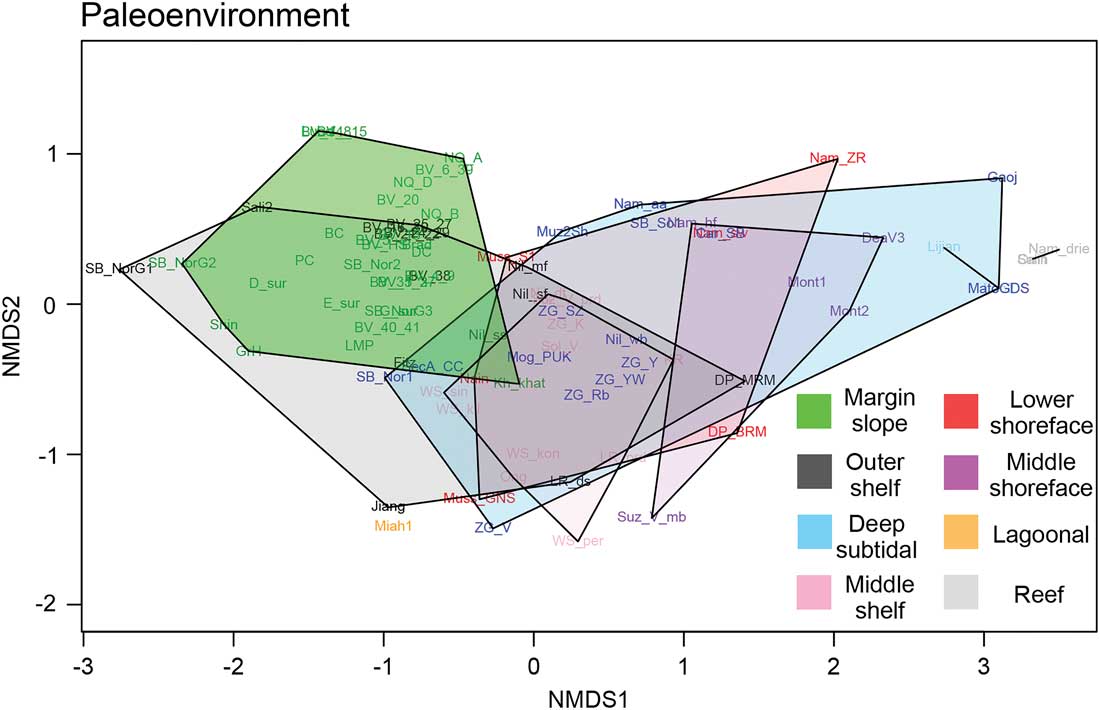
Figure 5 Ediacaran localities expressed as polygons binned by depositional–paleoenvironmental designations.
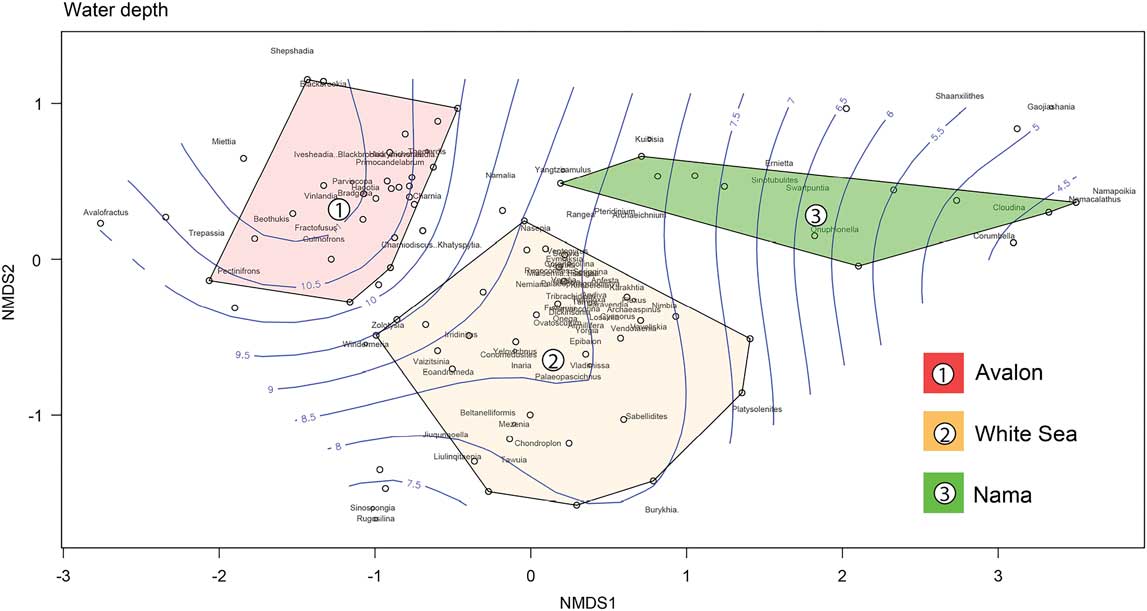
Figure 6 Relative water-depth contour averaging of localities with ordinated Ediacaran taxa overlain. From left to right: (11) slope/basin; (10) outer shelf (below SWWB); (9) middle shelf (well below FWWB, above SWWB); (8) inner shelf (between FWWB and SWWB); (7) lower shoreface (above FWWB); (6) middle shoreface; (5) upper shoreface; (4) reef margin complex. Not significant: (3) lagoonal/restricted; (2) peritidal (intertidal); (1) fluvial/deltaic. Overlain dashed polygons represent the Waggoner (Reference Waggoner2003) Avalon, White Sea, and Nama assemblages.
The results display a remarkably smooth bathymetric gradient with minimal computational stress, illustrated by a general shallowing trend from the Avalonian assemblage (left) to Nama assemblage (right) (Fig. 6). The Avalonian assemblage is highly stenotopic, correlated with deep-water slope/basinal (depth rank 11) and offshore outer shelf well below SWWB (depth rank 10) settings. The White Sea assemblage occupies a similarly narrow, but shallower bathymetric range, from offshore middle shelf (well below FWWB and near SWWB, depth rank 9) to deep subtidal inner shelf (between FWWB and SWWB, depth rank 8) (Fig. 6). Beta-diversity values display strong support for this taxonomic contrast between water depths, recording higher dissimilarity scores than either the lithology or time-bin variables (Table 2).
Perhaps the most interesting conclusion from this analysis is the departure the Nama assemblage takes from any bathymetric correlation. As discussed, latest Ediacaran communities appear to be as much defined by the absence of White Sea Ediacara-type taxa as the introduction of novel tubular and mineralizing organisms. This trend appears coincident with a significant increase in water-depth tolerance among remaining soft-bodied terminal Ediacara biota. Taxa such as Rangea, Charnia, Pteridinium, and Charniodiscus are repeatedly found in eurytopic water depths ranging from offshore middle shelf (depth rank 10: [Nil_mf], [Nil_sf]) to middle shoreface (depth rank 6: [Nam_hf]), with isolated occurrences of Namalia in depths as great as slope/basinal (depth rank 11: [SJb_Nor2]) and Charniodiscus as shallow as upper shoreface (depth rank 5: [Nil_ss]) (Bouougri and Porada 2007; Gehling and Droser Reference Gehling and Droser2013; Narbonne et al. Reference Narbonne, Laflamme, Trusler, Dalrymple and Greentree2014). Additionally, many of these taxa also display extremely long stratigraphic ranges (Narbonne et al. Reference Narbonne, Saylor and Grotzinger1997; Fedonkin et al. Reference Fedonkin, Gehling, Grey, Narbonne and Vickers-Rich2007a, and references therein; Grazhdankin et al. Reference Grazhdankin, Balthasar, Nagovitsin and Kochnev2008; Liu et al. Reference Liu, McIlroy, Matthews and Brasier2013; Narbonne et al. Reference Narbonne, Laflamme, Trusler, Dalrymple and Greentree2014). These results provide strong global evidence that depauperate Nama communities were composed of stratigraphically long-ranging cosmopolitan survivors with broad environmental and likely ecological tolerances. In contrast, although White Sea localities with apex diversity such as Nilpena (Australia) share similar bathymetry [Nil_ss], Nil_wb], [Nil_df] to those in Namibia [Nam_hf], [Nam_sw], [Nam_aa], older environmentally tolerant taxa such as Dickinsonia and Tribrachidium (see Gehling and Droser Reference Gehling and Droser2013; Hall et al. Reference Hall, Droser, Gehling and Dzaugis2015) are conspicuously absent from later Nama communities. Previous workers have suggested this absence of polyfacies taxa in the latest Ediacaran could indicate a true global extinction signal occurring abruptly at the end of the White Sea; however, it was presumed premature to conclude that the low-diversity terminal Nama assemblages represent evidence of either early extinction or changed preservational circumstances (Gehling and Droser Reference Gehling and Droser2013). As we find globally a high correlation between remaining Ediacara taxa in the present Nama assemblage and large bathymetric-range tolerance, this provides support for a model in which surviving taxa were ecological generalists that were able to colonize a latest Ediacaran Period (<549 Ma) punctuated by conditions that were now limiting to preceding Ediacarans until their ultimate disappearance at the Proterozoic/Cambrian boundary (Laflamme et al. Reference Laflamme, Darroch, Tweedt, Peterson and Erwin2013; Darroch et al. Reference Darroch, Sperling, Boag, Racicot, Mason, Morgan, Tweedt, Myrow, Johnston, Erwin and Laflamme2015).
Clearly, there were likely many other direct, indirect, and resource gradients that may have controlled the distribution of Ediacaran taxa. Oceanographic factors such as temperature, nutrient availability, and biologically relevant periods of oxygenation have profound impacts on the distribution of animals in modern marine environments that certainly translate into the stratigraphic record. These factors will undoubtedly require attention in the future as novel geochemical proxies are applied to the rock record.
Conclusions
The Ediacaran Period spans a unique and dynamic interval in the paleontological record, providing insight into both early metazoan origins at the end of the Proterozoic and emergence of profound biological and environmental drivers that would facilitate the subsequent Cambrian radiation of animal complexity. Ultimately, these mechanisms rely on well-defined chronostratigraphic constraints to illustrate any temporal interrelationships between biology and the environment on evolutionary patterns. The lack of a clear biostratigraphic record for the Ediacaran Period is a significant barrier that hinders such correlation.
Our updated paleontological database supports the taxonomic integrity of the traditional Avalon, White Sea, and Nama assemblages as coherent, distinct faunal associations as originally proposed in Waggoner (Reference Waggoner2003). However, not reflected in earlier studies is the high level of taxonomic disparity that exists in new localities such as in China when compared to the classic assemblages in Newfoundland, Russia, Australia, and Namibia. This suggests that the emerging taxonomic heterogeneities that presently exist are likely to grow as worker effort increases the known diversity in both novel and present localities. With time, this trend could potentially challenge current Ediacaran assemblage concepts and will require continued revision of the global Ediacaran data set.
Taphonomic results expand on previous studies and demonstrate that lithology (and paleoenvironment) is largely decoupled from the pervasive moldic preservation, occurring in both siliciclastic and carbonate lithofacies and at depths ranging from deep-water slope/basinal to upper shoreface. This ubiquity is reflected within ordination plots, as convex hulls for each lithology show limited separation toward any of the traditional assemblages. Some styles of moldic preservation such as Fermeuse and to a lesser degree Flinders appear to select against complete preservation of epifaunal frondose taxa. However, shallower-water localities such as Nilpena, Australia, are an exception to this trend, preserving a low diversity of frondose-specific taxa in otherwise highly diverse communities. This may therefore point to other mechanisms controlling the absence of diverse frondose communities in shallow-water environments. The Ediacaran carbonaceous compression record meanwhile displays markedly different taxonomic trends, and is highly correlated with shale and mudstone lithologies. The current carbonaceous compression window appears to select against many Ediacara-type taxa; however, due to the highly underrepresented nature of this taphonomic mode, carbonaceous compression settings are likely to be a productive facies for paleontological research moving forward.
Beyond taphonomy, both geologic time and bathymetry play an important role in understanding the Avalon, White Sea, and Nama assemblages, at least in a stratigraphic context. Despite the early first appearance of Ediacara biota in the ~579 Ma deep-water Drook Formation, Newfoundland, new geochronological data now demonstrate that the taxonomically and geographically distinct Avalonian and White Sea assemblages were likely temporally coeval at least during the Avalon–White Sea transition ca. 560–557 Ma (Noble et al. Reference Noble, Condon, Carney, Wilby, Pharaoh and Ford2014). Furthermore, both these assemblages are tightly constrained to narrow bathymetric ranges and are therefore likely to be disparate paleoenvironmental–ecological biotopes that were spatially restricted in marine settings. This patchy paleoenvironmental distribution could account for significant stratigraphic gaps in the fossil record, since bathymetrically restricted taxa will be strongly affected by gradual changes in paleoenvironmental settings. This is likely to be the case in the youngest sections of both Newfoundland and Charnwood Forest, where shallowing upward successions do not preserve the diverse frondose assemblages that occur in older, deep-water sections. Conversely, this can be argued for the absence of many White Sea taxa in older, deep-water Avalonian strata. Overall, these paleoenvironmental attributes are in opposition to the core requirements of index fossils, potentially lessening the strength for either of the Avalonian or White Sea assemblages to be used as discrete faunal stages to demarcate Ediacaran biostratigraphy.
The Nama Assemblage (549–541 Ma), meanwhile, appears to be a unique faunal stage in the terminal Ediacaran, defined by a global loss of diversity, coincident with the survival of bathymetrically unrestricted, long-ranging Ediacara-type taxa. In an extinction scenario, ecological theory predicts surviving faunal communities should be composed of generalist taxa with broad niche tolerances (Darroch et al. Reference Darroch, Sperling, Boag, Racicot, Mason, Morgan, Tweedt, Myrow, Johnston, Erwin and Laflamme2015); this appears to be directly reflected by database results. These remaining biota are coeval with the globally occurring, mineralized taxon Cloudina. Easily recognizable and distributed in carbonate stratigraphy worldwide, it appears to be an excellent index fossil candidate for a terminal Ediacaran stage–level subdivision, indicative of a globally correlative biozone ca. 549–541 Ma. The final establishment of a robust Ediacaran biostratigraphy is one step that will ultimately allow for temporal integration of fossil, biological, and environmental records to assess macroevolutionary patterns on the eve of the Cambrian radiation.
Acknowledgments
We thank P. Wagner and E. Sperling for their helpful discussion and comments. T.H.B. was supported by the University of Toronto, Mary H. Beatty Fellowship, and National Sciences and Engineering Research Council of Canada–Canada Graduate Scholarship-Master’s; S.A.F.D. thanks the Smithsonian National Museum of Natural History for financial support; and M.L. thanks the Connaught Foundation, National Sciences and Engineering Research Council of Canada, and National Geographic Society for generous funding.
Supplementary Material
Data available from the Dryad Digital Repository: http://dx.doi.org/10.5061/dryad.1mh30