Introduction
Anthropogenic activity has caused rapid deterioration of the environmental situation throughout the world. One of the main problems is air pollution. In most industrialized countries, special measures are applied to reduce atmospheric pollution, aimed at developing ways to reduce the formation of harmful substances and methods for cleaning gases from already formed products.
There are several methods (sorption, catalytic, carbamide, ammonium-magnesite, etc.) and technologies (technology for removing sulfur dioxide from flue gases, Ebara technology) that make it possible to clean the flue gases from harmful components. The effectiveness of these measures is quite high; however, their significant disadvantage is the high cost of equipment and operation, as well as the complexity of technological processes. In addition, all the equipment is designed for high performance. It should be remembered that small and medium energy enterprises significantly deteriorate the ecological situation, which in turn requires the use of compact and inexpensive technological solutions for gas cleaning.
The technology of Ebara Corporation based on the plasma-chemical conversion of flue gases with ammonia has proven successful (Hirota et al., Reference Hirota, Hakoda, Taguchi, Takigami, Kim and Kojima2003; Chmielewski et al., Reference Chmielewski, Licki, Pawelec, Tyminski and Zimek2004; Ighigeanu et al., Reference Ighigeanu, Martin, Calinescu, Zissulescu, Oproiu, Manaila and Craciun2006; Ighigeanu et al., Reference Ighigeanu, Calinescu, Martin, Matei, Bulearca and Ighigeanu2009; Kikuchi and Pelovski, Reference Kikuchi and Pelovski2009). In the process of a plasma-chemical reaction initiated by a continuous electron beam, solid compounds are formed which are removed by electrostatic filtration. Ebara Corporation technologies are currently used in Indianapolis, USA, and Karlsruhe, Germany. In Indianapolis, two accelerators are used (0.8 MeV and 160 kW each) for gas cleaning (1000 ppm SO2 and 400 ppm NOx) at a gas flow power of 1.6–3.2 × 104 m3/h. Karlsruhe uses two accelerators (0.3 MeV and 180 kW each) for gas cleaning (50–500 ppm SO2 and 300–500 ppm NOx) at a gas flow rate of 1–2 × 104 m3/h. In the technology of Ebara Corporation, linear continuous accelerators of high cost, dimensions, and costs for protecting personnel from ionizing radiation are used.
An alternative may be the organization of plasma-chemical binding of the harmful components of flue gases, initiating the process with a pulsed electron beam (Mizuno et al., Reference Mizuno, Clements and Davis1984; Davis and Mizuno, Reference Davis and Mizuno1986; Denisov et al., Reference Denisov, Kuznetsov, Novoselov and Tkachenko1998, Reference Denisov, Kuznetsov, Novoselov and Tkachenko2001; Nakagawa et al., Reference Nakagawa, Mannami, Natsuno and Nishikata2002; Imada and Yatsui, Reference Imada and Yatsui2006).
The pulsed accelerators, especially those of the nanosecond range, made it possible to sharply reduce the dimensions of high-voltage generation circuits by increasing the dielectric strength under pulsed action. Therefore, the use of transient processes to form voltage can significantly reduce the overall dimensions of the equipment while maintaining the same level of accelerating voltage, as well as to develop power which can not be achieved for continuous accelerators (units of GW). An increase in the energy input rate in some cases enables the use of threshold effects that are impossible with a lower power, which ultimately can decrease specific energy consumption to achieve a similar cleaning effect. Nanosecond vacuum diodes are also much simpler in design and able to operate at a relatively low vacuum (up to 0.1 Pa fore vacuum).
The initiation of plasma-chemical processes by a pulsed electron beam is one of the actively developing methods for the activation of chemical processes. The use of pulsed electron beams ensures the formation of plasma with a high degree of nonequilibrium ion and electron temperatures, which is a significant advantage when used in various industrial processes. The industrial process with a new level of energy and resource efficiency is a modern trend that has scientific and economic feasibility. Reducing unproductive energy losses in heating of units, aggregates, binders by combining the reaction and plasma volumes will result in higher productivity and economic efficiency of production. The use of nonequilibrium, fast processes in plasma will significantly increase the speed of chemical processes and therefore reduce costs.
The well-known simplified model of chemical reactions during electron beam flue gas treatment is presented in Figure 1.
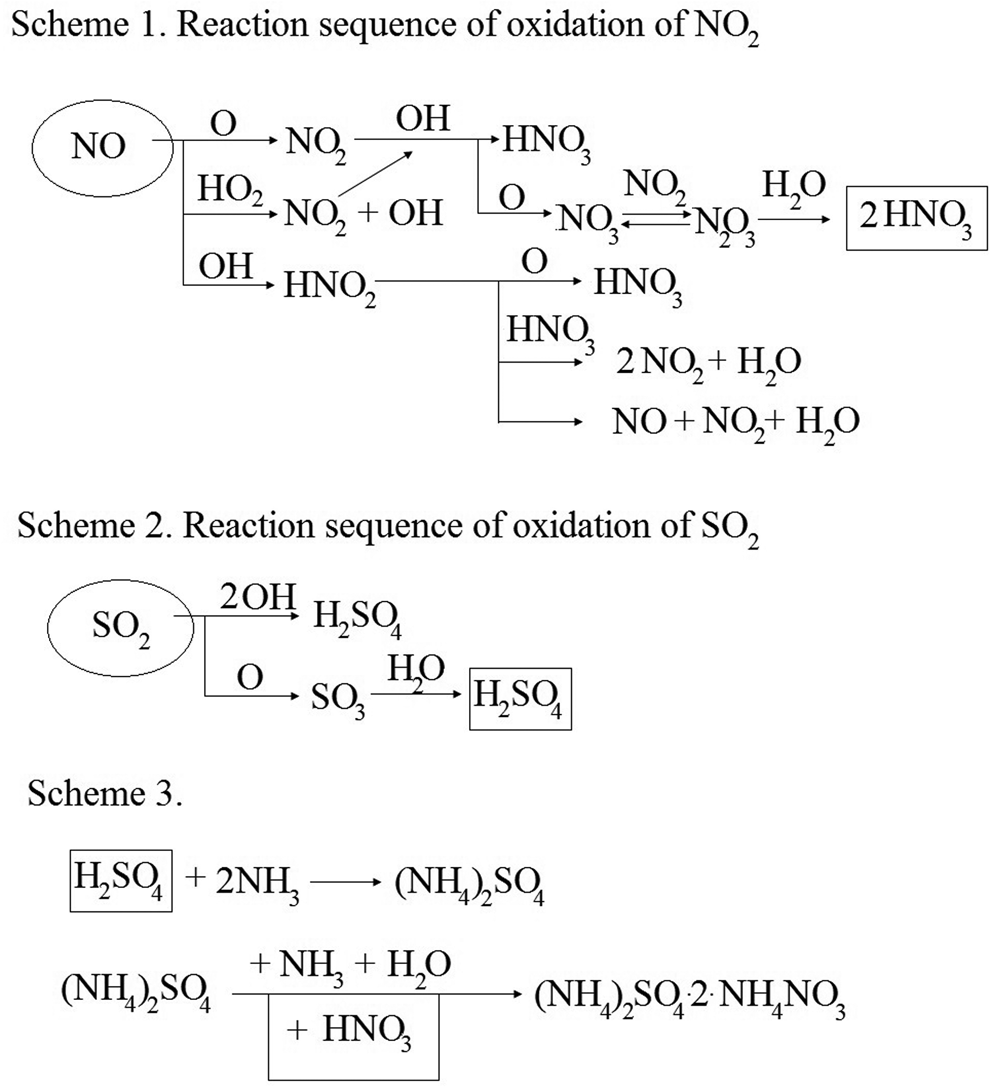
Fig. 1. A simplified model of chemical reactions during electron beam flue gas treatment (Ighigeanu et al., Reference Ighigeanu, Calinescu, Martin, Matei, Bulearca and Ighigeanu2009).
As can be seen from the scheme, the main reactive particles initiating the initial binding reactions of nitrogen and sulfur oxides are atomic oxygen and a hydroxyl radical.
Atomic oxygen is produced by breaking a chemical bond in an O2 molecule due to the action of an electron with an energy of more than 5.2 eV.
In the presence of water vapor, the generation of OH radicals occurs as follows:


If in the first case, the generation of OH radicals occurs as a result of the interaction of atomic oxygen with a water molecule and does not require additional energy, then in the second case, for the decay of a water molecule in the singlet-excited state, it is necessary to apply an energy of 8.4 eV.
In fact, for an efficient flue gas treatment, it is necessary to ensure conditions under which the primary electrons of the beam, as well as the secondary electrons formed as a result of the impact ionization process would provide the action on oxygen and water molecules uniformly and throughout the reactor volume with an energy sufficient for their dissociation.
However, it must be taken into account that the values of these energies can be significantly reduced due to (1) the transfer of the energy to the vibrational and rotational degrees of freedom of the molecules by more thermalized electrons and (2) excitation of the electron subsystem.
However, there is currently no data on the processes occurring in the interaction of pulsed electron beams with high-pressure gases, which are the main ones in technological processes. These are a mixture of gases at high pressure with a complex chemical composition.
Identifying the laws governing the propagation of pulsed electron beams and the charge dissipation of a pulsed electron beam in gas compositions, and determining the calculated ratios of the specific absorbed energy in the region of high pressures of gas compositions, are important tasks of pulsed plasma chemistry, since the productivity of plasma-chemical technologies is determined by the pressure in the reactor and its volume. A review of the literature on the interaction of pulsed beams of charged high-power particles with gas and condensed media has shown a large number of theoretical and experimental studies in gas media at low pressures (Miller et al., Reference Miller, Gerardo and Poukey1972; Wallis et al., Reference Wallis, Sauer, Sünder, Rosinskii, Rukhadze and Rukhlin1975; Artamonov et al., Reference Artamonov, Gorbunov, Kuksanov and Salimov1981; Erwin and Kunc, Reference Erwin and Kunc1988; Kuperman, Reference Kuperman2001; Leger et al., Reference Leger, Loiseau, Held, Eichwald, Defoort and Dupillier2006; Yousfi et al., Reference Yousfi, Leger, Loiseau, Held, Eichwald, Defoort and Dupillier2006; Anania et al., Reference Anania, Geer, de Loos, Reitsma and Jaroszynsk2008). Most experimental results on the propagation of electron beams have been currently obtained in axially symmetric drift spaces using metal and dielectric drift tubes with a diameter comparable to the transverse size of the electron beam (Wallis et al., Reference Wallis, Sauer, Sünder, Rosinskii, Rukhadze and Rukhlin1975; Erwin and Kunc, Reference Erwin and Kunc1988; Leger et al., Reference Leger, Loiseau, Held, Eichwald, Defoort and Dupillier2006). In addition, numerous experiments on the transport of electron beams in various gases [in the self-focusing mode at a distance L of the order of several “betatron lengths” (Miller et al., Reference Miller, Gerardo and Poukey1972; Artamonov et al., Reference Artamonov, Gorbunov, Kuksanov and Salimov1981)] show that the most efficient propagation of the electron beam occurs in the low-pressure region of p ~1 Torr. The use of pulsed electron beams for flue gas cleaning is limited by two factors that determine the parameters of the accelerators used: (1) to reduce the radiation load on the personnel and (2) optimal conditions for the initiation of a chemical reaction – first of all, homogeneous conditions throughout the reactor volume.
The aim of the current work to measure the average value of the specific absorbed energy in the presence of water vapor and soot. A mixture of gases such as nitrogen, carbon dioxide, and oxygen was chosen as the medium under study, since these types of gases are the main components of flue gases. Typical flue gas contents were given as follows: nitrogen 78–86%, carbon dioxide 8–15%, and oxygen 2–6% (Mizuno et al., Reference Mizuno, Clements and Davis1984; Davis and Mizuno, Reference Davis and Mizuno1986; Denisov et al., Reference Denisov, Kuznetsov, Novoselov and Tkachenko1998, Reference Denisov, Kuznetsov, Novoselov and Tkachenko2001; Nakagawa et al., Reference Nakagawa, Mannami, Natsuno and Nishikata2002; Imada and Yatsui, Reference Imada and Yatsui2006). In the experiments, the nitrogen content was 83%, carbon dioxide was 14%, oxygen was 2.6%. The selected mixture simulates the composition of the flue gas. Water vapor and ash are chosen as a condensed phase. These substances are either components of flue gases, or starting reagents or products of plasma-chemical reactions of the cleaning process using the pulsed electron beam.
Experimental setup
The studies were performed on a bench using a TEA-500 pulsed electron accelerator (Remnev et al., Reference Remnev, Furman, Pushkarev, Karpuzov, Kondrat'ev and Goncharov2004), a drift chamber, and a sectioned calorimeter with the total beam charge monitor function (Egorov et al., Reference Egorov, Serebrennikov, Isakova and Poloskov2007).
The TEA-500 pulse electron accelerator consists of the following main blocks: a gas-filled pulse voltage generator (assembled according to the Arkady-Marx scheme), a double forming line, an autotransformer, and a diode unit. The autotransformer in this design of the accelerator is its feature. An autotransformer is used to match a low-resistance water double forming line with a high-resistance impedance of an explosive-emission planar diode during the formation of a working pulse. A solid graphite cathode was used as a cathode, while the 140-μm-thick aluminum foil and a supporting lattice with an optical transparency of 70% were used as an anode.
In a sectioned calorimeter to control the charge of the beam, a Faraday cup and a calorimeter were used. Each section of the collector is thermally insulated and serves to determine the distribution of energy over the cross section of the electron beam. The heating of each section is measured using a separate non-contact temperature sensor that can automatically receive and process data. At the same time, each section of the collector is connected electrically with a low-inductance shunt of low resistance, forming the collector of a Faraday cup, which enables to estimate the total current and charge of the electron beam. Two independent methods for recording signals were used: the charge was recorded by a Faraday cup using an oscilloscope, while the energy was recorded using a computerized program. The error in measuring the total energy of the electron beam by a sectioned calorimeter does not exceed ±8%. The error in measuring the total charge of the beam is not more than 5% for the sectioned and integral Faraday cylinders. In addition, the design of a sectioned calorimeter provides for barriers that prevent a large accumulation of powder particles on the sections of the Faraday cup (aerodynamic barrier). During the operation of the sectioned calorimeter, the energy distribution of the electron beam in the cross section was estimated.
Figure 2 shows the experimental design.

Fig. 2. Experimental design. (1) A TEA-500 pulsed electron accelerator diode chamber, (2) a drift chamber, (3) a sectioned calorimeter with total beam charge monitor function, and (4) a fan.
The metal drift chamber had a length L = 46 cm, which made it possible to efficiently propagate a pulsed electron beam in the injection region. Based on the results of preliminary studies of plasma-chemical processes of flue gas purification by a pulsed electron beam, the following humidity values of 15 ± 5% and 50 ± 15% and the pressure of 750, 560, and 375 Torr in the drift chamber were selected.
The experimental mixtures with suspended particles were circulated by two fans mounted in a closed loop of the drift pipe. The air flow rate was measured using an anemometer (Egorov et al., Reference Egorov, Serebrennikov, Isakova and Poloskov2007). It was 0.35 m/s at a pressure of 375 Torr, 0.51 m/s at 560 Torr, and 0.6 m/s at 750 Torr. The amount of water vapor was estimated by measuring the humidity in the drift chamber using a multifunction meter (https://merapribor.ru/catalog/potok/raskhodomery-vozdukha-i-gazov-e-e-elektronik/izmeritel-omniport-30/). Humidity values of 15 ± 5% and 50 ± 15% and pressure in the drift chamber of 750, 560, and 375 Torr were chosen.
The electron beam was extracted through the anode window, an aluminum foil of 140 μm thick, supporting its lattice with optical transparency of 70%. The beam parameters were measured using a Rogowski coil and a capacitive voltage divider. The scatter of the current and voltage values recorded by the sensors did not exceed 5%. The parameters of the pulsed electron accelerator were accelerating voltage amplitude of 450 kV with a half-amplitude duration of 60 ns (Remnev et al., Reference Remnev, Furman, Pushkarev, Karpuzov, Kondrat'ev and Goncharov2004). The charge of the beam extracted beyond the anode foil was 440 μC. The electron beam was an energy flux with a diameter of 5 cm.
During the experiments, the drift chamber was filled with the medium under study, into which the electron beam was injected. The value of the charge and the energy distribution over the cross-section of the electron beam extracted for the anode foil were measured using a sectioned calorimeter with the total beam charge monitor function (Mizuno et al., Reference Mizuno, Clements and Davis1984). This calorimeter was installed inside the movable tube, which made it possible to measure at a variable distance from the accelerator output window along the entire length of the drift chamber.
Results and discussion
Study on the process of dissipation of charge and energy of a pulsed electron beam in a mixture of gases: nitrogen, carbon dioxide, and oxygen in the presence of water vapor
Figure 3 shows the characteristic thermal profile of the electron beam imprint obtained using a sectioned calorimeter with the total beam charge monitor function. For this imprint, the initial temperature before irradiation was 21°С. The sections were numbered in a clockwise spiral from the reference points indicated in the figure (white figures: 1, 2, 8, 20, and 38 in the figure). The effective diameter of the sectioned calorimeter was 114 mm.
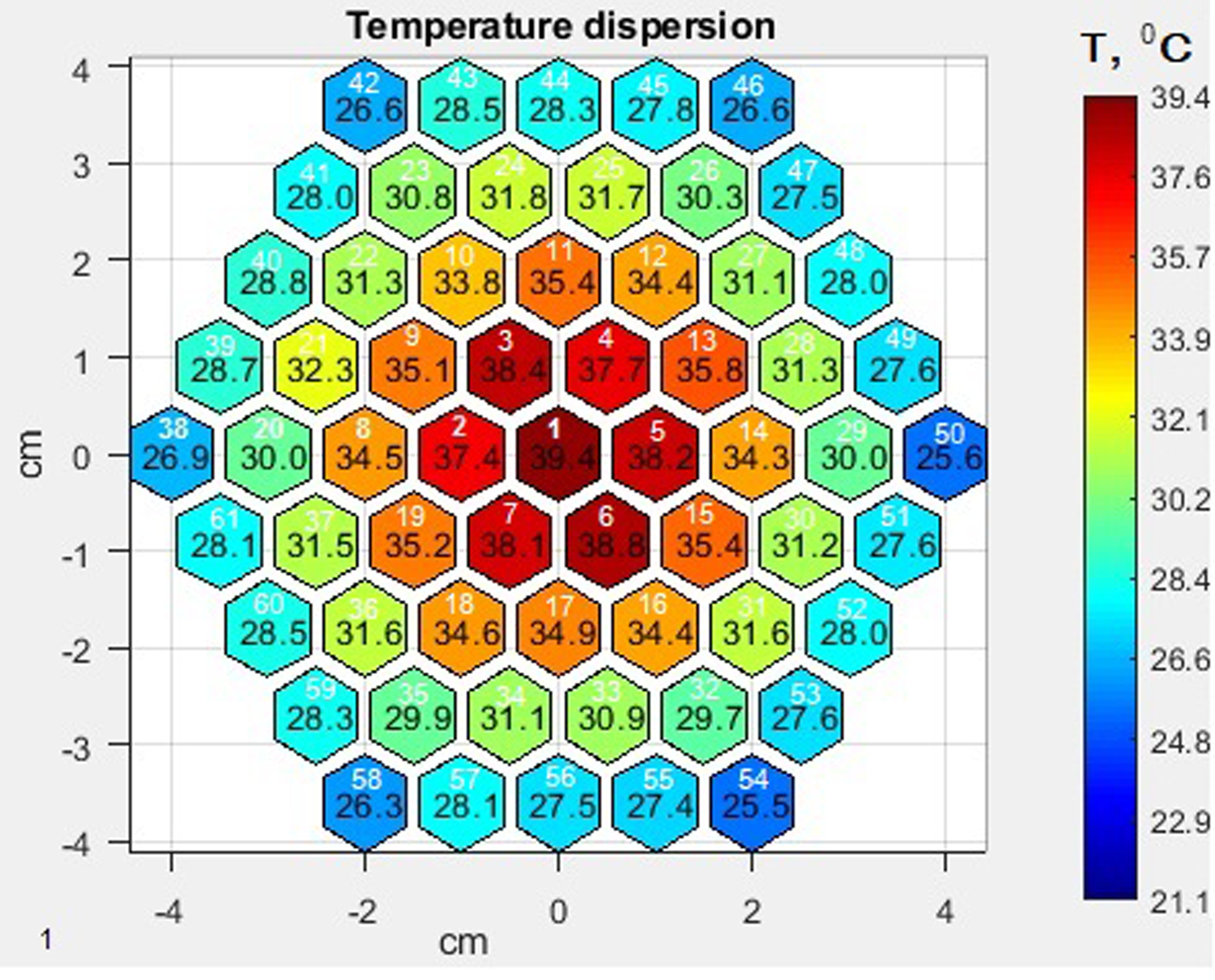
Fig. 3. A thermal profile of the electron beam after 10 pulses at a pulse repetition rate of 1 pps.
Figure 4 shows the energy received by each section of a sectioned collector for 10 irradiation pulses. The order of section numbers on the abscissa axis is based on the developed numbering (Fig. 3).

Fig. 4. Electron beam energy recorded by collector sections.
At total pressures of 750, 560, and 375 Torr and humidity of 15% in the drift chamber, the thermal profiles of the pulses of the electron beam, recorded at various distances from the anode foil when the beam passes through the gas mixture, are similar in the presence of water vapor. With the increasing distance from the anode foil, the thermal profile of the print of the pulsed electron beam has a more uniform distribution in the cross section.
Current and voltage oscillograms, and thermal profiles of the pulsed electron beam imprints, were recorded at distances of 6–46 cm (step 5 cm) from the accelerator exit window when the electron beam passed through the gas mixture in the presence of water vapor at humidity of 15 and 50% and the pressure of 750, 560, and 375 Torr in the drift pipe. Typical waveforms of current and voltage are shown in Figure 5.

Fig. 5. Typical current oscillograms (I RC) extracted from the anode foil, voltage (U) of the pulsed electron beam, and current of the pulsed electron beam reaching the collector of a Faraday cup (I FC).
Analyzing the data from the collector of the calorimeter and the oscillograms of the current (I RC) extracted from the anode foil and the currents of the pulsed electron beam reaching the collector of the Faraday cup, we constructed the dependence of the ratio of the charge of the pulsed electron beam reaching the collector of the Faraday cup (q FC) on the charge of the pulsed electron beam introduced into the drift chamber (q 0, the charge of electrons removed from the anode foil) and the dependence of the average energy density carried by a pulsed electron beam on the distance at a pressure of 750, 560, and 375 Torr (Figs 6–8).

Fig. 6. Dependence of the ratio of the charge of a pulsed electron beam (q FC) reaching the collector of a Faraday cup on the charge of a pulsed electron beam (q 0) introduced into the drift chamber, and the dependence of the average energy density of a pulsed electron beam in the cross section of the calorimeter on the distance at a pressure of 750 Torr.

Fig. 7. Dependence of the ratio of the charge of a pulsed electron beam (q FC) reaching the collector of a Faraday cup on the charge of a pulsed electron beam (q 0) introduced into the drift chamber, and the dependence of the average energy density of a pulsed electron beam in the cross section of the calorimeter on the distance at a pressure of 560 Torr.

Fig. 8. Dependence of the ratio of the charge of a pulsed electron beam (q FC) reaching the collector of a Faraday cup on the charge of a pulsed electron beam (q 0) introduced into the drift chamber, and the dependence of the average energy density of a pulsed electron beam in the cross section of the calorimeter on the distance at a pressure of 375 Torr.
To assess the likelihood of plasma-chemical processes, the minimum values of the specific absorbed energy limited by the collector area of the Faraday cup were calculated. At a pressure of 750 Torr at the closest distance to the accelerator exit window, the average value of the specific absorbed energy in the propagation zone of the pulsed electron beam was 5.2 eV/molecule. The minimum value of the specific absorbed energy for the studied pressure of 750 Torr was 0.2 eV/molecule. At pressures in the drift chamber of 560 and 375 Torr, the maximum values of the specific absorbed energy were 6.7 and 11.4 eV/molecule, respectively. The minimum values of the specific absorbed energy were 0.6 eV/molecule at a pressure in the drift chamber of 560 Torr and 1.8 eV/molecule at a pressure of 375 Torr.
The ratio of the charge of a pulsed electron beam reaching the collector of the Faraday cup (q FC) to the charge of a pulsed electron beam introduced into the drift chamber (q 0), at a moisture content of not more than 20%, hardly depends on pressure in the selected ranges. With the increasing distance from the output window, this value decreases.
At humidity of 50% and pressure of 750 and 560 Torr, the value of the specific absorbed electron energy in the beam cross-section is comparable to a distance of 30 cm. The minimum value of the specific absorbed energy was fixed at a distance of 46 cm at 750 Torr and amounted to 0.3 eV/molecule. At the same distance at a pressure of 560 Torr, this value was 0.9 eV/molecule. The minimum value was fixed at a distance of 46 cm and pressure of 375 Torr: 1.9 eV/molecule. At a pressure of 375 Torr in the drift chamber, the electron beam propagated more efficiently than at pressures of 560 and 750 Torr.
With humidity in the drift chamber of 50% and pressures of 560 and 375 Torr, a decrease in the energy dissipation of the pulsed electron beam was observed, with an increase in charge dissipation compared to the corresponding values for humidity of 15%.
Study on the process of dissipation of charge and energy of a pulsed electron beam in a mixture of gases: nitrogen, carbon dioxide, and oxygen in the presence of ash particles
The results of a study of the process of charge and energy dissipation in a pulsed electron beam in the presence of ash are also presented. The ash concentration was 1.8% at a pressure in the drift chamber of 750 Torr and 2.5% at 560 Torr. Humidity was 50 ± 15% both at 750 Torr and at 560 Torr. The ash used was from coal from the Bogatyr strip mine of the Ekibastuz deposit (Kazakhstan). The ash was composed of carbon, silicon oxide, aluminum oxide, iron oxide, calcium oxide, magnesium oxide, titanium oxide, sulfur oxide, phosphorus oxide, potassium oxide, and sodium oxide.
The resulting diagrams of the ratio of the charge of the pulsed electron beam reaching the collector of the Faraday cup (q FC) and the charge of the pulsed electron beam introduced into the drift chamber (q 0), and the dependence of the average energy density transferred by the pulsed electron beam on distance at different pressures, are presented in Figures 9 and 10.

Fig. 9. Dependence of the ratio of the charge of a pulsed electron beam (q FC) reaching the collector of a Faraday cup on the charge of a pulsed electron beam (q 0) introduced into the drift chamber, and the dependence of the average energy density of a pulsed electron beam in the cross section of the calorimeter on the distance at a pressure of 750 Torr.

Fig. 10. Dependence of the ratio of the charge of a pulsed electron beam (q FC) reaching the collector of a Faraday cup on the charge of a pulsed electron beam (q 0) introduced into the drift chamber, and the dependence of the average energy density of a pulsed electron beam in the cross section of the calorimeter on the distance at a pressure of 560 Torr.
Comparing the values of the currents of a pulsed electron beam reaching the collector of the Faraday cup (I FC), when a pulsed electron beam propagates in a drift chamber at a pressure of 750 Torr in the presence of ash and moisture, it can be seen that the presence of ash affects the efficiency of beam propagation. However, at a pressure of 560 Torr, this feature is not observed. In this case, both in the presence of water and in the presence of ash, the current of the pulsed electron beam reaching the collector of the Faraday cup (I FC) decreases uniformly within the experimental error.
An analysis of the results of thermal profiles of pulses of a pulsed electron beam recorded at various distances from the anode foil when an electron beam passes through a gas mixture simulating flue gases in the presence of ash shows that the uniformity of the energy distribution of a pulsed electron beam in the beam cross-section increases with increasing transport distance.
The values of the specific absorbed energy were calculated without taking into account the mass of ash. The value of the specific absorbed energy of 0.34 eV/molecule was recorded at a pressure of 750 Torr to the maximum distance from the accelerator output window, and the value was 0.98 eV/molecule at a pressure of 560 Torr. The ratio of the pulsed electron beam reaching the collector of the Faraday cup (q FC) to the charge of the pulsed electron beam introduced into the drift chamber (q 0) ranged from 0.46–0.07 and 0.38–0.18 at pressure of 750 and 560 Torr, respectively.
The results demonstrate that with an increase in water vapor in the drift chamber, an increase in the propagation efficiency of a pulsed electron beam is observed. In this regard, when implementing the Ebara process, the length of the reaction chamber can be longer if the process is carried out at a humidity of 50 ± 15% than at a humidity of less than 20%. The presence of ash in the flue gas does not significantly affect the energy dissipation of the pulsed electron beam in the ash concentration range of 1.8–2.5%. The ratio of the charge of the pulsed electron beam reaching the collector of the Faraday cup (q FC) to the charge of the pulsed electron beam (q 0) introduced into the drift chambers at near and far distances are important values in designing the reaction volume of the plasma-chemical cleaning of flue gases.
Conclusion
The charge and energy dissipation of a pulsed electron beam in a gas composed of nitrogen, oxygen, and carbon dioxide in the presence of water vapor and ash particles were comprehensively studied. The beam propagated more efficiently at humidity of 50%. The ratio of the charge of the pulsed electron beam reaching the collector of the Faraday cup (q FC) to the charge of the pulsed electron beam introduced into the drift chamber (q 0) decreased when ash particles are added to the drift chamber (concentration 1.8–2.5%). The pinching of the beam, the formation of a virtual cathode, and the development of various types of instabilities (hose, resistive, and other types) were not fixed. The obtained values of the specific absorbed energy limited by the area of the Faraday cup collector at pressures of 750, 560, and 375 Torr at the closest distance to the accelerator exit window when transported in the presence of water vapor and ash particles exceed the energy values required to start chemical flue gas cleaning processes (5.2 and 8.4 eV). This suggests that a pulsed electron beam with parameters of an accelerating voltage amplitude of 450 kV and a half-amplitude duration of 60 ns can be used to treat the flue gases. The values of the specific absorbed energy limited by the area of the Faraday cup collector at pressures of 750, 560, and 375 Torr at the farthest distance to the accelerator exit window when transported in the presence of water vapor and ash particles make it possible to conclude that the drift pipe of 40 cm long is not suitable for use in the flue gas treatment. The results obtained are of practical importance and can be used in the design and development of a reaction chamber for flue gas treatment using a pulsed electron beam.
Acknowledgments
The reported study was funded by RFBR according to research project No. 18-32-20066. The authors thank Tomsk Polytechnic University (TPU, Tomsk, Russia) for technical support. Empirical experiments with the pulsed electron accelerator were carried out at TPU within the framework of the TPU Competitiveness Enhancement Program grant.