Introduction
Pampaloite was discovered in drill core 315, at a depth of 71.50 m during exploration drilling at the Pampalo gold prospect (also called the Ward prospect), that was later developed as the Pampalo gold mine by Endomines AGM (Endomines, Reference Endomines2018). The Pampalo mine (Fig. 1) is located in eastern Finland close to the Russian border, 65 km east from Joensuu and 46 km north of Ilomantsi village (Nurmi et al., Reference Nurmi, Sorjonen-Ward, Damstén, Nurmi and Sorjonen-Ward1993). The deposit is within the Archaean Hattu metasedimentary schist belt that extends 40 km south to north and continues on both directions across the Russian border to Eastern Karelia. The Hattu schist belt consists of hydrothermally altered and tectonically sheared biotite tonalities and metasedimentary epiclastic and felsic pyroclastic deposits (Fig. 1), hornblende-biotite granodiorites, submarine sulfide- and graphite-bearing metapelites altered to sericite schists, mafic and ultramafic rocks (soapstones and serpentinites), and quartz-feldspar porphyry dykes (Kojonen et al., Reference Kojonen, Johanson, O'Brien and Pakkanen1993). The Pampalo gold ore was located mainly in quartz–feldspar schist between metapelites and ultramafic intrusions (soapstones and serpentinites). Another gold ore type was found in the quartz–feldspar porphyry dykes. The gold ore lodes plunge 40° to the N. The deposit is still open in a N–S direction and more than 500 m deep (Endomines, Reference Endomines2018). The location of Pampalo drill core 315 is: 62o59'11’’N, 31o15'53’’E. A phase of similar composition is listed as an unnamed mineral (UM1984-41-Te:AuSb, Smith and Nickel, Reference Smith and Nickel2007).
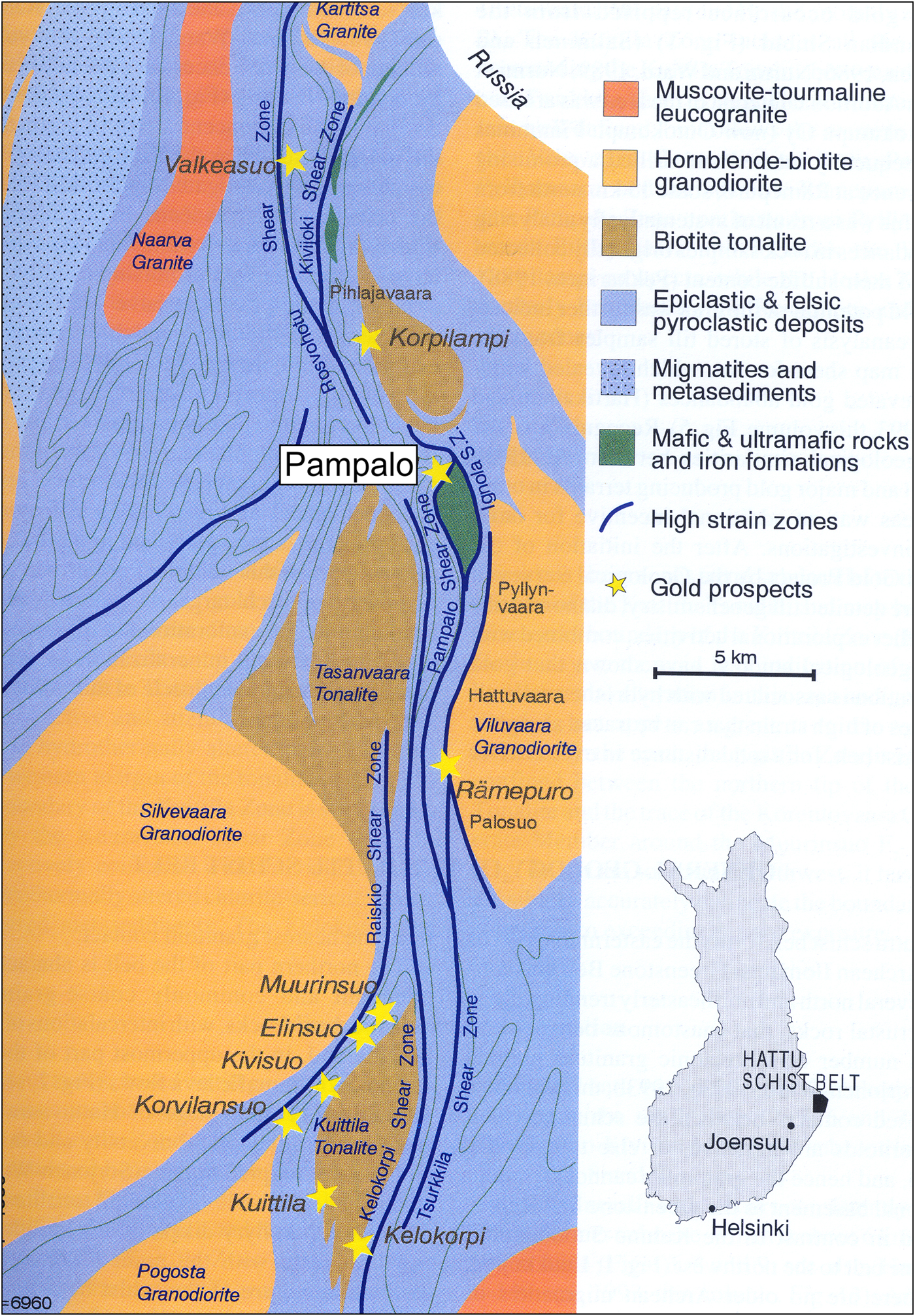
Fig. 1. Geological map of the Hattu schist belt, Ilomantsi, Eastern Finland, adapted from Nurmi et al. (Reference Nurmi, Sorjonen-Ward, Damstén, Nurmi and Sorjonen-Ward1993).
Pampaloite is intergrown with altaite, frohbergite and native gold in an open space between the gangue minerals suggesting late hydrothermal crystallisation of Te- and Sb-bearing fluids (Johanson and Kojonen Reference Johanson and Kojonen1989; Kojonen et al., Reference Kojonen, Johanson, O'Brien and Pakkanen1993; Reference Kojonen, Johanson and Pakkanen1994). The calaverite inclusions discovered in pyrite indicate high Te fugacity. Most of the gold grains found are intergrown with tellurides between the gangue minerals or in the fractures of pyrite. According to Afifi et al. (Reference Afifi, Kelly and Essene1988) this kind of Te-rich mineral paragenesis crystallised below 354°C, and typically below 250°C. Cabri (Reference Cabri1965) gives even lower values of final equilibrium for a gold–hessite–petzite association at ~50°C. The formation of pampaloite at low temperatures is also in accordance within the experimental study of the Au–Sb–Te system by Nakamura and Ikeda (Reference Nakamura and Ikeda2002), where the AuSbTe phase was synthesised at 350°C.
Both mineral and name were approved by the Commission on New Minerals, Nomenclature and Classification of the International Mineralogical Association (IMA2017-096, Vymazalová et al., Reference Vymazalová, Kojonen, Laufek, Johanson, Stanley, Plášil and Halodová2018). The mineral is named for the locality, the Pampalo gold mine, Finland. The holotype (polished thin section) is deposited at the Department of Earth Sciences of the Natural History Museum, London, UK, catalogue No BM 2017, 16.
Appearance, physical and optical properties
Pampaloite forms anhedral grains (up to 20 μm in diameter) in aggregates with gold, frohbergite and altaite (Fig. 2). Other ore minerals include pyrite, pyrrhotite, chalcopyrite, cubanite, galena, sphalerite, mackinawite, molybdenite, scheelite, rutile, tellurobismuthite, tsumoite, hessite, petzite, calaverite, volynskite, rucklidgeite, melonite, native bismuth, goethite, hematite, magnetite and chromite (Kojonen et al., Reference Kojonen, Johanson, O'Brien and Pakkanen1993). The most abundant gangue minerals are quartz, plagioclase, K-feldspar, biotite, muscovite, calcite and chlorite-group minerals; accessory minerals include garnet, staurolite, kyanite, tourmaline, rutile, titanite, apatite, zircon and epidote. The ultramafic wall rocks contain talc, carbonate, actinolitic amphibole, biotite and chlorite-group minerals.

Fig. 2. Pampaloite (pam) intergrown with native gold (yellow) altaite (al) and frohbergite (fr). Microscope image, reflected polarised light. Sample No. Ku 18516.
Pampaloite is opaque with a metallic lustre. The mineral is brittle. Values of VHN25 measured from five indentations is in the range of 245 to 295 kg/mm2 (measured on synthetic grains), with a mean value of 276 kg/mm2, which corresponds to a Mohs hardness of ~4–5. The density calculated on the basis of the empirical formula is 9.33 g/cm3. In plane-polarised reflected light, pampaloite is white (slightly bluish white in grains of synthetic material showing multiple orientations), has medium to strong bireflectance, weak reflectance pleochroism from slightly pinkish brown to slightly bluish white (only visible in grains of synthetic material containing multiple orientations), and strong anisotropy with blue to light brown rotation tints. It exhibits no internal reflections.
Reflectance measurements were made in air relative to a WTiC standard on both natural and synthetic pampaloite using a J & M TIDAS diode array spectrometer attached to a Zeiss Axiotron microscope. The results are tabulated (Table 1) and illustrated in Fig. 3.

Fig. 3. Reflectance data for natural pampaloite compared to synthetic pampaloite in air. The reflectance values (R%) are plotted versus wavelength (λ in nm).
Table 1. Reflectance data for natural and synthetic pampaloite.

The values required by the Commission on Ore Mineralogy are given in bold.
Composition
Chemical analyses were performed with a CAMECA SX-100 electron probe microanalyser in wavelength-dispersive mode using an electron beam focussed to 1–2 μm. Pure elements were used as standards. Concentrations were quantified on TeLα, SbLα and AuMα with an accelerating voltage of 15 keV, and a beam current of 10 nA on the Faraday cup. Other elements were below the detection limit.
The electron-microprobe results are given in Table 2. The empirical formulae calculated on the basis of 3 apfu are Au1.00Sb1.00Te1.00 for natural pampaloite and Au0.99Sb1.00Te1.01 for its synthetic analogue, with ideal formulae AuSbTe.
Table 2. Electron-microprobe analyses of natural and synthetic pampaloite.

Synthetic analogue
The small size and tiny intergrowths of pampaloite with altaite, frohbergite and native gold prevented its extraction and isolation in an amount sufficient for the relevant crystallographic and structural investigations. Therefore, these investigations were performed on the synthetic AuSbTe.
The synthetic phase AuSbTe was prepared in an evacuated and sealed silica-glass tube in a horizontal furnace in the Laboratory of Experimental Mineralogy of the Czech Geological Survey in Prague. To prevent loss of material to the vapour phase during the experiment, the free space in the tube was reduced by placing a closely fitting silica glass rod against the charge. The temperature was measured with Pt–PtRh thermocouples and is accurate to within ± 3°C. A charge of ~300 mg was carefully weighed out from the native elements. We used, as starting chemicals, gold powder (Aldrich Chemical Co., 99.999% purity), antimony pebbles (Aldrich Chemical Co., 99.999% purity), and a tellurium ingot (Aldrich Chemical Co., 99.95% purity). The starting mixture was first melted at 1000°C for two days, quenched and then ground in an agate mortar under acetone and reheated to 350°C for almost one year. The experimental product was not however homogenous and therefore the sample was heated for an additional nine months at 400°C which resulted in an equilibrated homogenous phase (Fig. 4). The sample was quenched by dropping the capsule in cold water.

Fig. 4. Synthetic pampaloite (AuSbTe) heated: (a) for 12 months at 350°C; and an unequilibrated sample (b) heated for an additional 9 months at 400°C until equilibrium was attained.
X-ray crystallography
Single-crystal diffraction of synthetic pampaloite
A small fragment 33 μm × 22 μm × 14 μm of synthetic AuSbTe was mounted on a glass fibre and examined using a Rigaku Super Nova single-crystal diffractometer with an Atlas S2 CCD detector utilising MoKα radiation, provided by a microfocus X-ray tube and monochromatised by primary mirror optics. The ω rotational scans were used for collection of three-dimensional intensity data. From a total of 2016 reflections, 760 were independent and, 664 were classified as unique with I > 3σ(I). Corrections for background, Lorentz effects and polarisation were applied during data reduction with the CrysAlis software. Corrections for absorption for spherical sample were applied with the JANA2006 program (Petříček et al., Reference Petříček, Dušek and Palatinus2014). The crystal structure was solved with a charge-flipping method using the program Superflip (Palatinus and Chapuis, Reference Palatinus and Chapuis2007) and subsequently refined by the full-matrix least-squares algorithm of JANA2006 program (Petříček et al., Reference Petříček, Dušek and Palatinus2014). The initial crystal structure solution contains three atomic sites in the asymmetric unit. One site was identified as an Au atom, and two sites as Sb atoms. All of the sites are in a general Wyckoff position 8f of the space group C2/c. Considering the composition is AuSbTe, it is obvious that Te should be placed in the structure. Owing to the similarity of X-ray scattering curves of Sb and Te atoms, it is difficult to distinguish these atoms using X-ray diffraction (XRD) data alone. Anisotropic refinement with Te and Sb atoms placed at the positions indicated in Table 3 (i.e. ordered structure), converged to R = 0.0311 and wR = 0.0777 for 664 reflections. Refinement of the pampaloite structure with inverse occupation of the positions (i.e. Sb on Te site and vice versa) led to the slight increase of R factors to R = 0.0324 and wR = 0.0837. Refinement of a structure with a random distribution of Sb and Te atoms (i.e. two Sb/Te mix sites with 0.5/0.5 occupancy) yielded R = 0.0320 and wR = 0.0800. The crystal-chemical environment of Sb and Te positions is very similar (see structure description) and thus cannot be used to decipher the Sb/Te occupancy. Nevertheless, the non-existence of a solid solution of pampaloite in the Au–Sb–Te system (Nakamura and Ikeda, Reference Nakamura and Ikeda2002), moderate temperature of synthesis of AuSbTe used in the structure solution, stoichiometry of the crystallographic positions 1:1:1 in the pampaloite structure corresponding to the stoichiometry of the mineral, suggest that there is very likely to be an ordered distribution of Sb and Te atoms in its crystal structure. A certain degree of disorder at Sb and Te positions cannot be ruled out. However, Sb and Te atoms are vital for pampaloite formation; analogous compounds containing only Au and Sb or Te, respectively (i.e. AuSb2 and AuTe2) show different crystal structures (see below). Thus, the structure of pampaloite was refined assuming distinct Sb and Te positions.
Table 3. Fractional coordinates and anisotropic displacement parameters (Å2) for synthetic pampaloite.

By analogy with the montbrayite structure (Bindi et al., Reference Bindi, Paar and Lepore2018), where the Au–Sb substitution was observed, the occupancy of the Au position was also refined (Au versus vacancy). The refinement indicated a fully occupied Au position. All atoms were refined with anisotropic displacement parameters. Refinement for 28 parameters converged to R = 0.0311 and wR = 0.0777 for 664 reflections. Details of data collection, crystallographic data and refinement for the ordered structure model are summarised in Table 4. Table 5 shows selected bond lengths. The crystallographic information files have been deposited with the Principal Editor of Mineralogical Magazine and are available as Supplementary material (see below).
Table 4. Crystallographic data for the selected crystal of synthetic pampaloite AuSbTe.

Table 5. Selected bond distances (<3.5 Å) in the pampaloite crystal structure.

Powder XRD of synthetic pampaloite
The powder XRD pattern of synthetic pampaloite was collected in the Bragg–Brentano geometry on a Bruker D8 Advance diffractometer equipped with the LynxEye XE detector and CuKα radiation. The data were collected in the range from 15° to 90°2θ with a step size of 0.005°2θ and 1 s counting time per step. The structure model obtained from a single-crystal XRD study of synthetic pampaloite was used as a starting structural model in the subsequent Rietveld refinement. The FullProf program (Rodríguez-Carvajal, Reference Rodríguez–Carvajal2006) and the pseudo-Voigt function were used to generate the shape of the diffraction peaks. The refined parameters include those describing peak shape and width, peak asymmetry, unit-cell parameters, and an overall isotropic displacement parameter. In total, 12 parameters were refined. No fractional coordinates were refined. The refinement indicated 1.5 wt.% Sb2Te3 impurity in the sample of synthetic pampaloite investigated. Table 6 summarises the results of the Rietveld refinement; Fig. 5 shows the final Rietveld plot. Table 7 presents the powder XRD data.

Fig 5. The observed (circles), calculated (solid line) and difference Rietveld profiles for synthetic pampaloite. The upper reflection markers correspond to synthetic pampaloite, and the lower markers to 1.5 wt.% Sb2Te3 impurity.
Table 6. Powder diffraction data collection and Rietveld analysis of a synthetic pampaloite.

Table 7. Powder XRD data for synthetic pampaloite (CuKα radiation).

The strongest lines are given in bold
Structure description
The crystal structure of pampaloite is based on deformed hexagonal packing of Sb and Te atoms where a half of the available octahedral voids is filled with Au atoms. As indicated in Fig 6, the Au atom shows 4 + 1+1 coordination by three Sb and three Te atoms with bond distances ranging from 2.693(1) to 3.257(1) Å (Table 5). Consequently, Au atoms form [AuSb3Te3] elongated octahedra having Sb and Te atoms in a facial (fac) configuration. The coordination environment of Sb and Te atoms is similar. Both atoms have three short contacts with Au showing 2 + 1 bonding scheme. In addition, they form characteristic Sb–Te dumbbells with a bond distance of 2.829(2) Å. Contrary to the Te position, the Sb position shows one additional short contact with Sb at a distance of 3.270(2) Å. The Sb–Te separation of 2.829(2) Å in the pampaloite structure distance is slightly shorter than the Sb–Sb bond distance of 2.908 Å in the structure of native Sb (Barrett et al., Reference Barrett, Cucka and Haefner1963) and comparable to that of 2.834 Å observed in native Te (Adenis et al., Reference Adenis, Langer and Lindqvist1989). Similar bonding distances of 2.84 Å and 2.85 Å were observed between Te and Te atoms in the structure of krennerite Au3AgTe8 (Dye and Smyth, Reference Dye and Smyth2012) and stützite Ag5−xTe3 (Bindi and Keutsch, Reference Bindi and Keutsch2018), respectively. Comparable bonding distances of 2.879 Å were also observed in the structure of synthetic PdSbTe (Foecker and Jeitschko, Reference Foecker and Jeitschko2001), which can be considered as an ordered derivative of the pyrite structure with the [SbTe] dimeric anions. The Sb–Te separations in pampaloite are even shorter than the Sb–Te distances in an octahedral double layer found in Sb2Te3 (2.9789(5) and 3.1680(4) Å, Anderson and Krause, Reference Anderson and Krause1974).

Fig. 6. (a) Polyhedral representation of the pampaloite structure emphasising the [AuTe3Sb3] octahedra. The Sb-Te bonds connecting the layers are indicated by green lines. (b) Detailed view of one layer composed of the edge-sharing [AuTe3Sb3] octahedra.
The [AuSb3Te3] octahedra share Sb–Sb, Te–Te and Sb–Te edges and form sheets oriented parallel to ($\bar{1}$01) planes. The octahedral sheets are connected by Sb–Te dumbbells across the interlayer space (Fig 6). The structure model of pampaloite presented in this work strongly resembles the average structure of calaverite AuTe2 (Bindi et al., Reference Bindi, Arakcheeva and Chapuis2009; Schutte and de Boer, Reference Schutte and Boer1988), sylvanite AuAgTe4 (Pertlik, Reference Pertlik1984) and muthmannite AuAgTe2 (Bindi and Cipriani, Reference Bindi and Cipriani2004). In all structures, Au show more or less distorted octahedral coordination.
Whereas the sheets of the edge-sharing [AuTe6] octahedra in the average structure of calaverite are linked by Te–Te bonds, the sheets of [AuSb3Te3] octahedra in the pampaloite structure are connected by a system of Sb–Te bonds. In the sylvanite structure, [AuTe6] and [AgTe6] octahedra share form sheets, which are combined to a network by means of Te–Te dumbbells (2.82 Å). Contrary to that, the sheets of [AuTe6] octahedra in muthmannite are connected by sheets of [AgTe6] octahedra. Moreover, the structure of calaverite is incommensurately modulated (Bindi et al., Reference Bindi, Arakcheeva and Chapuis2009; Schutte and de Boer, Reference Schutte and Boer1988).
The pampaloite structure crystalises in a unique structure type, no exact structural analogues have been observed. It can be considered as a monoclinic derivative of the CdI2 structure type. From a chemical point of view, pampaloite is very close to aurostibite AuSb2 and montbrayite (Au,Ag,Sb,Pb,Bi)23(Te,Sb,Pb,Bi)38, however its crystal structure differs from the above mentioned minerals. Aurostibite shows a three-dimensional network composed of corner-sharing [AuSb6] octahedra (Furuseth et al., Reference Furuseth, Selte and Kjekshus1965) whereas pampaloite shows a layered structure. In the montbrayite structure (Bindi et al., Reference Bindi, Paar and Lepore2018), the [AuTe6] octahedra form edge-sharing octahedral chains along [101], generating empty spaces with Te–Te contacts (from 2.83 to 2.90 Å).
Proof of identity of natural and synthetic pampaloite
The structural identity between the synthetic AuSbTe and the natural material was confirmed by electron back-scattered diffraction (EBSD). For that purpose, we used a TESCAN Mira 3GMU scanning electron microscope combined with an EBSD system (NordlysNano detector, Oxford Instruments). The natural sample was prepared for investigation by re-polishing the surface with colloidal silica (OP-U) for 20 min to reduce the surface damage. The EBSD patterns were collected and processed using a proprietary computer program AZtec HKL (Oxford Instruments). The solid angles calculated from the patterns were compared with a synthetic AuSbTe match containing 76 reflectors to index the patterns. The Kikuchi patterns obtained from the natural material (seven measurements on different spots on natural pampaloite) were found to match the patterns generated from the structure of synthetic AuSbTe provided by our crystal-structure determination (Fig. 7). The values of the mean angular deviation (MAD) between the calculated and measured Kikuchi bands range between 0.32° and 0.27°. These goodness-of-fit values reveal a very good match; MAD <1° are considered as indicators of an acceptable fit.

Fig. 7. EBSD image of natural pampaloite; in the right pane, the Kikuchi bands are indexed.
The EBSD study, chemical identity and optical properties confirmed the correspondence between natural and synthetic materials and thereby legitimise the use of the synthetic phase for the complete characterisation of pampaloite.
Acknowledgements
The authors acknowledge Ulf Hålenius, Chairman of the CNMNC and its members for helpful comments on the submitted data. This work was supported by the Grant Agency of the Czech Republic (project No. 18-15390S) and through an internal project 331400 from the Czech Geological Survey. The authors are grateful to Dr. Milan Drábek (Czech Geological Survey) for the help with the experimental work and Dr. Zuzana Korbelová (Institute of Geology AS CR, v.v.i.) for carrying out the electron microprobe analyses. JP thanks the project no. LO1603 under the Ministry of Education, Youth and Sports National sustainability program I of the Czech Republic. CJS acknowledges Natural Environment Research Council grant NE/M010848/1 Tellurium and Selenium Cycling and Supply. The reviewer's comments of Luca Bindi, Louis J. Cabri, Peter Leverett and Editor Stuart Mills are greatly appreciated.
Supplementary material
To view supplementary material for this article, please visit https://doi.org/10.1180/mgm.2018.129