INTRODUCTION
Large seed reserves are a feature of many tropical woody species (Baraloto & Forget Reference BARALOTO and FORGET2007, Foster & Janson Reference FOSTER and JANSON1985, Hammond & Brown Reference HAMMOND and BROWN1995, Kelly Reference KELLY1995, Metcalfe & Grubb Reference METCALFE and GRUBB1995). The utility of these reserves has been linked to many factors: large seed mass may increase the probability that seeds buried deep in the soil are able to establish (Dalling & Harms Reference DALLING and HARMS1999, Molofsky & Augspurger Reference MOLOFSKY and AUGSPURGER1992), and may confer tolerance to seed predators when partially consumed (Harrington et al. Reference HARRINGTON, GADEK and EDWARDS2005, Mack Reference MACK1998). At the post-establishment phase seed reserves can be used to replace seedling tissue consumed by herbivores (Green & Juniper Reference GREEN and JUNIPER2004a, Harms & Dalling Reference HARMS and DALLING1997) or damaged by falling litter and trampling (Clark & Clark Reference CLARK and CLARK1989). Large seed mass may also provide a source of energy reserves that can be used to maintain a positive carbon balance or to supplement photosynthetic carbon gain for seedlings that persist for long periods under very low light conditions (Boot Reference BOOT and Swaine1996; but see Baraloto et al. Reference BARALOTO, FORGET and GOLDBERG2005, Myers & Kitajima Reference MYERS and KITAJIMA2007).
The ability of seed reserves to confer these benefits depends in part on where they are located on the seedling, how long they are conserved by the growing seedling and when they are re-allocated to other structures. In some species, cotyledonary seed reserves are retained for weeks or months after the production of a functional leafy shoot (Dalling & Harms Reference DALLING and HARMS1999, Edwards et al. Reference EDWARDS, GADEK, WEBER and WORBOYS2001, Green & Juniper Reference GREEN and JUNIPER2004b). It has been suggested that this delayed use of cotyledon reserves may in part be determined by the seedling's light environment (Dalling & Harms Reference DALLING and HARMS1999, Rose & Poorter Reference ROSE, POORTER and ter Steege2003). Here we explore how cotyledonary reserves are used in sun and shade in a large-seeded tree species, Gustavia superba (H.B.K.) Berg (Lecythidaceae). The principal characteristics of the seed ecology of this species are the ability to germinate after substantial pre-dispersal seed damage, the preservation of hypogeal cotyledonary reserves long after the establishment of a functional seedling, and the ability to resprout multiple times following browsing damage (Dalling & Harms Reference DALLING and HARMS1999, Harms et al. Reference HARMS, DALLING and AIZPRÚA1997). These characteristics may be common among large-seeded tropical tree species (Baraloto & Forget Reference BARALOTO and FORGET2007, Harms & Dalling Reference HARMS and DALLING1997); they have been reported for taxa in a range of families including Lauraceae (Beilschmiedia, Chlorocardium, Endiandra), Fabaceae (Carapa, Castanospermum, Prioria), Sapindaceae (Castanospora) and Calycanthaceae (Idiospermum) (Dalling et al. Reference DALLING, HARMS and AIZPRÚA1997, Edwards & Gadek Reference EDWARDS and GADEK2002, Edwards et al. Reference EDWARDS, GADEK, WEBER and WORBOYS2001, Green & Juniper Reference GREEN and JUNIPER2004a, Reference GREEN and JUNIPERb; Harms & Dalling Reference HARMS and DALLING1997, ter Steege et al. Reference TER STEEGE, BOKDAM, BOLAND, DOBBELSTEEN and VERBURG1994).
Our previous experimental studies of G. superba seed ecology have examined cotyledonary resource use in the screened growing house under relatively low light conditions (0.4–1.0 mol m−2 d−1 PAR; Dalling & Harms Reference DALLING and HARMS1999, Harms et al. Reference HARMS, DALLING and AIZPRÚA1997). These studies showed that significant cotyledonary resources remained attached to seedlings for up to 9 mo after germination. Removal of cotyledonary reserves significantly affected seedling growth for at least the first 3 wk after germination, and were essential for successful resprouting following a shoot removal treatment that simulated browsing damage (Dalling & Harms Reference DALLING and HARMS1999). Furthermore, cotyledonary resource allocation to resprouting seedlings appears to be tightly regulated; repeated shoot removal from resprouting plants resulted in the production of successively smaller resprouts where each new shoot was constructed from a fixed proportion of remaining cotyledonary reserves (Dalling & Harms Reference DALLING and HARMS1999).
Gustavia superba cotyledons are especially attractive and vulnerable to insect and mammalian predators (Forget Reference FORGET1992, Sork Reference SORK1985, Reference SORK1987), thus retaining cotyledon reserves after emergence may incur costs (Dalling & Harms Reference DALLING and HARMS1999). As large seedlings that lack cotyledons are also able to resprout, Dalling & Harms (Reference DALLING and HARMS1999) suggested that at some point in ontogeny a shift occurs from dependency on seed reserves to reserves stored in stems and roots for resprouting after defoliation events. A similar suggestion was made by Myers & Kitajima (Reference MYERS and KITAJIMA2007) for other tropical tree species. Thus, cotyledonary reserves may be important for resprouting during the first few weeks after seedling emergence, but once roots and stems become developed cotyledonary tissues may be translocated to other parts of the seedling.
In this study we explored how light availability, seed mass and biomass removal affected cotyledonary resource usage and biomass allocation to roots. If cotyledonary persistence primarily functions to permit long-term seedling survival under very low light conditions in forest understoreys (Foster Reference FOSTER1986, Osunkoya et al. 1984), then we predict (1) that the rate of cotyledonary resource use will be higher in the shade than in the sun. If larger seeds maintain a greater proportion of reserves in storage as the seedling develops (i.e. the ‘reserve effect’ hypothesis, Leishman et al. Reference LEISHMAN, WRIGHT, MOLES, WESTOBY and Fenner2000, Westoby et al. Reference WESTOBY, LEISHMAN and LORD1996; but see Baraloto et al. Reference BARALOTO, FORGET and GOLDBERG2005, Green & Juniper Reference GREEN and JUNIPER2004b), then we predict (2) a relatively lower rate of cotyledonary use for seedlings produced from larger seeds. If cotyledonary reserves are an adaptation to permit seedling resprouting after shoot damage (Baraloto & Forget Reference BARALOTO and FORGET2007, Dalling & Harms Reference DALLING and HARMS1999, Green & Juniper Reference GREEN and JUNIPER2004a), then we predict (3) higher cotyledonary use in damaged than in undamaged seedlings. On the other hand, if root reserves, rather than cotyledon reserves, are used to replace shoot tissue after damage (Baraloto & Forget Reference BARALOTO and FORGET2007, Myers & Kitajima Reference MYERS and KITAJIMA2007), then we predict (4) a lower root biomass fraction (RMF) for damaged seedlings compared with undamaged ones when considering both the tissue removed by defoliation and resprout tissue combined.
Thus, in this study we carried out two experiments. We first analysed the effects of light availability on cotyledon persistence and biomass allocation to roots of G. superba seedlings through the first 4 mo after germination. Then, we analysed the effects of light availability and seed size on the response of seedlings to different biomass removal treatments. In particular, we evaluated how light availability and seed size affect cotyledon resource usage and root biomass allocation after seedling damage.
METHODS
Species description and study site
Gustavia superba is a common subcanopy tree of humid tropical forests of Central America (Prance & Mori Reference PRANCE and MORI1979). At the study site, Barro Colorado Island in central Panama (described in detail in Leigh Reference LEIGH1999), G. superba is abundant in 60–100-y-old secondary forest and on exposed peninsulas along the shore of the island (Croat Reference CROAT1978). Gustavia superba produces large (150–600 g) indehiscent fruits that fall to the ground and are broken or opened by scatterhoarding rodents, which subsequently bury some of the seeds (Forget Reference FORGET1992, Sork Reference SORK1985). Fruits contain 2–20 seeds varying in size from 3–30 g. Seeds lack a protective seed coat and consist mostly of cotyledonary tissue (Dalling & Harms Reference DALLING and HARMS1999). Germination of G. superba seeds occurs within a few weeks of dispersal and may be hypogeal if seeds are buried (Molofsky & Augspurger Reference MOLOFSKY and AUGSPURGER1992), or semi-hypogeal when seeds remain on the soil surface (Kitajima Reference KITAJIMA1992). Seeds successfully germinate from small cotyledonary fragments (Harms et al. Reference HARMS, DALLING and AIZPRÚA1997), or following extensive damage by larvae of sesiid moths (Dalling & Harms Reference DALLING and HARMS1999, Harms & Aiello Reference HARMS and AIELLO1995). Leaf area is produced in flushes of 3–12 leaves that developed very quickly, expanding from 20% of full size to full size in 4 d (Aide Reference AIDE1991, Aide & Londoño Reference AIDE and LONDOÑO1989).
Experimental design
In July 2000, we collected seeds from fallen intact fruits beneath c. 10 G. superba trees along Barbour trail on Barro Colorado Island. Seeds were checked for insect infestation (Harms & Aiello Reference HARMS and AIELLO1995) and then stored in dark moist conditions to allow seeds containing insect larvae to be detected. After 1 mo, all rotten or infested seeds were discarded. The remaining intact seeds were weighed individually and ordered according to their fresh mass. Unusually large or small seeds were discarded to leave a subset of 200 seeds (13.80 ± 0.36 g, mean ± SE; range: 4.12–32.52 g). Of these seeds, ten were randomly selected and oven-dried at 70 °C for 48 h to estimate initial dry mass. The remaining seeds were then randomly subdivided into two groups for use in the experiments described below. For Experiment 1 a set of 42 seeds was randomly allocated to two light treatments (both treatments had similar mean seed mass: t-test = −0.18; df = 40; P = 0.86; high light = 12.95 ± 1.08 g and low light = 13.21 ± 1.08 g). For Experiment 2 the remaining seeds were divided into ‘small’ (10.06 ± 0.24 g; mean ± SE; range 6.61–11.98 g) and ‘large’ seeds (18.22 ± 0.66 g; range 12.88–27.89 g) and then also allocated to two light treatments in a stratified random manner to ensure equality of mean seed masses.
In August 2000, seeds from both experiments were placed in a screened growing house under the two light conditions. A ‘sun’ treatment simulated light conditions in small treefall gaps (7–12 mol m−2 d−1 PAR; approximately 10% of full sun), and the ‘shade’ treatment simulated forest understorey conditions (0.4–0.8 mol m−2 d−1 PAR; approximately 0.6% of full sun). Seeds were sown individually on the surface of large (7.5 L) pots to prevent seedlings from becoming pot-bound, and were filled with a 3:1 mixture of forest soil and rinsed sea sand. Pots were watered once a week as required. Several seedlings developed two or more concurrent above-ground sprouts. These seedlings were removed from the data prior to analyses (Dalling & Harms Reference DALLING and HARMS1999, Green & Juniper Reference GREEN and JUNIPER2004a).
Experiment 1: Light effects on cotyledonary resource use and biomass allocation
To determine how light availability influences cotyledon persistence and biomass allocation we followed seedling growth over the first 4 mo after seed germination. We tracked the germination and leaf expansion date of each seedling, and harvested three seedlings from each light treatment at seven time periods (4, 7, 12, 20, 34, 55 and 90 d after first full leaf expansion). At harvest time we measured seedling height and calculated leaf area from regression equations developed in a separate field study (Barberis Reference BARBERIS2001). Seedlings were then separated into leaves, stem, root and cotyledon and their dry mass estimated. For each harvest date we calculated the proportion of cotyledonary mass remaining (i.e. cotyledon dry mass at the end/initial seed dry mass calculated by regression; CR/CI). We also calculated the mass fraction of biomass (excluding cotyledons) allocated to root (RMF), stem (SMF) and leaves (LMF), where RMF + SMF + LMF = 1. Finally, we calculated the mass fraction of biomass allocated to cotyledons (CMF).
Experiment 2: Light and seed-size effects on response to simulated herbivory
To determine how light and seed size affect the ability of seedlings to resprout we tracked the germination and leaf expansion of individual seedlings as before. Three weeks after full leaf expansion, eight seedlings from each light and seed-size treatment combination were allocated to one of the following damage treatments: (1) control (no damage) (2) 100% defoliation, (3) 100% defoliation and stem excision just above the cotyledonary attachment. These damage levels were designed to simulate common damage suffered by G. superba seedlings when attacked by insect herbivores (treatment 2) or when browsed by mammals or damaged by falling debris (treatment 3). At the time of treatment application we dried and weighed the leaves and stem harvested from each plant, and measured the leaf area harvested.
Six weeks after treatment application, seedlings were harvested and separated into leaves, stem, root and cotyledons. Plant height, leaf number, leaf area, root length and dry mass were measured, and the specific leaf area (SLA; leaf area per unit leaf dry mass) was calculated. For each combination of light, seed size and biomass removal treatment we calculated the leaf area ratio (LAR; leaf area per unit total plant dry mass) and biomass allocation (excluding cotyledons) to root (RMF), stem (SMF) and leaf (LMF). As in the first experiment, we also calculated the mass fraction of biomass allocated to cotyledons (CMF). These variables were calculated for the final harvest mass only (H), and also for the sum of the harvest mass and the mass of excised tissue removed when treatments were applied (H+T). Finally, we estimated the proportion of the initial cotyledon mass remaining by the end of the experiment (CR/CI). During the experiment several seedlings were damaged by agoutis, which entered the screened growing house, reducing the final sample size to 74 seedlings.
Statistical analyses
Experiment 1
The effects of light treatment on (1) time to seedling emergence, (2) time from seedling emergence to the stage of fully expanded leaves, and (3) the initial number of expanded leaves were analysed using Mann–Whitney U-tests. The effect of light treatment on seedling height and leaf area at full leaf expansion was analysed with t-tests. Both variables were log10-transformed to improve the homogeneity of variances.
Differences in cotyledon mass persistence (CR/CI), plant architecture (height, leaf area and biomass) and biomass allocation (RMF, SMF, LMF and CMF) through time between light environments were analysed separately with general linear models. Light environment was used as a categorical factor and time (in log10 d after leaves were fully expanded; logdays) was treated as a covariate. Data were analysed for residual normality and homoscedasticity. All variables were either log10- or arcsine-transformed to improve the homogeneity of variance. The slopes of the relationships between the transformed response variables and logdays were compared using a model that included the logdays × transformed response term. Where the interaction term was not significant, the model was refitted, assuming a common slope, and intercepts were compared. F-tests were run considering Type III Sums of Squares.
Experiment 2
General linear models were used to analyse the effects of seed size, light environment, different damage levels and their interactions on (1) time to seedling emergence, (2) time from seedling emergence to the stage of fully expanded leaves, and (3) seedling height, leaf number and leaf area at the time of full leaf expansion and at treatment application time. Variables were log10-transformed to improve homogeneity of variances.
The effects of seed size, light environment and different biomass removal intensities on leaf number, stem length (height), root length, leaf area, total biomass, root mass, stem mass, leaf mass, cotyledon mass, SLA, LAR, RMF, SMF, LMF and CMF were analysed separately with general linear models. Variables were log10- or arcsine-transformed to improve the homogeneity of variance where appropriate. F-tests were run considering Type III Sums of Squares. As there were no effects of seed size or its interaction with habitat and damage treatments for all allocation variables, we pooled the small- and large-seed data and ran the tests again, without including the seed size factor. All tests were done using SAS 8.0 (SAS Institute Inc., Cary, North Carolina, USA).
RESULTS
Experiment 1
Light treatment did not affect the timing of seedling emergence (Sun median = 24 d and shade median = 22 d; Mann–Whitney U-test: U = 463; N = 21 for both samples; P = 0.77), but affected the time elapsed between emergence and full leaf expansion (U = 284; N = 21 for both samples; P < 0.001). Seedlings grown in the sun grew faster (median = 13 d) than seedlings grown in the shade (median = 22 d). At the time of full leaf expansion there were no differences between seedlings grown in high or low light either in leaf number (median for both samples = 5 leaves; U = 468; N = 21 for both samples; P = 0.67) or in leaf area (Sun = 130.0 cm2 and shade = 133.7 cm2; t-test: t = −0.12; df = 40; P = 0.91). However, seedlings grown in the sun were shorter compared to those in the shade (Sun = 12.1 cm and shade = 18.6 cm; t = −5.53; df = 40; P < 0.001).
Total biomass without cotyledons increased with time (Figure 1a). However, overall, the net transfer of resources out of the cotyledons resulted in a non-significant change in total seedling biomass with time (Table 1, Figure 1b). Throughout the study period, seedlings increased their non-cotyledon root mass fraction as well as their leaf number, stem and root length, and decreased their leaf mass fraction; stem mass allocation was unchanged (Table 1; Figure 1c–h). Cotyledonary mass, and the fraction of cotyledonary reserves remaining (CR/CI) decreased (Table 1, Figure 1i). There were significant interactions between time since leaf expansion (logdays) and light environment for several variables (Table 1). Seedlings grown in the sun had longer and heavier roots (Figure 1c, g), whereas seedlings grown in the shade allocated more to stem biomass (Figure 1d). In contrast there was no difference in the proportion of cotyledon mass remaining (CR/CI) at harvest between light environments (Figure 1i).

Figure 1. Variation in plant size (a, b, f, g, h), biomass allocation (c, d, e), and cotyledon resource use (i) in relation to time after leaves were fully developed for Gustavia superba seedlings grown in sun (empty circles) and shade (filled circles). N = 3 for each Time × Light treatment combination. Mean and SE are shown. L×T denotes Light × Treatment interactions. Note that Time (d) is plotted on a log scale.
Table 1. Summary statistics for general linear models performed on plant trait data of Gustavia superba. Bold values denote significant differences (P < 0.05). Where the Time × Light interaction was not significant general linear models were re-run omitting the interaction term.

Experiment 2
The effects of light environment on the timing of emergence, time to full expansion, leaf number, area and stem length were similar to those reported for Experiment 1. Seed mass did not affect the timing of seedling emergence (large seeds = 19.9 ± 1.2 d; small seeds = 20.6 ± 1.4 d; F1,62 = 0.15, P = 0.69), or the time elapsed between emergence and full leaf expansion (large seeds = 13.6 ± 0.4 d; small seeds = 14.6 ± 0.5 d; F1,62 = 2.21, P = 0.14). At the stage of full leaf expansion seedlings from large seeds had more leaves (large seeds = 5.0 ± 0.1 leaves and small seeds = 4.4 ± 0.1 leaves; F1,62 = 7.41, P = 0.008), and a higher leaf area (large seeds = 160.7 ± 1.07 cm2 and small seeds = 115.6 ± 1.07 cm2; F1,62 = 11.62, P = 0.001) compared with those from small seeds.
There were no significant differences in seed size, seedling emergence or growth among biomass removal treatments before treatment application (all P > 0.05). At treatment time, seedlings that originated from larger seeds had higher leaf mass (F1,62 = 17.9, P < 0.01), and leaf area (F1,62 = 13.3, P < 0.01) than those from smaller seeds. In contrast, there were no differences in these variables between light treatments (all P > 0.05). There were no differences among biomass removal treatments for leaf mass and leaf area (both P > 0.05).
Seed size had no effect on biomass allocation patterns (SLA, LAR, RMF, SMF and CMF were not significantly different between large and small seeds; all P > 0.05; data not shown). However, at harvest time, seedlings produced from large seeds had greater total biomass, greater root, stem, leaf and cotyledon mass, and larger leaf area and leaf number than seedlings from small seeds (Table 2; Figure 2 a-g). Cotyledonary persistence (CR/CI) was unaffected by seed mass (F1,62 = 1.98; P = 0.164). Moreover, there were no significant interactions of seed mass with light environment or biomass removal (for all size and biomass allocation variables P > 0.05; data not shown).
Table 2. Summary statistics (F and P values) for general linear models performed on plant size of Gustavia superba for two data sets (a) excluding the biomass of removed tissues and (b) including the biomass of removed tissues. Bold values denote significant differences (P < 0.05). Light: high vs. low light. Seed size: Small seeds vs. large seeds. Biomass removal: control (no damage), 100% defoliation, and 100% defoliation and shoot excision. For all other interactions there were no significant differences (P > 0.05).

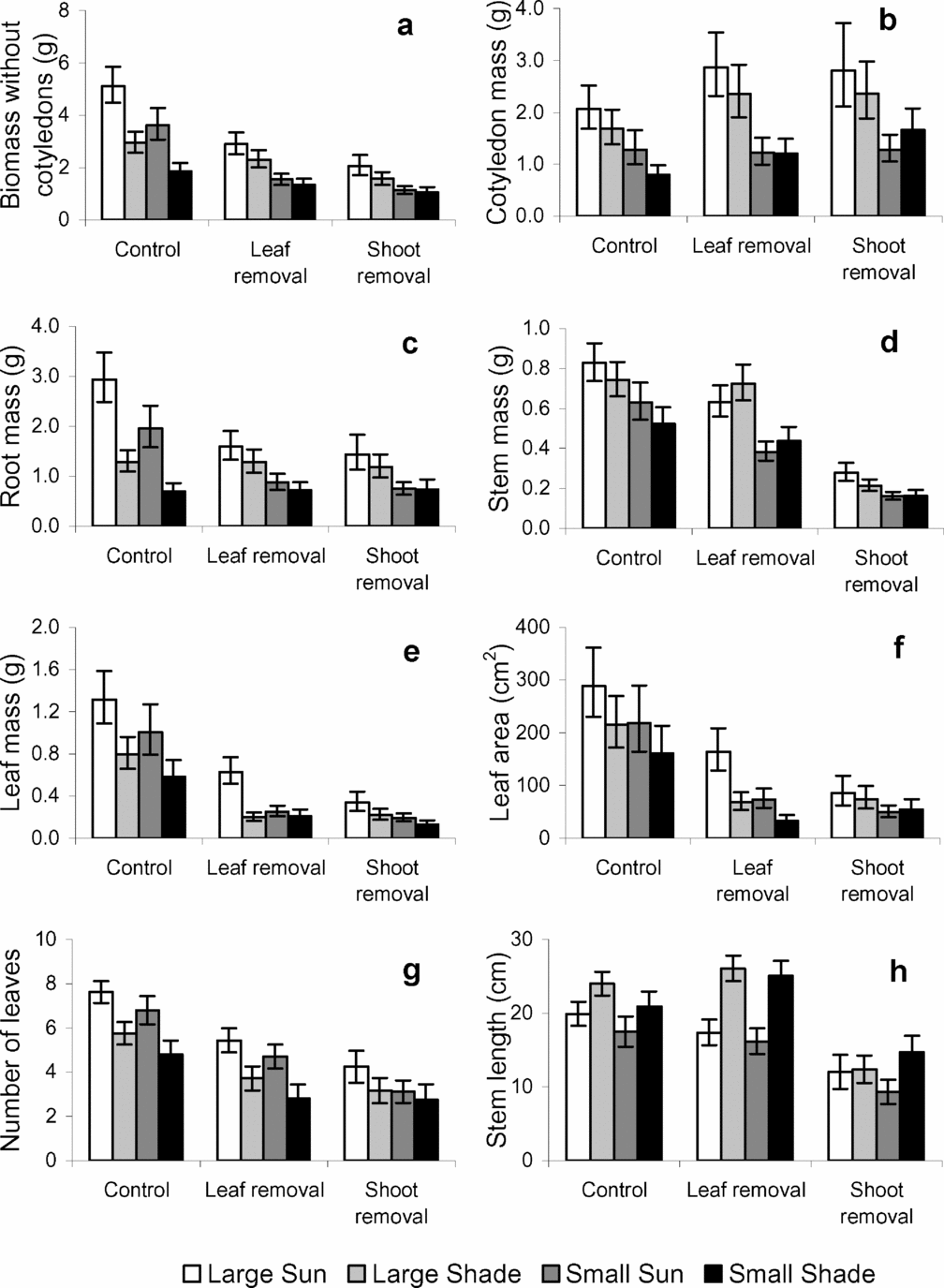
Figure 2. Effects of light, seed size and biomass removal on total seedling biomass excluding cotyledons (a), biomass components (b-e), leaf area and number (f–g) and stem length (h) for Gustavia superba seedlings at the time of harvest. Mean and SE are shown.
As observed in Experiment 1, seedlings grown in high light were shorter, but had more leaves, leaf area, and biomass (root and shoot mass) compared with seedlings grown in the shade (Table 2, Figure 2). Biomass removal (i.e. leaf or shoot removal 3 wk after leaves were fully expanded) reduced leaf number, leaf area and total biomass by the end of the experiment (Table 2, Figure 2). However, most of these treatment effects on biomass variables disappeared when the tissue biomass harvested at treatment time was added to the final harvest biomass (Table 2).
As expected, removal of shoot or foliar tissue modified most biomass allocation patterns at harvest time (Table 3, Figure 3a–f). Thus cotyledon mass fraction (CMF) ranged from 0.3 in control plants to almost 0.6 in damaged seedlings (Table 3; Figure 3d). However, biomass removal did not affect the proportion of cotyledonary mass remaining by the end of the experiment (CR/CI; Figure 3g). Non-defoliated seedlings had higher LMF, higher LAR and lower SLA than seedlings from both shoot and leaf removal treatments (Table 3; Figure 3c, e, f). They also had lower RMF and higher SMF than those from the shoot removal treatment, but not than those from the leaf removal treatment (Table 3; Figure 3a, b).
Table 3. Summary statistics (F and P values) for general linear models performed on allocation variables for Gustavia superba plants growing in different light environments (high vs. low), and under different biomass removal treatments (control (no damage), 100% defoliation, and 100% defoliation and shoot excision) for two data sets (a) excluding the biomass of removed tissues and (b) including the biomass of removed tissues. Bold values denote significant differences (P < 0.05).

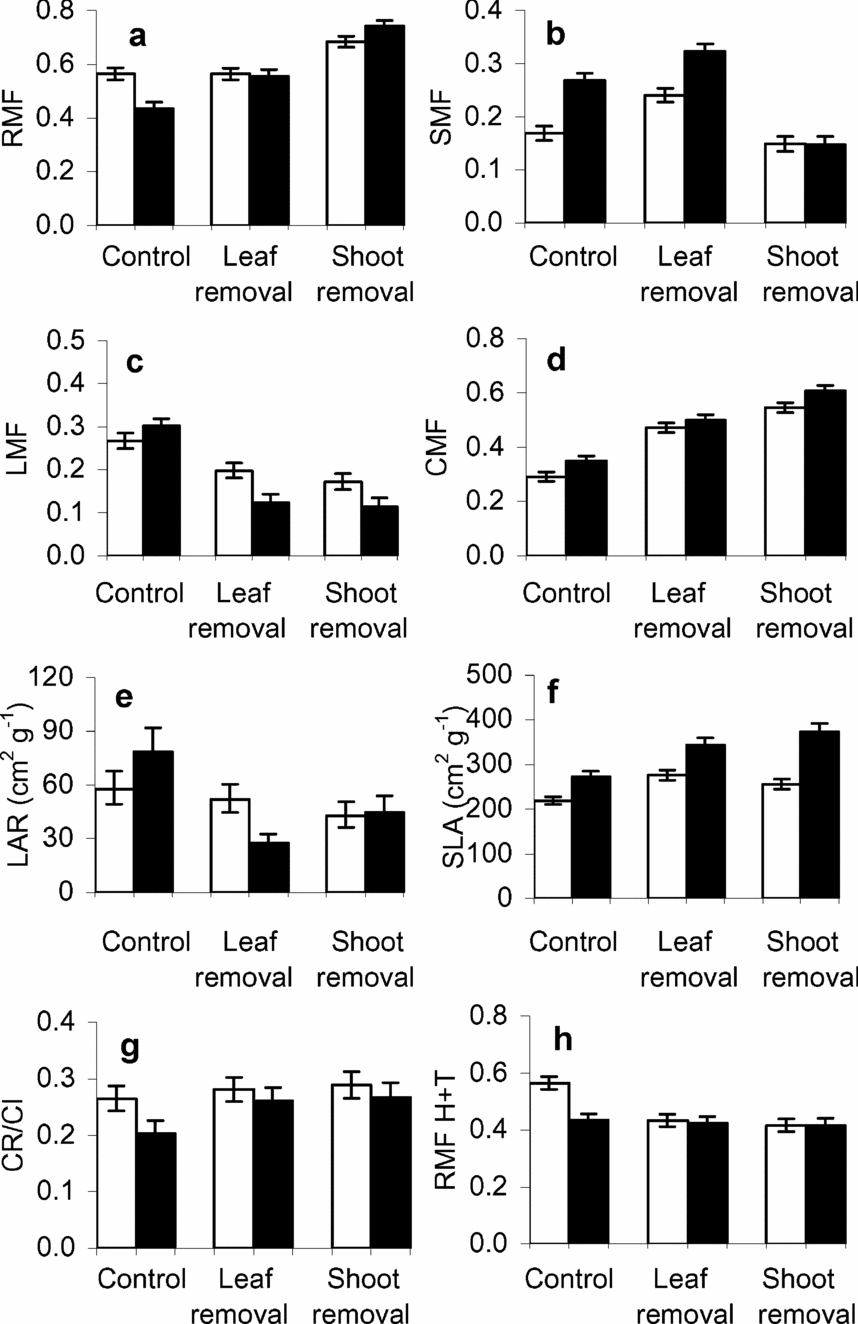
Figure 3. Effects of light and biomass removal on biomass fractions (a–d, h), leaf area allocation (e–f) and cotyledon resource usage (g) at harvest time for Gustavia superba seedlings grown in high light (open bars) and low light (filled bars). Mean and SE are shown. H+T denotes the biomass harvested at treatment time added to the resprout biomass.
There were no interactions between seed size and damage treatments for any size or allocation variable (all P > 0.05; data not shown). In contrast, there were significant interactions of light environment with the damage treatments for several size and allocation variables (Tables 2 and 3; Figures 2 and 3). Excluding cotyledonary mass, only seedlings that were in the no-damage treatment grew larger and had higher root mass, in high versus low light (Table 2; Figure 2a, c). In low light, there were no differences among biomass removal treatments in RMF when tissue biomass harvested at treatment time was added to the final harvest data (Table 3; Figure 3h). In contrast, in high-light conditions, seedlings of both damage treatments had lower RMF when tissue biomass harvested at treatment time was added to the final harvest data than undamaged seedlings (Figure 3h). However, it should be noted that RMF following resprouting was not related to the resprout biomass (Figure 4a, b), nor was it related to the sum of total biomass produced at harvest and resprout shoot biomass (Figure 4c, d).
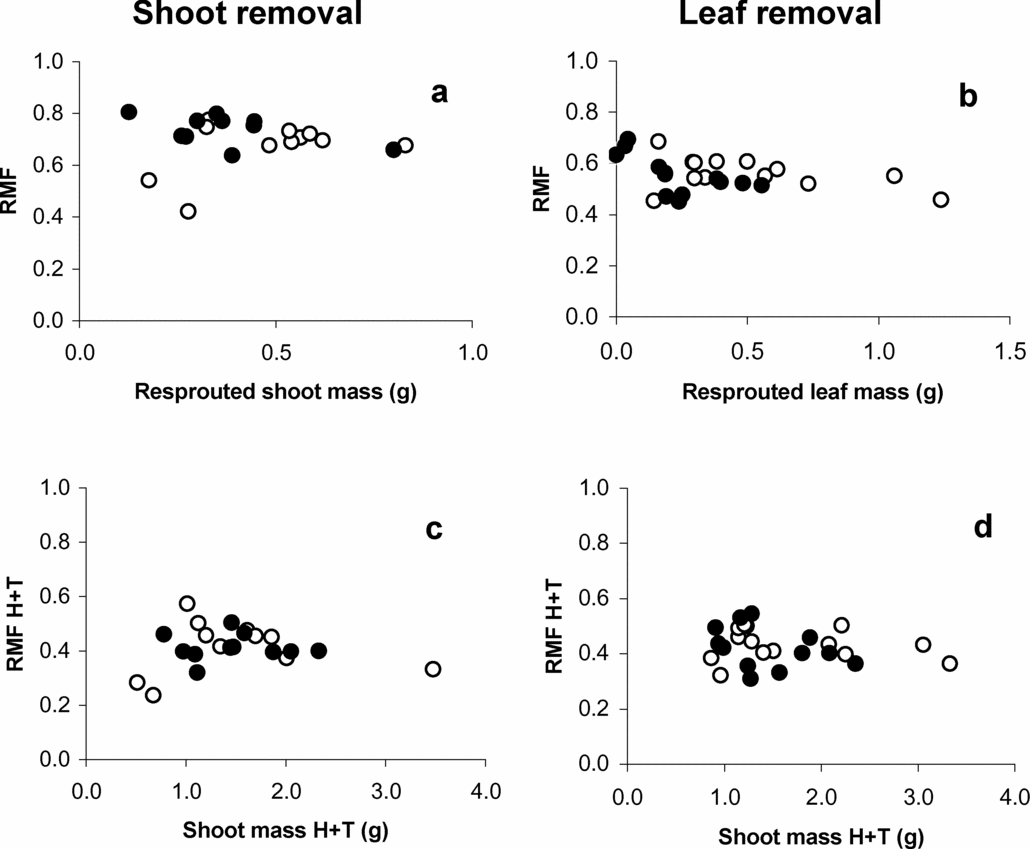
Figure 4. Variation of root mass fraction in relation to resprouted (a, b) and total shoot biomass (c, d) for shoot (a, c) and leaf removal (b, d) treatments in high light (open circles) and low light (filled circles) environments for Gustavia superba seedlings. H+T denotes the biomass harvested at treatment time added to the resprouted biomass.
DISCUSSION
Light effects on cotyledonary resource use and biomass allocation
In common with several large-seeded tropical tree species (Dalling & Harms Reference DALLING and HARMS1999, Dalling et al. Reference DALLING, HARMS and AIZPRÚA1997, Edwards & Gadek Reference EDWARDS and GADEK2002, Edwards et al. Reference EDWARDS, GADEK, WEBER and WORBOYS2001, Green & Juniper Reference GREEN and JUNIPER2004b), less than half of the cotyledonary mass of G. superba was used for seedling construction (about 40% when first leaf expansion occurred). About 20% of the cotyledonary mass of G. superba still remained 90 d after first full leaf expansion in both high- and low-light environments. Thus, our results did not support our first hypothesis that the retention of cotyledonary tissue after seedling emergence provides resources to supplement photosynthetic carbon gain under low-light conditions. This is consistent with observations made on Chlorocardium rodiei seedlings by ter Steege et al. (Reference TER STEEGE, BOKDAM, BOLAND, DOBBELSTEEN and VERBURG1994). Based on the assumption that all Chlorocardium seedlings were 1 y old, they found no difference in cotyledonary mass along a gradient of light availability. However, in an accompanying experiment Chlorocardium seedling survival over 8 wk was strongly negatively affected by cotyledon removal (ter Steege et al. Reference TER STEEGE, BOKDAM, BOLAND, DOBBELSTEEN and VERBURG1994).
In agreement with previous field studies, G. superba seedlings grown in high light were larger than those from low light (Molofsky & Fisher Reference MOLOFSKY and FISHER1993, Sork Reference SORK1987), and had higher root:shoot ratios than seedlings grown in low light (Barberis Reference BARBERIS2001). These differences in biomass allocation between light environments may simply be related to ontogeny (i.e. ‘apparent plasticity’; Weiner Reference WEINER2004, Wright & McConnaughay Reference WRIGHT and MCCONNAUGHAY2002). As G. superba produces leaves in flushes (Aide Reference AIDE1991), and we recorded differences in root mass but not in leaf or stem mass between light environments, high-light plants may have been accumulating carbohydrate reserves in their roots prior to the next leaf flush. This is possible as G. superba has a coarse taproot (López & Kursar Reference LÓPEZ and KURSAR1999, Tyree et al. Reference TYREE, VÉLEZ and DALLING1998), which is likely to store reserves as observed in other tropical species (Ichie et al. Reference ICHIE, NINOMIYA and OGINO2001). Differences in RMF between high- and low–light-grown plants, despite similar rates of reduction of cotyledonary resources, probably therefore reflect a combination of cotyledonary resource and photosynthate translocation to roots in high-light plants, and possibly, cotyledonary resource allocation to shoot mass in low-light plants.
Light and seed size effects on response to simulated herbivory
Our results did not support our second hypothesis that cotyledonary persistence depends on seed size. We predicted a lower rate of cotyledonary resource use for seedlings produced from larger seeds based on the ‘reserve effect’ hypothesis (Leishman et al. Reference LEISHMAN, WRIGHT, MOLES, WESTOBY and Fenner2000, Westoby et al. Reference WESTOBY, LEISHMAN and LORD1996), which states that larger seeds maintain a greater proportion of reserves in storage as the seedling develops. The absence of a seed size effect on seedling growth or allocation after seedling damage is consistent with the results observed by Green & Juniper (Reference GREEN and JUNIPER2004a), and with our earlier result where sequential resprout mass produced by individual seeds was a fixed proportion remaining cotyledonary mass (Dalling & Harms Reference DALLING and HARMS1999). In agreement with Baraloto & Forget (Reference BARALOTO and FORGET2007) we did not find any interaction between seed size, light and damage treatment. Thus there is no evidence for a ‘reserve effect’ in G. superba at least at the range of seed masses included in this experiment.
Biomass removal (either as leaf or shoot tissue) did not affect cotyledonary use (i.e. CR/CI) despite affecting biomass allocation to roots, stem and leaves. Thus our data did not support our third hypothesis. If cotyledon reserves are not used for resprouting after biomass removal, it follows that seedlings must use reserves from other organs. It is likely that reserves came from roots, because in high light, damage-treated seedlings had a lower RMF than undamaged ones when all biomass was included (i.e. sum of harvest biomass plus tissue removed when treatments applied). Surprisingly, however this pattern was not observed for seedlings grown in low light, perhaps because the resprout biomass was insufficient to cause a detectable reduction in RMF.
Using observations of biomass allocation through time in our first experiment, we can conclude that, at the time that damage treatments were applied (i.e. 20 d after leaves fully expanded), there would have been no differences between light treatments in RMF (Figure 1a). Forty-two days later, at harvest time, there was an interaction between light and biomass removal treatments for root mass and RMF, such that only seedlings in the no damage treatment grew larger and had higher root mass in high versus low light. Thus, there were no differences in RMF between light treatments for leaf or shoot removal treatments. The absence of a light treatment effect on biomass allocation in the damage treatments may be explained by the time to leaf flushing. While there were no differences in the timing of leaf flush between light treatments for leaf or shoot removal treatments (P > 0.05, data not shown), undamaged seedlings grown in high light flushed earlier than those in the shade (17 ± 2 d vs. 28 ± 3 d, respectively; P < 0.05). Based on the lack of differences in root biomass allocation and timing of leaf flush between light environments, it seems that the rate of reserve translocation from root to shoot after damage was similar between light environments. To detect differences between light treatments in reserve translocation, future experiments need to be longer and to include different times of damage application. It would also be useful to include a low light level below the light compensation point, to prevent photosynthate accumulation during the experiment (see Myers & Kitajima Reference MYERS and KITAJIMA2007).
Overall, these results partially support our fourth hypothesis and also the suggestion of Dalling & Harms (Reference DALLING and HARMS1999) that cotyledonary reserves are important for resprouting mainly in the first few weeks after seedling emergence, when shoot damage is most likely to occur. Once roots become developed however, cotyledonary reserves are slowly translocated to roots. It should be noted here that we tracked resource allocation using biomass fractions. Increases in RMF and SMF are assumed to reflect greater allocation to carbon storage, however the same pattern would also be observed if plants invested heavily in structural tissue for defence (Canham et al. Reference CANHAM, KOBE, LATTY and CHAZDON1999). As pointed out by Baraloto et al. (Reference BARALOTO, FORGET and GOLDBERG2005), future studies will require not only measurements of cotyledon and seedling biomass through time, but also quantification of structural vs. non-structural carbohydrates in stem and root tissue (Myers & Kitajima Reference MYERS and KITAJIMA2007).
The question that still remains is why G. superba retains its cotyledons for weeks after germination, exposing them to damage or removal from scatter-hoarding rodents. A plausible explanation is that cotyledons are retained for as long as necessary for the seedling to develop a taproot to translocate the reserves into. Furthermore, exposed cotyledons that are removed by scatter-hoarders have the potential to develop elsewhere; even small cotyledon fragments of G. superba are capable of sprouting into new seedlings through apparent somatic embryogenesis (Harms et al. Reference HARMS, DALLING and AIZPRÚA1997, Harrington et al. Reference HARRINGTON, GADEK and EDWARDS2005).
ACKNOWLEDGEMENTS
I.M.B. acknowledges FOMEC, Universidad Nacional de Rosario, Argentina, for financial support for his PhD including the fieldwork and living expenses in Panama. C. Hernández and D. Morán helped with the fieldwork. Y. Mendoza and E. Ayarza helped to weigh the dry samples. Kyle Harms, Jonathan Myers and two anonymous reviewers provided valuable comments that improved the manuscript.