Introduction
A decade ago, the amount of structurally characterised anhydrous sulfates of copper and alkali metals was very limited. Most of the known compounds belonged to minerals from volcanic fumaroles. Euchlorine, KNaCu3O(SO4)3 (Scordari and Stasi, Reference Scordari and Stasi1990; Siidra et al., Reference Siidra, Borisov, Lukina, Depmeier, Platonova, Colmont and Nekrasova2019a), and chlorothionite, K2Cu(SO4)Cl2 (Giacovazzo et al. Reference Giacovazzo, Scandale and Scordari1976), were discovered more than a century ago in fumaroles of Vesuvius volcano, Italy. Much later, after the Great Fissure Tolbachik Eruption in Kamchatka in 1975–1976, a large number of new endemic anhydrous copper sulfates were identified (Vergasova and Filatov, Reference Vergasova and Filatov2012). The overwhelming majority of the latter belonged to new structure types and had no known synthetic analogues. A well-known characteristic of transition metal sulfates is their excellent solubility and hydration in air resulting in the appearance of a large number of reaction by-products. The latter requires special conditions during transportation and work in the laboratory. Despite the significant number of articles on copper and alkali metal sulfates that have appeared in the last few years, rubidium copper sulfates are almost unknown. It is known that in the series of alkali metals from Na to Cs in the structures of oxides and oxysalts the phenomenon of morphotropism is observed commonly. As a rule, potassium and rubidium representatives are typically isostructural, whereas sodium and caesium examples can adopt their own structure types.
The wave of interest in anhydrous transition metal sulfates and alkalis in the 2010s is associated with access to specific magnetic cluster-topology (Fujihala et al., Reference Fujihala, Koorikawa, Mitsuda, Morita, Tohyama, Tomiyasu, Koda, Okabe, Itoh, Yokoo, Ibuka, Tadokoro, Itoh, Sagayama, Kumai and Murakami2017, Reference Fujihala, Sugimoto, Tohyama, Mitsuda, Mole, Yu, Yano, Inagaki, Morodomi, Kawae, Sagayama, Kumai, Murakami, Tomiyasu, Matsuo and Kindo2018; Furrer et al., Reference Furrer, Podlesnyak, Pomjakushina and Pomjakushin2018), and also with the search for novel electrode materials for possible application as cathodes in rechargeable metal-ion batteries (Masquelier and Croguennec, Reference Masquelier and Croguennec2013). For such applications sulfates represent a promising class of compounds given their superior electronegativity and high redox potential by the inductive effect (Rousse and Tarascon, Reference Rousse and Tarascon2014).
In addition to experimental work aimed at the synthesis of rubidium analogues of anhydrous potassium and sodium sulfates, one of the goals of this work was also an analysis and a brief review, below, of the geochemistry of rubidium in volcanic environments.
Geochemistry of rubidium in volcanic environments
Quaternary arc volcanism on the Kamchatka peninsula, Russia, produced a huge volume of basaltic rocks which form numerous large volcanoes and monogenetic volcanic fields. Among the active volcanoes the most well known and studied is the Tolbachik volcanic complex; it is a part of the Klyuchevskaya Volcanic Group in the Central Kamchatka Depression (Churikova et al., Reference Churikova, Gordeychik, Edwards, Ponomareva and Zelenin2015a). The Tolbachik complex consists of two stratovolcanoes, extinct Ostry Tolbachik and active Plosky Tolbachik, and a monogenetic volcanic field known as Tolbachinsky Dol. The latter was formed during several eruptions including the Great Fissure Tolbachik Eruption in 1975–76 and the Tolbachik fissure eruption in 2012–2013 (Volynets et al., Reference Volynets, Edwards, Melnikov, Yakushev and Griboedova2015).
The large volume of erupted lavas due to Tolbachik's recent activity is well studied in terms of their mineralogy and geochemistry, including direct sampling during eruptions (Churikova et al., Reference Churikova, Gordeychik, Edwards, Ponomareva and Zelenin2015a). The Tolbachik lavas and scoria consist of basalts and basaltic andesites with subordinate trachybasalts and basaltic trachyandesite. Basalts, on the basis of potassium content, are subdivided into medium- and high-K varieties; another basalt subdivision based on the MgO/Al2O3 ratio comprises high-magnesian, high-alumina and intermediate rocks. Published whole-rock geochemical data (e.g. Churikova et al., Reference Churikova, Gordeychik, Iwamori, Nakamura, Ishizuka, Nishizawa, Haraguchi, Miyazaki and Vaglarov2015b, Volynets et al., Reference Volynets, Edwards, Melnikov, Yakushev and Griboedova2015) show large variations in content of both major and trace elements, e.g. MgO = 2.1–10.7 wt.%, Na2O = 2.3–4.2 wt.%, K2O = 0.7–3.1 and Cr = 7–745 ppm. An unusual geochemical feature of the Tolbachik basalts is their enrichment in copper with 100–250 ppm in bulk rock samples (Portnyagin et al., Reference Portnyagin, Duggen, Hauff, Mironov, Bindeman, Thirlwall and Hoernle2015). On multi-element normalising diagrams (relative to N-type mid-ocean ridge basalts) the Tolbachick basaltic rocks show enrichment in large-ion lithophile elements (e.g. Cs, Rb and Ba) and depletion in high-field-strength elements (e.g. Nb or Ta). This is considered to be a typical signature of subduction-related magmas (Churikova et al., Reference Churikova, Dorendorf and Wörner2001). Mineralogy, trace-element geochemistry and data from Sr–Nd–Pb isotopic studies suggest involvement of several components during formation of primary melts including subducted Pacific sediments, oceanic basaltic crust with additional fluid flux from subducted material (Churikova et al., Reference Churikova, Dorendorf and Wörner2001; Portnyagin et al., Reference Portnyagin, Duggen, Hauff, Mironov, Bindeman, Thirlwall and Hoernle2015) and assimilation of ore-bearing hydrothermal veins at the crustal level (Zelenski et al., Reference Zelenski, Kamenetsky and Hedenquist2016).
Tolbachik is also well known due to strong fumarolic activity and remarkable mineralogy of fumarolic deposits. They have been studied since 1976 and hundreds of new minerals with unusual combinations of both abundant and trace elements have been found and described in detail (Vergasova and Filatov, Reference Vergasova and Filatov2012; Pekov et al., Reference Pekov, Zubkova and Pushcharovsky2018a). Particularly abundant are sulfate minerals with Na+ and K+ as major cations. Other alkaline elements, Rb and Cs, are rare in fumarolic minerals. Yet, some species contain these elements in trace to minor amounts (up to 1.95 wt.% Rb2O in cesiodymite and 4.11 wt.% Cs2O in averievite, Pekov et al., Reference Pekov, Zubkova, Agakhanov, Pushcharovsky, Yapaskurt, Belakovskiy, Vigasina, Sidorov and Britvin2018b, Vergasova et al., Reference Vergasova, Starova, Filatov and Anan'ev1998) including cesiodymite that contains Cs as the major constituent (Pekov et al., Reference Pekov, Zubkova, Agakhanov, Pushcharovsky, Yapaskurt, Belakovskiy, Vigasina, Sidorov and Britvin2018b).
Rubidium, the element on which this paper is focused, is a trace element in Tolbachik basaltic rocks with concentrations between 13 ppm (primitive high-Mg basalts) and 89 ppm (evolved low-Mg basaltic andesites). RbN values (normalised relative to N-MORD) vary from 24 to 159. On Harker-type diagrams, the element exhibits a clear positive correlation with K2O (Supplementary Fig. 1) and this relationship seems to be common for the Quaternary volcanic rocks from the Kurile–Kamchatka region (Popolitov and Volynets, Reference Popolitov and Volynets1982). In basalts, Rb is likely to be hosted by plagioclase pheno- and microphenocrysts and possibly by the groundmass glass.
Rubidium is also present in high-temperature magmatic gases (1030–1060°C) sampled during the 2012–13 eruption and considered to be uncontaminated. The measured Rb content in condensate was 1.0±0.2 ppm (Zelenski et al., Reference Zelenski, Malik and Taran2014) and 1.7 ppm (Chaplygin et al., Reference Chaplygin, Lavrushin, Dubinina, Bychkova, Inguaggiato and Yudovskaya2016). A slightly lower concentration of Rb (0.78 ppm) was measured in the gases of the 1975–1976 eruption (Menyailov and Nikitina, Reference Menyailov and Nikitina1980). In contrast, the Rb content in the low-temperature gas (690°C) from the 2012–13 eruption was found to be outstandingly high at 16.5 ppm (Chaplygin et al., Reference Chaplygin, Lavrushin, Dubinina, Bychkova, Inguaggiato and Yudovskaya2016). However, the strong enrichment of trace elements in the gas was explained as a secondary feature due to “evaporation at forced pumping during sampling and possible dissolution of earlier precipitated sublimates in the gas conduit” (Chaplygin et al., Reference Chaplygin, Lavrushin, Dubinina, Bychkova, Inguaggiato and Yudovskaya2016). Despite the low Rb content in Tolbachik gases, it is essentially above that in gases produced by other volcanoes. For instance, exhalations of Kudryavy, Kurily, Russia; Gorelyi, Kamchatka, Russia; Erta Ale, Afar, Ethiopia; Mount St. Helens, USA; and White Island, New Zealand volcanoes exhibit extremely low Rb content in condensate with values between 0.22 and 0.002 ppm (Tedesco and Toutain, Reference Tedesco and Toutain1991; Symonds and Reed, Reference Symonds and Reed1993; Taran et al. Reference Taran, Hedenquist, Korzhinsky, Tkachenko and Shmulovich1995; Zelenski et al., Reference Zelenski, Fischer, de Moor, Marty, Zimmermann, Ayalew, Nekrasov and Karandashev2013; Chaplygin et al., Reference Chaplygin, Taran, Dubinina, Shapar and Timofeeva2015). The high enrichment factor for Rb in Tolbachik gases (LogEFRb = 2–3, Chaplygin et al., Reference Chaplygin, Lavrushin, Dubinina, Bychkova, Inguaggiato and Yudovskaya2016; Zelenski et al., Reference Zelenski, Malik and Taran2014) suggests that the element was transported in the form of volatile species from a source and not derived from wall rocks due to contamination.
To date, whole-rock compositional data for basalts with different degrees of alteration by fumarolic gases are still lacking and one can but suggest the tentative mechanisms of gas enrichment by the trace elements to the levels essential for the crystallisation of Rb-enriched minerals. For instance, such strong Rb enrichment could be due to its extraction by hot hydrated gases from the plagioclase and glass, and/or dissolution of some earlier precipitated fumarolic minerals, e.g. sulfates, as suggested by Chaplygin et al. (Reference Chaplygin, Lavrushin, Dubinina, Bychkova, Inguaggiato and Yudovskaya2016). In this case, some helpful data can be collected from the results of model experiments, including chemical composition, structure, formation mechanism (chemical transport, crystallisation from melt, hydration rate etc.) and other features of synthetic Rb-containing species chemically relevant to the currently few known minerals. As yet, rubidium accumulates mostly in the copper sulfate or oxysulfate salts [alumoklyuchevskite, K3Cu3AlO2(SO4)4 (Siidra et al., Reference Siidra, Nazarchuk, Zaitsev, Lukina, Avdontseva, Vergasova, Vlasenko, Filatov, Turner and Karpov2017); belousovite, KZn(SO4)Cl (Siidra et al., Reference Siidra, Nazarchuk, Lukina, Zaitsev and Shilovskikh2018a); cesiodymite, CsKCu5O(SO4)5 and cryptochalcite, K2Cu5O(SO4)5 (Pekov et al., Reference Pekov, Zubkova, Agakhanov, Pushcharovsky, Yapaskurt, Belakovskiy, Vigasina, Sidorov and Britvin2018b); parawulffite, K5Na3Cu8O4(SO4)8 and wulffite, K3NaCu4O2(SO4)4 (Pekov et al., Reference Pekov, Zubkova, Yapaskurt, Belakovskiy, Chukanov, Lykova, Savelyev, Sidorov and Pushcharovsky2014)] and in the following two copper vanadates: (Cu5O2)(AsO4)(VO4)⋅(Cu0.5□0.5)Cl (Siidra et al., Reference Siidra, Nazarchuk, Agakhanov and Polekhovsky2019b) and averievite Cu5O2(VO4)2⋅n(Cs,Rb,K)Cl (Vergasova et al., Reference Vergasova, Starova, Filatov and Anan'ev1998). The current paper reports the results of our further studies of multinary Rb–Cu sulfates where no less than eight new compounds representing four new structure types have been observed.
Experimental
Synthesis
Potassium- and copper-bearing sulfate minerals such as chlorothionite, K2Cu(SO4)Cl2, piypite, K4Cu4O2(SO4)4⋅(Na,Cu)Cl and cryptochalcite, K2Cu5O(SO4)5, were found in fumarolic sublimates, hence the chemical vapour transport method (CVT-method) was chosen due to similarity with natural conditions of mineral formation from volcanic gases. In our case, however, most reactions did not produce essential sublimates therefore the conventional solid-state synthesis (Nekrasova et al., Reference Nekrasova, Siidra, Zaitsev, Ugolkov, Colmont, Charkin, Mentré, Chen, Kovrugin and Borisov2021) in evacuated silica tubes was preferred.
Synthesis of Rb2Cu(SO4)Cl2 and Rb4Cu4O2(SO4)4·(Cu0.83Rb0.17Cl)
Single crystals of Rb2Cu(SO4)Cl2 and Rb4Cu4O2(SO4)4⋅(Cu0.83Rb0.17Cl) were grown by slow cooling of a melt containing anhydrous CuSO4 (Prolabo, 98%) and RbCl (Alfa Aesar, 99%) in the ratio 1:2. The precursors were pre-dried at 200°C for 2 h to remove traces of absorbed water and then mixed rapidly and ground in an agate mortar in air for 5 min. The mixtures were pressed into pellets (ca. 5 mm × 2 mm) and loaded into silica ampoules (ca. 10 cm × 0.8 cm), which were evacuated (10–2 mbar) and sealed. The ampoules were placed horizontally in a tubular furnace and heated up to 700°C over 3 h and kept at 700°C for 10 h. Cooling to 550°C was performed over 72 h, and an additional 12 h was taken to reach room temperature. The products consisted of sky-blue crystals of Rb2Cu(SO4)Cl2 and green crystals of Rb4Cu4O2(SO4)4⋅(Cu0.83Rb0.17Cl) (Fig. 1).
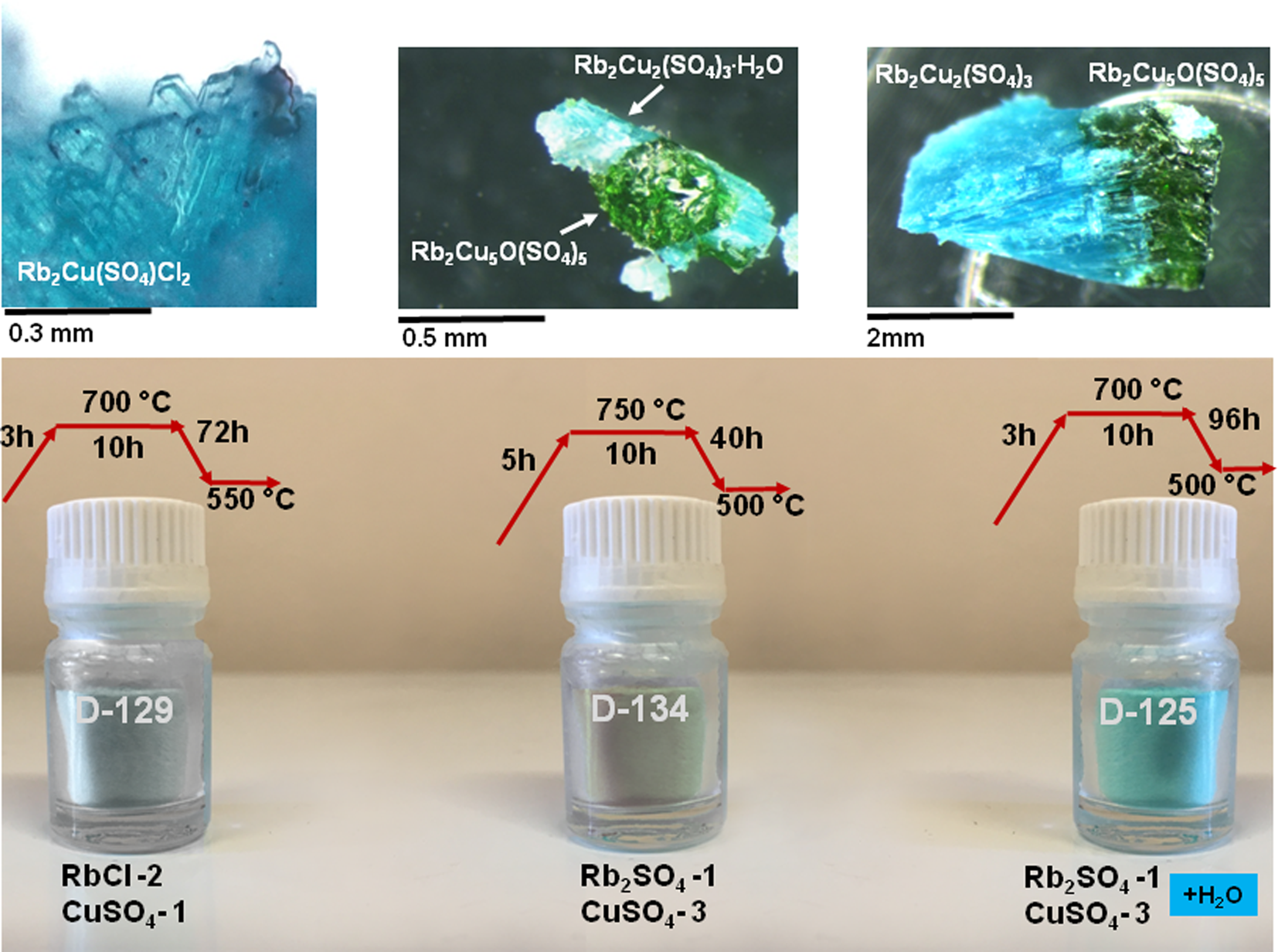
Fig. 1. Crystals of blue Rb2Cu(SO4)Cl2, green Rb2Cu5O(SO4)5, blue Rb2Cu2(SO4)3(H2O) and Rb2Cu2(SO4)3. Initial reagent mixtures and schemes of the synthesis for each compound are shown.
Synthesis of Rb2Cu2(SO4)3 and Rb2Cu5O(SO4)5
Crystals of Rb2Cu2(SO4)3 and Rb2Cu5O(SO4)5 were formed in the same experimental run. Rb2SO4 (Sigma-Aldrich, 99.99%) and CuSO4 (Prolabo, 98%) were mixed carefully in an agate mortar in the ratio 3:1, pelletised, and sealed as described above. The ampoule was heated up to 700°C for 3 h, soaked for 10 h, cooled to 500°C in 96 h and further cooled in 12 h to room temperature. The products consisted of green Rb2Cu5O(SO4)5 and sky-blue Rb2Cu2(SO4)3 crystals (Fig. 1).
Synthesis of Rb2Cu2(SO4)3(H2O)
The new compound Rb2Cu2(SO4)3(H2O) was synthesised during a side process. A 3:1 mixture of Rb2SO4 (Sigma-Aldrich, 99.99%) and CuSO4 (Prolabo, 98%) was exposed to air for one week. The colour of the mixture turned light blue due to hydration of copper sulfate. The mixture was processed as described above; the ampoule was heated to 750°C in 5 h, soaked for 10 h, then cooled to 500°C over a period of 40 h, after which the furnace was switched off. The products consisted of anhydrous green crystals of Rb2Cu5O(SO4)5 and hydrated light-blue crystals of Rb2Cu2(SO4)3(H2O).
Synthesis of RbMCu(SO4)2 (M = Na, K, Rb)
These three new compounds were prepared according to a slightly different protocol. Mixtures of Rb2SO4, Na2SO4, or K2SO4 (all pre-heated at 140°C for 3–4 h) and CuSO4 (obtained by dehydration of CuSO4⋅5H2O at 450°C for 4 h) were mixed in a 1:1:2 molar ratio, ground, and placed in silica capsules (5 mm inner diameter, 100 mm long). The tubes were evacuated to 3⋅10–2 Torr, flame sealed and placed in a vertical furnace with ‘cold’ ends protruding out so that any absorbed water would condense therein. The mixtures were annealed first at 350°C for 96 h; the ampoules were opened, inhomogeneous samples re-ground and further annealed at 450–475°C for more 96 h. The samples partially melted and formation of green or blue crystals occurred on cooling.
Though the hygroscopicity of the compounds in discussion was not studied, it is very likely that these compounds would hydrate readily in moist air. High hygroscopicity and rapid hydration was reported for several potassium analogues, e.g. K2Cu(SO4)2 (Zhou et al., Reference Zhou, Liu, Ang and Zhang2020) and K2Cu2(SO4)3 (Lander et al., Reference Lander, Rousse, Batuk, Colin, Dalla Corte and Tarascon2017); most natural copper sulfate minerals are also unstable in moist air (Siidra et al., Reference Siidra, Borisov, Lukina, Depmeier, Platonova, Colmont and Nekrasova2019a, Reference Siidra, Borisov, Charkin, Depmeier and Platonova2021a). The most likely reason for such behaviour is non-rigidity of the coordination sphere of both alkali cations (due to weak binding to any ligands) and copper (due to the pronounced Jahn–Teller effect). An indirect indication comes from the observation that evacuation of silica tubes containing re-ground samples was relatively slow, most likely due to elimination of water absorbed upon grinding. Nonetheless, the complex sulfates seem to be less hygroscopic compared to CuSO4 and Rb2SO4.
Single-crystal X-ray studies
Single crystals of all the compounds were isolated visually under a binocular microscope and mounted on thin glass fibres for X-ray diffraction analysis using a Bruker APEX II DUO X-ray diffractometer with a micro-focus X-ray tube operated with MoKα radiation at 50 kV and 0.6 mA. The data were integrated and corrected for absorption using a multi-scan type model implemented in the Bruker programs APEX and SADABS (Bruker, 2014). More than a hemisphere of X-ray diffraction data was collected for each crystal. The structure determinations and refinements were performed using SHELXL software (version 2018/3) (Sheldrick, Reference Sheldrick2015). Crystallographic information for all obtained phases is summarised in Table 1. Atomic coordinates and additional structural information are provided in the crystallographic information files and are available as Supplementary material (see below).
Table 1. Crystallographic data for the new anhydrous alkali copper sulfates.

Results
Structures without additional O2– anions.
Rb2Cu(SO4)2
Statistics of diffraction intensities and systematic extinctions were consistent with the space group Pna21. The |E 2–1| parameter was equal to 0.668, which clearly indicated high probability of a non-centrosymmetry (NCS) (Marsh, Reference Marsh1995), confirmed by subsequent structure solution and refinement.
The asymmetric unit contains one symmetrically independent Cu2+, two Rb+ and two S6+ cations. All Cu–O bonds ≤3.05 Å and Rb–O bonds ≤3.55 Å were taken into consideration. The Cu1 atom forms four very strong Cu–Oeq bonds (≤ 2 Å) resulting in a CuO4 square which is complemented by a fifth, longer Cu–Oap bond of 2.182 Å, to form a CuO5 distorted tetragonal pyramid (Fig. 2). The Cu1 atom forms one additional long bond of 2.994(4) Å, resulting in a [4+1+1] CuO6 octahedron strongly distorted by the Jahn–Teller effect. Such ‘Jahn-Teller’ type of coordination geometry of Cu2+ cations is rather common in minerals and inorganic materials (Burns and Hawthorne, Reference Burns and Hawthorne1995).

Fig. 2. Coordination environments of Rb+, Cu2+ and S6+ cations (top) in the crystal structure of Rb2Cu(SO4)2. General projections of the crystal structure of Rb2Cu(SO4)2 along the c and a axis, respectively (bottom).
Both Rb cations centre irregular polyhedra of oxygen atoms (eight vertices for Rb1 atom аnd nine for Rb2). Each of two S sites centres the respective tetrahedra. The average S–O bond-lengths, 1.47 Å, are consistent with the value of 1.475 Å reported for sulfate minerals (Hawthorne et al., Reference Hawthorne, Krivovichev, Burns, Alpers, Jambor and Nordstrom2000).
The CuO6 polyhedra share O3–O5 and O4–O5 oxygen edges and O1 and O7 vertices with SO4 tetrahedra forming clusters depicted in Fig. 2. These are linked into an open framework with a three-dimensional system of channels occupied by the Rb+ cations. The structural topology of the [Cu(SO4)2]2– framework in Rb2Cu(SO4)2 is unique and has not been observed previously. The projection in the bc plane provides good evidence of the ‘up’ only orientation of all the sulfate groups, along the polar 21 c-axis, confirming the NCS character of this material.
The Cu1–S1 and Cu1–S2 distances in Rb2Cu(SO4)2 are 2.5718(13) Å and 2.9737(12) Å, respectively. In fact, edge sharing between sulfate tetrahedra and transition metal-centred octahedra is rather uncommon. A similarly short Cu–S distance of 2.593 Å is observed in chlorothionite, K2CuCl2(SO4) (Giacovazzo et al., Reference Giacovazzo, Scandale and Scordari1976) wherein the CuO6 octahedron shares an edge with a SO4 group. Edge sharing has also been observed recently in the complex Zn sulfate majzlanite, K2Na(ZnNa)Ca(SO4)4 (Siidra et al., Reference Siidra, Nazarchuk, Zaitsev and Shilovskikh2020), with a Zn–S distance of 2.8704(4) Å.
RbNaCu(SO4)2 and RbKCu(SO4)2
RbNaCu(SO4)2 and RbKCu(SO4)2 are isotypic and crystallise in the monoclinic space group C2/c. The lattice parameters given in Table 1 show a strong unit-cell expansion by 7.62% on replacement of Na by K. Both structures contain, in their asymmetric units, one symmetrically independent Cu site, two S sites and three A (A = Rb, K and Na) sites labelled A1 to A3 (Fig. 3).

Fig. 3. Coordination environments of A + (A = Rb, K and Na), Cu2+ and S6+ cations in the crystal structures of RbNaCu(SO4)2 (a) and RbKCu(SO4)2 (b). General projections of the crystal structure of RbNaCu(SO4)2 (c) and RbKCu(SO4)2 (d) along the c axis.
The Cu2+ cation features four Cu–Oeq short bonds with distances of ~2 Å forming a nearly square-planar coordination. The lengths of the fifth (longer) Cu–Oap differ markedly: 2.236(2) Å in RbNaCu(SO4)2 versus 2.547(3) Å in RbKCu(SO4)2. The effect of the alkali cation size on the Cu2+ coordination has been observed before in layered copper hydrogen selenite halides (Charkin et al., Reference Charkin, Markovski, Siidra, Nekrasova and Grishaev2019). The CuO5 tetragonal pyramids are complemented by a sixth long and relatively weak bond of 2.604(2) Å and 2.713(3) Å for RbNaCu(SO4)2 and RbKCu(SO4)2, respectively. Thus, the coordination of the Cu2+ cation can also be described as [4+1+1].
In contrast to KNaCu(SO4)2 (Borisov et al., Reference Borisov, Siidra, Kovrugin, Golov, Depmeier, Nazarchuk and Holzheid2021), the cation ordering in the rubidium analogue is less perfect. Only the A1 site in the sodium compound is occupied exclusively by Rb+ while all the other alkali sites demonstrate mixed occupancies as:


The A2 and A3 sites in RbNaCu(SO4)2 contain only a minor amount of Rb+ admixture. The coordination environments for these sites are typical for Na+ cations (distorted octahedra, Fig. 3), while a CN = 11 for the A1 site is typical for Rb+. In RbKCu(SO4)2, the A2 and A3 sites show coordination numbers (CN = 10 for both sites) in accordance with the larger radius of K+. In this case, cation disorder is more pronounced which agrees with the smaller relative differences in cation size.
The S atoms are central to approximately regular SO4 tetrahedra. Akin to Rb2Cu(SO4)2, the sulfate moieties share one of the edges with CuO6 octahedra (Cu–S2 = 2.8315(8) Å and 2.8756(11) Å for RbNaCu(SO4)2 and RbKCu(SO4)2, respectively) (Fig. 3), despite unfavourable repulsive interactions between Cu2+ and S6+.
RbNaCu(SO4)2 and RbKCu(SO4)2 are formally isostructural to the recently reported KNaCu(SO4)2 (Borisov et al., Reference Borisov, Siidra, Kovrugin, Golov, Depmeier, Nazarchuk and Holzheid2021) and K2Cu(SO4)2 (Zhou et al., Reference Zhou, Liu, Ang and Zhang2020), although some differences in the cation coordinations are clearly visible. The SO4 tetrahedra and the CuO6 polyhedra form the porous [Cu(SO4)2]2– framework with two types of channels parallel to the c axis. The larger (elliptical) channels are occupied preferably by larger Rb+ (A1 sites), whereas the smaller ones are filled mostly by Na+ (in RbNaCu(SO4)2) or K+ (in RbKCu(SO4)2). Note the complete size-based ordering of alkali cations in KNaCu(SO4)2 (Borisov et al., Reference Borisov, Siidra, Kovrugin, Golov, Depmeier, Nazarchuk and Holzheid2021), which is somewhat less pronounced in its Rb-based analogues.
Rb2Cu2(SO4)3
The crystal structure of another new anhydrous sulfate, Rb2Cu2(SO4)3 with langbeinite-type A +2M 2+2(SO4)3 stoichiometry (Table 1) contains two symmetrically independent Rb sites, two Cu sites and three S atoms (Fig. 4).

Fig. 4. Coordination environments of Rb+, Cu2+ and S6+ cations (top) in the crystal structure of Rb2Cu2(SO4)3. [Cu2(SO4)3]2– ribbon (only short and strong Cu–O bonds are shown) (bottom left) and general projection of the crystal structure of Rb2Cu2(SO4)3 along the a axis (bottom right).
In Rb2Cu2(SO4)3, Rb+ and S6+ cations have typical coordination environments, similar to the aforementioned, whereas the coordination of the Cu2+ cations demonstrate interesting features worthy of discussion. The Cu1 atom has four short and strong Cu–Oeq bonds thus forming CuO4 squares. However, it has three additional very long Cu1–O5 = 2.864(12), Cu1–O1 = 2.925(15) Å and Cu1–O10 = 2.981(15) Å bonds, thus forming CuO7 [4+3] coordination environments. These Cu–O bonds have bond-valence contributions of 0.04–0.03 valence units and thus should be taken into consideration. The Cu2 atom has CuO6 [4+1+1] coordination environments, as in Rb2Cu(SO4)2 described above. Both the CuO6 and CuO7 polyhedra demonstrate bidentate bridging with SO4 tetrahedra.
Rb2Cu2(SO4)3 is isostructural to K2Cu2(SO4)3 described by Lander et al. (Reference Lander, Rousse, Batuk, Colin, Dalla Corte and Tarascon2017). The Cu-centred polyhedra and SO4 tetrahedra form [Cu2(SO4)3]2– ribbons depicted in Fig. 4. The arrangement of sulfate tetrahedra and Cu-centred polyhedra was originally described by Lander et al. (Reference Lander, Rousse, Batuk, Colin, Dalla Corte and Tarascon2017) as a herringbone pattern.
Rb2Cu2(SO4)3(H2O) ‘hydrolangbeinite’
The dominant motif of the novel Rb2Cu2(SO4)3(H2O) structure are layers formed by the corner-sharing and edge-sharing between sulfate tetrahedra and Cu–O polyhedra (Fig. 5). The [Cu2(SO4)3(H2O)]2– layers are parallel to the ab plane. In general, a layered character is typical for hydrated copper oxysalt structures. In Rb2Cu2(SO4)3(H2O), the layers are linked together by Rb1 and Rb2 atoms coordinated by eight and ten oxygen atoms, respectively. Note that water molecules are bonded exclusively to Rb1 atoms. The Cu1 site shows a typical coordination with the formation of CuO5 tetragonal pyramids with an almost planar CuO4 base. The Cu2-centred CuO6 octahedron demonstrates an exceptionally strongly distorted character. Cu2–O5 and Cu2–O6 bonds are strongly inclined (Fig. 5) relative to the equatorial plane of octahedron. This distortion is again due to the edge-sharing through O5–O6 with the S2O4 tetrahedron. The topology of the [Cu2(SO4)3(H2O)]2– layer is somewhat similar to that one for the [Cu2(SO4)3]2– ribbons in anhydrous Rb2Cu2(SO4)3 (Fig. 4). The rearrangement of sulfate groups in Rb2Cu2(SO4)3(H2O) is provoked by the hydration of one of the Cu-centred polyhedra. The vertex occupied by the water molecule is unshared and projected into the interlayer space.

Fig. 5. Coordination environments of Rb+, Cu2+ and S6+ cations (top) in the crystal structure of Rb2Cu2(SO4)3(H2O). [Cu2(SO4)3(H2O)]2– layer (only short and strong Cu–O bonds are shown) (bottom left) and general projection of the crystal structure of Rb2Cu2(SO4)3(H2O) along the b axis (bottom right).
Rb2Cu(SO4)Cl2
The coordination environments of atoms in monoclinic (C2/c) Rb2Cu(SO4)Cl2 are very similar to those described earlier in its natural K-analogue, chlorothionite, K2Cu(SO4)Cl2 (Giacovazzo et al., Reference Giacovazzo, Scandale and Scordari1976) crystallising in the orthorhombic space group Pnma. The Cu atom shows mixed-ligand coordination environments with oxygen and chlorine atoms, thus forming CuO2Cl2 coordination environments complemented by the two very long Cu–Cl bonds of 3.2020(8) Å (Fig. 6). These Cu–Cl bonds are much shorter in chlorothionite (3.0467(2) Å). The SO4 tetrahedra in Rb2Cu(SO4)Cl2 and chlorothionite show very similar S–O bond length values. Two symmetrically independent Rb atoms have strongly dissimilar coordination environments: Rb1O4Cl4 and Rb2O4. Rb2 has two Rb–Cl distances of 3.6652(10) Å and two of 3.6908(9) Å which are beyond the limit of 3.55 Å for the consideration of bonds. Also note the unusually low coordination number of K in chlorothionite.

Fig. 6. Coordination environments of Rb+, Cu2+ and S6+ cations (top) in the crystal structure of Rb2Cu(SO4)Cl2. General projection of the crystal structure of Rb2Cu(SO4)Cl2 along the a axis (bottom). Weak and long Cu–Cl bonds are not shown for clarity.
In Rb2Cu(SO4)Cl2, the main structural feature of edge-sharing between sulfate tetrahedra and CuO2Cl4 octahedra remains intact. The Cu–S1 distances are 2.5785(10) Å and 2.593(1) Å in Rb2Cu(SO4)Cl2 and chlorothionite, respectively.
The observed symmetry lowering in Rb2Cu(SO4)Cl2 compared to K2Cu(SO4)Cl2 is probably due to the replacement of K+ by the larger Rb+. This also causes a significant elongation of Cu–Cl bonds in the CuO4Cl2 octahedra.
Structures with additional O2– anions
Rb4Cu4O2(SO4)4·(Cu+0.83Rb0.17Cl), rubidium analogue of piypite
Piypite, K4Cu4O2(SO4)4⋅(Na,Cu)Cl, was described from the fumaroles of the Second scoria cone by Vergasova et al. (Reference Vergasova, Filatov, Serafimova and Stalova1984). The crystal structure was solved (R 1 = 0.035) using a sample of ‘caratiite’ from Mount Vesuvius, Naples, Italy, by Effenberger and Zemann (Reference Effenberger and Zemann1984). It belongs to the tetragonal symmetry, I4, with a = 13.60(2) Å, c = 4.98(1) Å and V = 921.1 Å3. Later, Kahlenberg et al. (Reference Kahlenberg, Piotrowski and Giester2000) solved the structure (R 1 = 0.028) of its synthetic sodium analogue, Na4Cu4O2(SO4)4⋅(MCl) (M = Na, Cu, □) in a supercell with P4/n, a = 18.451(1), c = 4.9520(2) and V = 1685.86 Å3.
The new compound Rb4Cu4O2(SO4)4⋅(Cu0.83Rb0.17Cl) crystallises analogous to the potassium compound in space group I4, a = 14.171(14), c = 4.991(5), V = 1002(2) Å3 and R 1 = 0.043. Proceeding from K to Rb, the value of the a parameter is affected most. We observed no indication of the supercell suggested by Kahlenberg et al. (Reference Kahlenberg, Piotrowski and Giester2000). In the structure of Rb4Cu4O2(SO4)4⋅(Cu0.83Rb0.17Cl), chlorine is disordered over two Cl1A (site occupation factor (s.o.f.) = 0.44(7)) and Cl1B (s.o.f. = 0.55(7)) sites with Cl1A–Cl1B = 0.82(2) Å. All of the atoms except ClA were refined anisotropically.
The structure of Rb4Cu4O2(SO4)4⋅(Cu0.83Rb0.17Cl) (Fig. 7) contains one symmetrically unique Rb position, one Cu, one S, and one mixed metal site M, occupied by Cu+ and Rb+ cations in a ratio indicated above. The Rb+ cation is coordinated by seven O atoms and two Cl atoms. The Cu site is coordinated by four oxygens forming a distorted CuO4 square complemented by one apical O2– anion at a distance of 2.395(8) Å and another one at 3.086(11) Å. As a result, an essentially elongated CuO6 octahedron is formed.
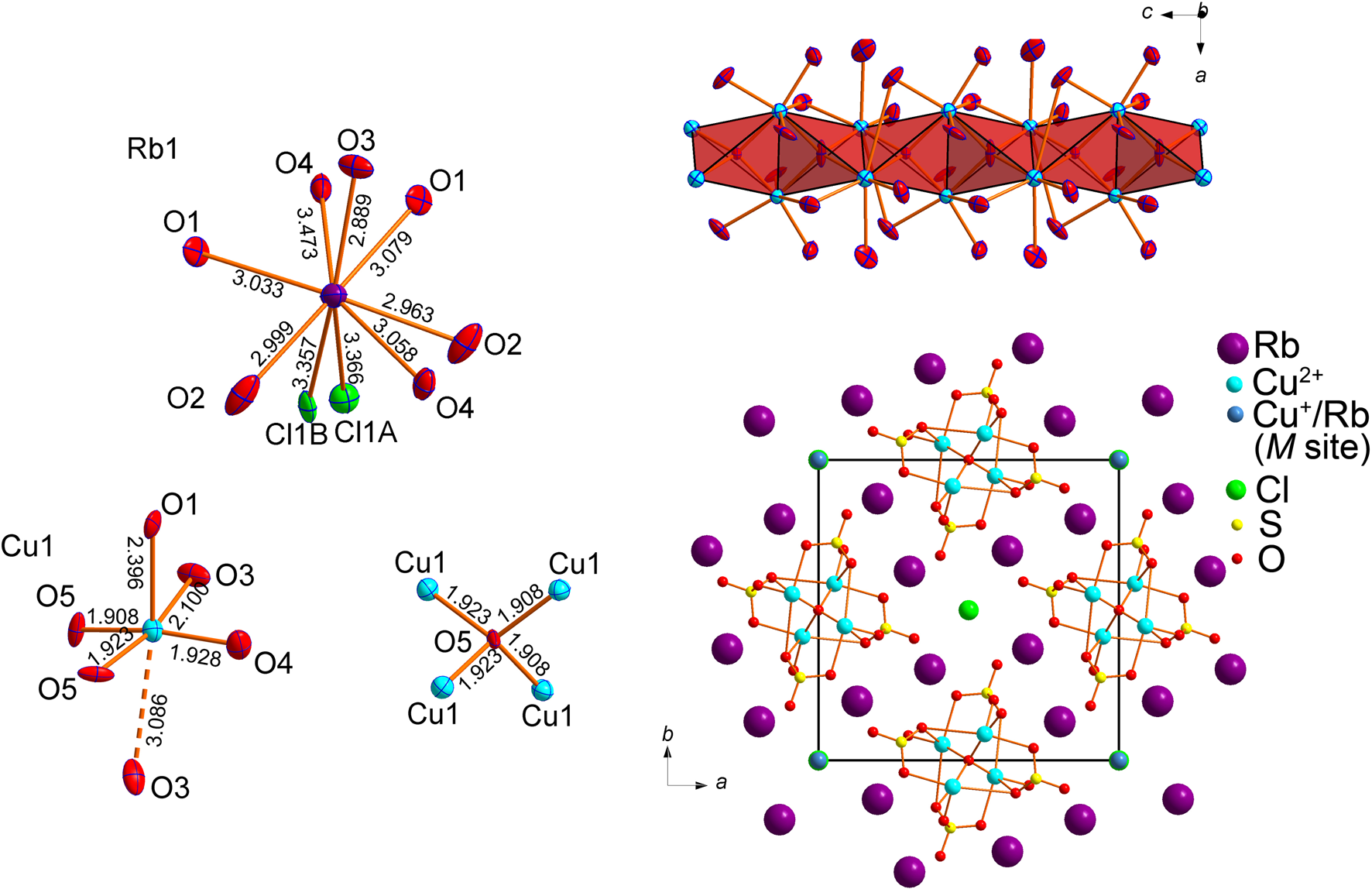
Fig. 7. Coordination environments of Rb+, Cu2+ cations and additional O2– anions in the crystal structure of Rb4Cu4O2(SO4)4⋅(Cu0.83Rb0.17Cl) (left). Polyhedral representation of the oxocentred [Cu2O]2+ chains surrounded by the oxygen atoms of sulfate groups (top right). General projection of the crystal structure of Rb4Cu4O2(SO4)4⋅(Cu0.83Rb0.17Cl) along the с axis (bottom right) (Rb–Cl, M–Cl and M–O bonds are omitted for clarity).
The mixed M site has a refined occupancy of Cu+0.83(7)Rb0.17(7). There are two strong bonds to the Cl atoms (M–Cl1A = 2.08(5) Å and M–Cl1B = 2.09(3) Å) which correspond to a typical linear Cu+Cl2– anion. There are also four equivalent M–O2 distances of 2.900(9) Å that correspond to Rb–O bonds.
The O1, O2, O3 and O4 atoms constitute the tetrahedral SO42– groups and are further designated as Ot. The ‘additional’ O5 oxygen atom is bonded exclusively to the Cu2+ cations (Fig. 7) and is designated as Oa. The Cu2+−Oa distances vary in the range of 1.908(15)–1.922(15) Å, whereas Cu2+−Ot ranges from 1.908(15) to 3.086(11) Å. Because of the higher strength of the Cu−Oa bonds compared to the weaker Cu−Ot bonds, one can describe the copper–oxide substructure of Rb4Cu4O2(SO4)4⋅(Cu0.83Rb0.17Cl) in terms of oxocentred OCu2+4 tetrahedra.
These tetrahedra link together via common edges to form [Cu2O]2+ single chains (Fig. 7). In such units, each tetrahedron shares two of six edges with adjacent tetrahedra. The [Cu2O]2+ single chains extend along the c axis. The chains are surrounded by SO4 tetrahedra in a rod-like arrangement. Rb+ cations fill the space in between the rods. In turn, the channels filled by CuCl2– linear complexes are formed between the rubidium atoms. Formation of ‘guest’ metal-chloride species with Cu+ cations in the channels is characteristic for fumarolic minerals: averievite, Cu5O2(VO4)⋅nMClx (M = Cu, Cs, Rb and K) (Vergasova et al., Reference Vergasova, Starova, Filatov and Anan'ev1998; Kornyakov et al., Reference Kornyakov, Vladimirova, Siidra and Krivovichev2021) and aleutite, Cu5O2(AsO4)(VO4)⋅(Cu0.5□0.5)Cl (Siidra et al., Reference Siidra, Nazarchuk, Agakhanov and Polekhovsky2019b). A similar phenomenon was observed in the family of copper-lead selenite bromides (Siidra et al. Reference Siidra, Kozin, Depmeier, Kayukov and Kovrugin2018b): the oxocentred OCu2+nPb4–n tetrahedra were present only in the structures containing both Cu2+ and Cu+. Whenever Cu2+ was the sole copper species, the anionic sublattice was formed only by SeO32– and Br– with no ‘extra’ O2– present. A possible explanation, which evidently needs to be further verified, is that the Cu+ forms strong covalent bonds to the halide anions. The terminal atoms of the strong halo- and/or oxoanions form weaker bonds to the dications (Pb2+ or Cu2+), and the ‘extra’ O2– anions are one of the ways to complete their bond-valence sums to saturation.
Rb2Cu5O(SO4)5, rubidium analogue of cryptochalcite and cesiodymite
Rb2Cu5O(SO4)5 is isostructural to the recently described cryptochalcite, K2Cu5O(SO4)5, and cesiodymite, CsKCu5O(SO4)5 (Pekov et al., Reference Pekov, Zubkova, Agakhanov, Pushcharovsky, Yapaskurt, Belakovskiy, Vigasina, Sidorov and Britvin2018b). Note that both these fumarolic minerals contain significant amounts of Rb (up to 1.95 wt.% Rb2O). The new compound Rb2Cu5O(SO4)5 is the first synthetic representative of this structure type. The initial model started from the atomic coordinates provided by Pekov et al. (Reference Pekov, Zubkova, Agakhanov, Pushcharovsky, Yapaskurt, Belakovskiy, Vigasina, Sidorov and Britvin2018b). The unit-cell volume of Rb2Cu5O(SO4)5 (V = 1770.9(3) Å3) is nearly halfway between those of cryptochalcite (V = 1751.73(10) Å3) and cesiodymite (V = 1797.52(16) Å3). The structure of cryptochalcite-type compounds, and Rb2Cu5O(SO4)5 in particular, represents an interesting example of the framework formed by both CuOn polyhedra and oxocentred OCu4 tetrahedra. The [Cu5O(SO4)5]2– open framework, with Rb+ in the channels, can be described as consisting of two types of alternating blocks, A and B (Fig. 8). The A-blocks (in the middle) are formed by O2Cu6 dimeric units and sulfate tetrahedra, whereas B-blocks (right) are formed by CuO5 tetragonal pyramids and sulfate tetrahedra.

Fig. 8. General projection of the crystal structure of Rb2Cu5O(SO4)5 along the a axis. The [Cu5O(SO4)5]2– open framework can be described as consisting of two types of blocks, A and B. A-blocks (centre) are formed by O2Cu6 dimeric units and sulfate tetrahedra, whereas B-blocks (right) are formed by CuO5 tetragonal pyramids and sulfate tetrahedra. Rb–O bonds are omitted for clarity.
Discussion and concluding remarks
The compounds reported for the first time and the determination of their crystal structures significantly expand the family of anhydrous copper sulfates. Some of the obtained anhydrous rubidium copper sulfates turned out to be isostructural to known compounds and minerals. An example of this is Rb2Cu5O(SO4)5, which is isostructural to cesiodymite, CsKCu5O(SO4)5 and cryptochalcite, K2Cu5O(SO4)5 (Table 2). This fact, as well as the high contents of rubidium in natural samples, shows that the discovery of a mineral species with an alkali position, occupied essentially or even predominantly by rubidium, is highly probable. Our synthesis of a number of mineral analogues also demonstrates the possibility of introducing rubidium cations into the already known structural architectures. A slight symmetry lowering, with most structural features left intact, is observed for the Rb-analogue of chlorothionite.
Table 2. Crystallographic parameters of known cryptochalcite-type A 2[Cu5O(SO4)5] compounds.

In addition, the minerals and synthetic framework compounds of the A 2Cu(SO4)2 series demonstrate a vivid example of morphotropism with the formation of structural types depending on the size of the cations residing in the cavities of the [Cu(SO4)2]2– open framework. To date, five types can be distinguished (Table 3). We propose to call this series of compounds ‘saranchinaite-type’, as the stoichiometry A 2Cu(SO4)2 was first encountered during the discovery and description of saranchinaite Na2Cu(SO4)2 (Siidra et al., Reference Siidra, Lukina, Nazarchuk, Depmeier, Bubnova, Agakhanov, Avdontseva, Filatov and Kovrugin2018c) and shortly thereafter its synthetic analogue (Kovrugin et al., Reference Kovrugin, Nekrasova, Siidra, Mentré, Masquelier, Stefanovich and Colmont2019). Saranchinaite forms a framework with a unique topology (further denoted as type α) that has not been identified in other anhydrous copper and alkali metal sulfates. Note the presence of only a minor admixture of potassium in one of the positions in the mineral (Siidra et al. Reference Siidra, Lukina, Nazarchuk, Depmeier, Bubnova, Agakhanov, Avdontseva, Filatov and Kovrugin2018c). K(Na,K)Na2[Cu2(SO4)4] (Siidra et al., Reference Siidra, Charkin, Kovrugin and Borisov2021b) crystallises in a completely different structure type (β). The compound Rb2Cu(SO4)2 described in this work also forms a new structure type, which we term δ. The recently described family of compounds KMCu(SO4)2 (M = Na, K) (Borisov et al., Reference Borisov, Siidra, Kovrugin, Golov, Depmeier, Nazarchuk and Holzheid2021; Zhou et al., Reference Zhou, Liu, Ang and Zhang2020) is substantially supplemented by two rubidium representatives, RbNaCu(SO4)2 and RbKCu(SO4)2. Attempts to obtain a solely sodium-bearing example of this structural type (γ) were unsuccessful. Sodium analogues of anhydrous sulfates discussed herein, except of chlorothionite, represent their own structure types. However, euchlorine-type A 2Cu3O(SO4)3 (A 2 = Na2, NaK and K2) compounds (Nekrasova et al., Reference Nekrasova, Tsirlin, Colmont, Siidra, Vezin and Mentré2020) and minerals (Vergasova et al., Reference Vergasova, Starova, Filatov and Anan'ev1988; Siidra et al., Reference Siidra, Nazarchuk, Zaitsev, Lukina, Avdontseva, Vergasova, Vlasenko, Filatov, Turner and Karpov2017, Reference Siidra, Borisov, Lukina, Depmeier, Platonova, Colmont and Nekrasova2019a) crystallise in the same structure type. Framework compounds such as e.g. saranchinaite-type and cryptochalcite-type sulfates may show different architectures depending on the size of the alkali metal, whereas e.g. euchlorine- and chlorothionite-type sulfates having layered and chain structures are more flexible and can include alkali metals of quite different ionic radii.
Table 3. Crystallographic parameters of known saranchinaite-type A 2[Cu(SO4)2] compounds.

Note that the structural architectures of anhydrous sulfates do not relate exclusively to framework ones. Thus, piypite and its synthetic analogues are based on chain copper oxide–sulfate complexes; chlorothionite and its polymorphs are also based on chain complexes formed via weak Cu–Cl bonds. The discovery of a new Rb2Cu(SO4)Cl2 monoclinic polymorph of chlorothionite (Table 4) seems to be of particular interest considering the recently discovered interesting magnetic properties of synthetic K2Cu(SO4)X 2 (X = Cl, Br) (Soldatov et al., Reference Soldatov, Smirnov, Povarov, Hälg, Lorenz and Zheludev2018; Bo et al., Reference Bo, Wang, Wan and Wan2020) and Na2Cu(SO4)Cl2 (Fujihala et al., Reference Fujihala, Mitsuda, Mole, Yu, Watanabe, Yano, Kuwai, Sagayama, Kouchi, Kamebuchi and Tadokoro2020). Note that both sodium and potassium analogues are strictly isostructural. An interesting pattern with a change in symmetry depending on the size of the alkaline cation is observed in the structures of piypite and its synthetic analogues (Table 5). Rb4Cu4O2(SO4)4⋅(Cu+0.83Rb0.17Cl) and K4Cu4O2(SO4)4⋅(Na,Cu)Cl crystallise in I4 space group, while Na4Cu4O2(SO4)4⋅(MCl) (M = Na, Cu and □) crystallises in P4/n. It can be seen that filling the channels with metal chloride (MeCl) complexes shows significant variations. It should be noted that the structural features of piypite from the fumaroles of Tolbachik volcano have not been studied to date. Effenberger and Zemann (Reference Effenberger and Zemann1984) explicitly state in their article that “the nature of the Me atoms is not completely clear”.
Table 4. Crystallographic parameters of known chlorothionite-type A 2Cu(SO4)X 2 compounds.

Table 5. Crystallographic parameters of known piypite-type Rb4[Cu4O2(SO4)4]⋅(MCl) compounds.

Rb2Cu2(SO4)3 is a further example of crystallisation in the already known structure type first observed for K2Cu2(SO4)3 (Lander et al., Reference Lander, Rousse, Batuk, Colin, Dalla Corte and Tarascon2017). In Rb2Cu2(SO4)3, the unit-cell parameters increase significantly in accordance with an increase in the ionic radius of the alkali cation. The stoichiometry of the A 2M 2+2(SO4)3 compounds corresponds to one of the most common potassium and magnesium sulfates – langbeinite, K2Mg2(SO4)3, which crystallises in the cubic space group P213. In the fumaroles of the scoria cones of the Tolbachik volcano, langbeinite is also one of the most common anhydrous sulfates. Langbeinite-type compounds show very wide variations in isomorphic substitutions (Gattow and Zemann, Reference Gattow and Zemann1958). The main factor for the formation of the A 2Cu2(SO4)3 orthorhombic structure is the presence of Jahn–Teller copper cations. In the same series of syntheses, during which Rb2Cu2(SO4)3 was obtained, ‘hydrolangbeinite’ Rb2Cu2(SO4)3(H2O) was found, formed as a result of a minor hydration of the initial mixture of reagents. Hydrated langbeinites are currently unknown for any composition of minerals or synthetic compounds. The new compound obtained shows the probability of detecting such compounds (especially for copper-containing ones) in the zones of fumaroles exposed to intense atmospheric precipitation. Alkali copper sulfates are known to form multiple hydrated ‘offsprings’ during exposure to moist air (Siidra et al., Reference Siidra, Borisov, Charkin, Depmeier and Platonova2021a).
In the determined new structural architectures, a number of features were revealed which were seldom observed before. The first is the bidentate coordination of the sulfate tetrahedra via edge- sharing to the Cu2+-centred coordination polyhedron. Until recently, such coordination was known only for the chlorothionite structure. The second is formation of ‘high-coordinated’ Cu atoms forming CuO7 polyhedra. We considered such a coordination when describing the crystal structure of saranchinaite (Siidra et al., Reference Siidra, Lukina, Nazarchuk, Depmeier, Bubnova, Agakhanov, Avdontseva, Filatov and Kovrugin2018c). The structures of the new compounds suggest that such coordination is not in fact so uncommon, at least among anhydrous alkali copper sulfates. All of the described features clearly indicate the importance of further systematic studies of anhydrous copper-sulfate systems. Their exploration, particularly of the new copper-oxide substructures with new coordination environments, is highly likely to lead to new potentially interesting magnetic properties due to unusual arrangements of magnetically active Cu2+ cations. The effect of the alkali cation size on the Cu2+ coordination has been demonstrated recently for the family of synthetic hydrogen selenites (Charkin et al., Reference Charkin, Markovski, Siidra, Nekrasova and Grishaev2019); one can expect that it will also be pronounced in other families, including sulfates.
The crystallographic parameters listed in Tables 2–5, show that anhydrous sulfates are a fruitful playground for the synthesis of new anhydrous compounds known previously only as prototype-structures in minerals. Conversely, it is highly probable that compounds, obtained so far only as synthetics, may exist as minerals in high-temperature fumaroles with strongly oxidising conditions and in sublimates of natural coal fires (e.g. Pautov et al., Reference Pautov, Mirakov, Siidra, Faiziev, Nazarchuk, Karpenko and Makhmadsharif2020).
It is worth noting here that synthetic analogues of various minerals, including sulfates, have been obtained using various synthetic approaches, which do not necessarily mimic the natural processes (or, more exactly, the currently assumed formation mechanisms). At present, condensation from a vapour phase or interaction of hot gases with basaltic scoria are considered to be the most likely formation mechanisms of fumarolic minerals. Yet, our studies clearly indicate that vapour transport in water-free systems can be ignored at least until 600°C wherein the only volatile species are copper chlorides. At least some of the compounds described herein have most probably crystallised from melts. Several test experiments have shown that at least some Rb-containing compositions melt at temperatures as low as 400–450°C; moreover, good-quality crystals and homogeneous samples were not produced even after long-time subsolidus heat treatments. Additional experiments are clearly necessary to elucidate the role of reaction kinetics in solid-state processes. The other synthetic approach, applied successfully to preparation of analogues of chlorothionite, is crystallisation from hot aqueous solutions. However, one can expect competitive formation of less soluble double sulfates, e.g. Tutton salts, or hydrolysis products, e.g. analogues of natrochalcite. Overall, the chemistry of hydrates with low water content remains in many respects a terra incognita. A possible solution lies in exploiting media with low chemical potential of water; our current example is the Rb2Cu2(SO4)3(H2O), formed under low vapour pressure (given the sealed tube had survived internal pressure upon annealing). A test experiment with the initially wet sulfate charge annealed in a temperature gradient between ambient and 650°C produced good-quality single crystals of various known hydrates including CuSO4⋅5H2O in the relatively cold part of the sealed silica tube. Therefore, this approach is promising in producing various hydrates scarcely accessible in any other way. The use of moist non-aqueous solvents seems not to be straightforward as they either do not dissolve most inorganic sulfates (alcohols) or coordinate competitively to copper (acetonitrile). The process involving water evidently also takes place under natural conditions, e.g. upon hydration–dehydration cycles. Yet, our studies of such processes for selected copper sulfate minerals indicate that these are very complicated (Siidra et al., Reference Siidra, Borisov, Charkin, Depmeier and Platonova2021a), include numerous steps and are not always reversible even for initially single-phase specimens. It is however very likely that these studies, extended into more complex systems, will produce even more intricate and intriguing architectures, compositions and possibly properties. Some of these studies are now in progress.
Acknowledgements
We are grateful to Uwe Kolitsch, Gerald Giester and Peter Leverett for many valuable comments. Technical support by the SPbSU X-ray Diffraction Resource Centre is gratefully acknowledged.
Supplementary material
To view supplementary material for this article, please visit https://doi.org/10.1180/mgm.2021.73