INTRODUCTION
Hydrothermal vent fields in the deep sea are typically distributed along the mid-ocean ridges generated by seafloor spreading, the volcanic arcs and back-arcs created by subduction, and also on ridge flanks and hot-spot volcanoes (Mullineaux, Reference Mullineaux, Bertness, Bruno, Silliman and Stachowicz2014). Since the discovery on the Galapagos Rift in 1977, at least 521 active vent fields have been documented around the world (Beaulieu et al., Reference Beaulieu, Baker, German and Maffei2013). Their invertebrate communities – predominantly composed of molluscs, crustaceans and annelids – depend on the primary production of chemoautotrophic bacteria (Van Dover, Reference Van Dover2000) and occur with high levels of endemism and biomass (Desbruyères et al., Reference Desbruyères, Segonzac and Bright2006).
The Mid-Atlantic Ridge (MAR) forms one of the five biogeographic provinces for the global hydrothermal vent communities (Moalic et al., Reference Moalic, Desbruyères, Duarte, Rozenfeld, Bachraty and Arnaud-Haond2012). At least nine vent fields exist on MAR to host faunal assemblages at the depth range of 810–4200 m (Kelley & Shank, Reference Kelley, Shank, Rona, Devey, Dyment and Murton2010; Wheeler et al., Reference Wheeler, Murton, Copley, Lim, Carlsson, Collins, Dorschel, Green, Judge, Nye, Benzie, Antoniacomi, Coughlan and Morris2013). More than 225 endemic species have been reported from this province with richness ranging from less than 30 to over 100 at each field (Kelley & Shank, Reference Kelley, Shank, Rona, Devey, Dyment and Murton2010). Individual fields along MAR are separated not only by geographic distances of several dozens to thousands of kilometres but also large topographic discontinuities, bathymetric differences and shearing oceanic currents. These potential barriers to dispersal might favour divergence among sites (Van Dover, Reference Van Dover1995; Desbruyères et al., Reference Desbruyères, Almeida, Comtet, Khripounoff, Le Bris, Sarradin and Segonzac2000).
Previous studies on the genetic connectivity of vent animals along MAR suggest different levels and patterns of variation, presumably depending on their larval ecology. Among five animal lineages so far investigated in this context, two lineages are each divided by a dispersal barrier into multiple species or populations, while three others exhibit genetic panmixia. The former includes the case of Bathymodiolus azoricus and B. puteoserpentis, reciprocal sister species in the bivalve family Mytilidae. These mussel species have largely parapatric distributions in the north and south, respectively, with a narrow overlapping zone at the Broken Spur vent field at 29°N (O'Mullan et al., Reference O'Mullan, Maas, Lutz and Vrijenhoek2001; Breusing et al., Reference Breusing, Biastoch, Drews, Metaxas, Jollivet, Vrijenhoek, Bayer, Melzner, Sayavedra, Petersen, Dubilier, Schihabel, Rosentiel and Reusch2016; see Figure 1 for the location of vent sites). Genetic discontinuity has also been shown for the commensal annelid Branchipolynoe seepensis that lives inside the mantel cavity of the same mussels, but in this case between the Snake Pit (23°N) and Logatchev (15°N) sites to the south (Daguin & Jollivet, Reference Daguin and Jollivet2005).
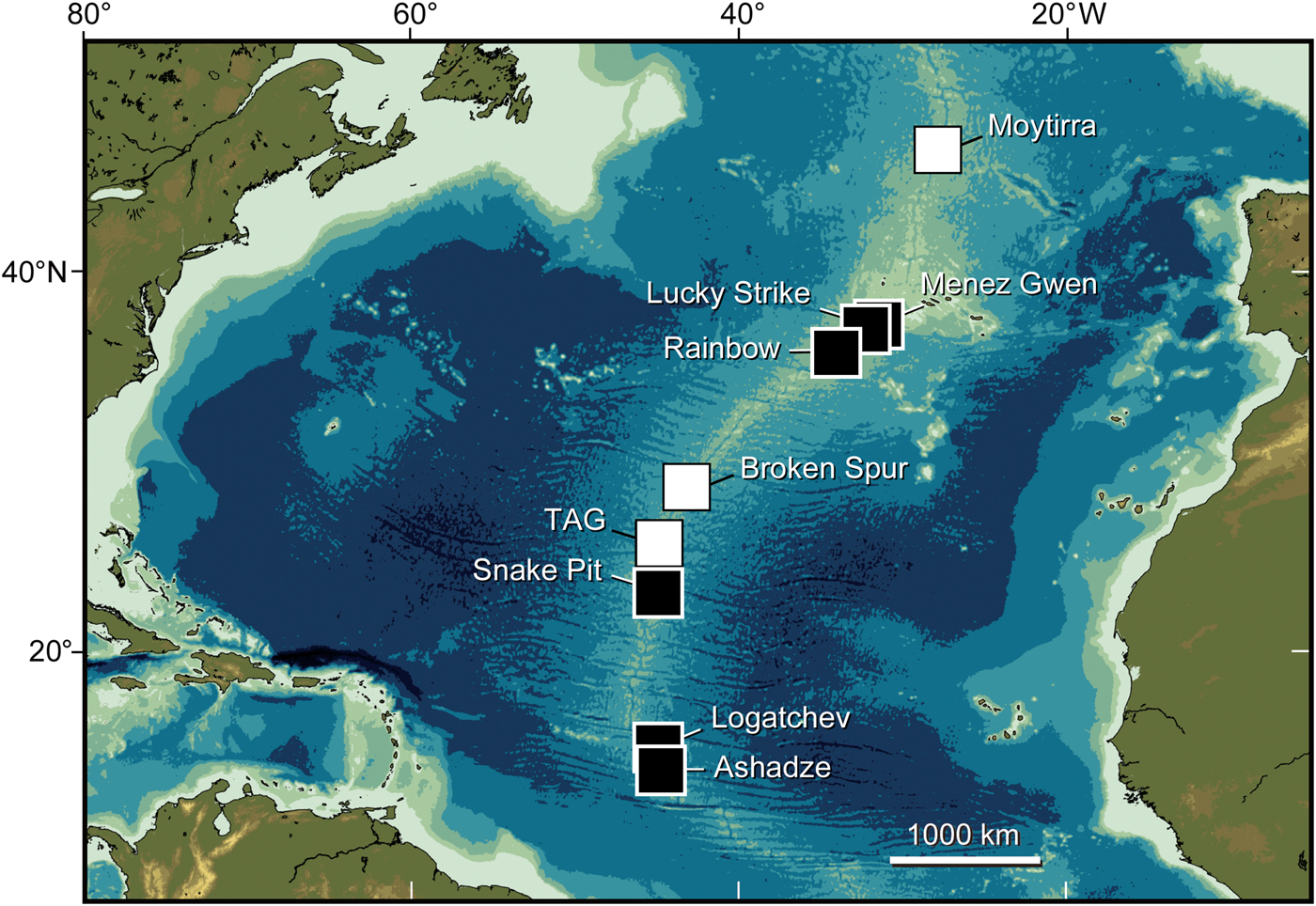
Fig. 1. Location of hydrothermal vent fields along northern Mid-Atlantic Ridge. Solid and open squares represent vents with and without ‘Shinkailepas’ briandi, respectively. DNA sequences were determined for specimens from Menez Gwen, Lucky Strike, Logatchev and Ashadze.
Three shrimp species of the family Alvinocarididae instead show population connectivity along the entire ridge. Rimicaris exoculata, among the most abundant animal species at vents on MAR, has a panmictic population that stretches 7100 km from the Rainbow field at 36°N to the South MAR at 5°S (Teixeira et al., Reference Teixeira, Cambon-Bonavita, Serrão, Desbruyéres and Arnaud-Haond2011, Reference Teixeira, Serrão and Arnaud-Haond2012). Another species in the same genus similarly shows genetic homogeneity between the Lucky Strike (37°N) and Logatchev (15°N) vent fields (Teixeira et al., Reference Teixeira, Olu, Decker, Cunha, Fuchs, Hourdez, Serrão and Arnaud-Haond2013: ESU2). The third, confamilial species in the genus Alvinocaris demonstrates population connectivity not only within MAR but also with cold methane seeps in the Gulf of Mexico and off the west coast of Africa (Teixeira et al., Reference Teixeira, Olu, Decker, Cunha, Fuchs, Hourdez, Serrão and Arnaud-Haond2013: ESU1). Larvae of alvinocaridid shrimps are thought to feed and disperse in mid-water, far above the influence of a hydrothermal plume, so that such barriers as depth, seafloor topography and transform faults would not constrain the connectivity of its local populations (Teixeira et al., Reference Teixeira, Cambon-Bonavita, Serrão, Desbruyéres and Arnaud-Haond2011; see also Adams et al., Reference Adams, Arellano and Govenar2012). Additional information on the population connectivity and early-life traits of various animal taxa on MAR would contribute towards a more inclusive understanding of formation of the hydrothermal vent fauna.
Here we focus on a gastropod species with wide geographic and bathymetric distributions to explore the larval dispersal and connectivity between vent fields along MAR. The red-blooded limpet ‘Shinkailepas’ briandi Warén & Bouchet, Reference Warén and Bouchet2001 (Neritimorpha: Phenacolepadidae; a new genus is now being proposed elsewhere) represents one of the commonest gastropods that occur at a depth range of 810–4090 m in the Menez Gwen, Lucky Strike, Rainbow, Snake Pit, Logatchev and Ashadze fields (Warén & Bouchet, Reference Warén and Bouchet2001; Kelley & Shank, Reference Kelley, Shank, Rona, Devey, Dyment and Murton2010; Fabri et al., Reference Fabri, Bargain, Briand, Gebruk, Fouquet, Morineaux and Desbruyeres2011; Figures 1 & 2). This species is found in clusters on rocks and shells of Bathymodiolus in volcanically active zones near venting orifices (Cuvelier et al., Reference Cuvelier, Sarradin, Sarrazin, Colaço, Copley, Desbruyères, Glover, Serrão Santos and Tyler2011). Its digestive system, including the radula, shares the same characteristics with other phenacolepadids (Fretter, Reference Fretter1984; Warén & Bouchet, Reference Warén and Bouchet2001), suggesting grazing on bacterial mats on hard substrates (Beck, Reference Beck1992; Kano et al., Reference Kano, Chiba and Kase2002). The aim of this paper is to investigate the population connectivity of ‘S.’ briandi along MAR as the first such study for gastropods and to explore the importance of larval duration and vertical migration for the biogeography of vent-endemic animal species.

Fig. 2. ‘Shinkailepas’ briandi from Menez Gwen (A; SMNH-89175; DNA: AORI_YK#866), Logatchev (B; SMNH-92814; AORI_YK#867) and Ashadze (C; SMNH-103209; AORI_YK#2872). All specimens were used for DNA sequencing.
MATERIALS AND METHODS
Population genetic structure of ‘Shinkailepas’ briandi was analysed using a total of 34 specimens deposited in the Swedish Museum of Natural History (SMNH). These specimens originated from four hydrothermal-vent fields, including Menez Gwen (2 specimens; SMNH-89175 and 89169), Lucky Strike (11; SMNH-84610), Logatchev (6; SMNH-92814) and Ashadze (15; SMNH-103209 and 103211), which collectively cover the geographic and bathymetric ranges of the species (Table 1; Figure 1).
Table 1. Locality data for ‘Shinkailepas’ briandi used in population genetic analysis.

Total DNA was extracted from foot tissue using the DNeasy Tissue Extraction Kit (Qiagen). 1327-bp fragments of the mitochondrial cytochrome c oxidase subunit I (COI) gene were amplified by the polymerase chain reaction (PCR) using the primer pair LCO1490 and COIa-NER (Folmer et al., Reference Folmer, Black, Hoeh, Lutz and Vrijenhoek1994; Kano & Kase, Reference Kano and Kase2004). Amplification was performed in 25 µl reaction mixtures of 17.5 µl DDW, 2.5 µl EX Taq buffer (10×), 2.0 µl dNTP mixture (2.5 µM each), 0.3 µl of each forward and reverse primer (20 µM), 0.13 µl Ex Taq Hot Start Version (TaKaRa) and 2.5 µl genomic DNA. PCR reactions were carried out with an initial denaturation step at 94°C for 40 s, followed by 35 cycles consisting of a denaturation step at 94°C (40 s), an annealing step at 42°C (60 s) and an extension step at 72°C (90 s). Amplicons were purified by ExoSAP-IT (USB) following the described protocol.
Sequencing reactions were prepared using a Big Dye Terminator Cycle Sequence Kit 3.1 (Applied Biosystems) and either of the newly designed internal primers COIf2-SHL (forward; 5′-ATTTTRATTATTCCTGGRTT-3′) and COIr2-SHL (reverse; 5′-AAYCCYAAAATACCAATTGA-3′). The reaction mixtures were analysed on ABI PRISM 3130xl sequencers after purification with a Big Dye XTerminator Purification Kit (Applied Biosystems). Obtained sequences were aligned and trimmed to exclude the amplification primers in MEGA 6.06 (Tamura et al., Reference Tamura, Stecher, Peterson, Filipski and Kumar2013). The final sequences (1276 bp each) have been deposited in DDBJ/ENA/GenBank databases with accession numbers LC215293–LC215326.
The population genetic structure of the species was analysed using ARLEQUIN 3.5 (Excoffier & Lischer, Reference Excoffier and Lischer2010). We estimated haplotype diversity h (Nei, Reference Nei1987), mean number of pairwise difference π 1 (Tajima, Reference Tajima1983) and nucleotide diversity π 2 (Nei, Reference Nei1987) for each local population. A parsimonious haplotype network was reconstructed using TCS 1.21 (Clement et al., Reference Clement, Posada and Crandall2000). Estimates of pairwise F st and exact test of differentiation (Hudson et al., Reference Hudson, Slatkin and Maddison1992; Raymond & Rousset, Reference Raymond and Rousset1995) were also conducted among the populations of the Lucky Strike, Logatchev and Ashadze vent fields, from which sufficient numbers of individuals could be sequenced. Moreover, the demographic history of the species was inferred by a mismatch distribution analysis (Rogers & Harpending, Reference Rogers and Harpending1992) and by computing Tajima's D (Tajima, Reference Tajima1989) and Fu's Fs (Fu, Reference Fu1997) in ARLEQUIN.
The following material was also investigated based on morphological criteria to verify previous occurrence records of the study species: ~300 specimens from Menez Gwen (SMNH-50960, 89169 and 89175 and Muséum national d'histoire naturelle, MNHN), Lucky Strike (SMNH-21173, 43246, 45411, 50925, 81666, 84610, 89167, 89168, 89182 and 89188 and MNHN), Rainbow (SMNH-50407 and 50409), Snake Pit (MNHN), Logatchev (SMNH-92814, 92830 and 103249) and Ashadze (SMNH-103194–103199, 103204 and 103208–103211). These specimens cover all known localities and populations of the Shinkailepadinae on MAR.
Some specimen lots included post-settlement juveniles with intact protoconchs or larval shells. The diameter of the protoconch, which corresponds to the shell size at settlement, was measured for 12 specimens from Lucky Strike (7 specimens; SMNH-21173 and 84610 and MNHN), Logatchev (2; SMNH-92830) and Ashadze (3; SMNH-103199). The measurement was made with 5 µm precision by tracing the outline of the protoconch using a Nikon SMZ1500 stereomicroscope equipped with a drawing tube (see Fukumori & Kano, Reference Fukumori and Kano2014 for details). More than a hundred pre-hatched larvae were also obtained and measured by opening 23 egg capsules on conspecific adult shells from the Lucky Strike field (SMNH-21173; see Warén & Bouchet, Reference Warén and Bouchet2001: figure 32).
RESULTS
Thirty-four nucleotide sequences of the partial COI gene (1276 bp) were determined for ‘Shinkailepas’ briandi. Each population of the Menez Gwen, Lucky Strike, Logatchev and Ashadze vent fields showed a high haplotype diversity (h = 1.00 ± 0.50) with high numbers of polymorphic sites (35 ≥ K ≥ 2) and mean pairwise differences (π 1 ≥ 2.00), as well as a low nucleotide diversity (π 2 ≤ 0.0065; Table 2). The reconstructed haplotype network consisted of 32 (including two shared and 30 singleton) haplotypes, which differed by one to 13 substitutions from each other (Figure 3). One of the two shared haplotypes originated from the Menez Gwen (38°N; 814–831 m depth) and Ashadze (13°N; 4090 m) vent fields, and the other from Ashadze and Lucky Strike (37°N; 1721 m). Neither the pairwise F st nor the exact test showed significant genetic differentiation among the local populations of Lucky Strike, Logatchev and Ashadze (Table 3).

Fig. 3. Haplotype network for ‘Shinkailepas’ briandi based on 1276-bp sequences of mitochondrial COI gene from 34 individuals. Colour and circle size reflect locality and haplotype frequency, respectively; undiscovered haplotypes are shown as small open circles. Each line represents one mutational step.
Table 2. Genetic diversity of mitochondrial COI sequences in ‘Shinkailepas’ briandi (1276 bp).

N, number of specimens; Nh, number of haplotypes; K, number of polymorphic sites; h, haplotype diversity; π 1, mean number of pairwise differences; π 2, nucleotide diversity.
Table 3. Genetic differentiation tests among three local populations of ‘Shinkailepas’ briandi based on 1276-bp sequences of mitochondrial COI gene (upper right: results of exact test; lower left: pairwise F st values).

None of the values were significant (*P > 0.05).
The mismatch distribution analysis suggested that the panmictic population of ‘S.’ briandi along MAR has experienced a sudden demographic expansion. The observed unimodal distribution with a mean of 7.12 substitutions (Figure 4) was not significantly different from the theoretical distribution under the sudden expansion model (τ = 8.43) with a sum of squared deviations of 0.008 (P = 0.24). The sudden expansion was also suggested by significantly negative (P < 0.05) values of Tajima's D (−1.98) and Fu's Fs (−25.05). By applying the evolutionary rates of COI gene for invertebrates (pairwise distance of 1.4–2.4% per million years; see Wilke, Reference Wilke2003), the population expansion of the species could be calculated to have occurred 0.28–0.48 million years ago (8.43 substitutions in 1276 base pairs).

Fig. 4. Mismatch distribution of ‘Shinkailepas’ briandi based on 1276-bp sequences of mitochondrial COI gene. Bars show observed distribution; line denotes expected distribution under sudden expansion model.
All specimens treated in this study had conchological and anatomical characteristics of the species, as shown for the type specimens in the original description (Warén & Bouchet, Reference Warén and Bouchet2001), although the shell size and colour of mineral deposits on the shell tend to differ among sites (Figure 2). The occurrence of ‘S.’ briandi was thus verified for not only the above four localities but also for the Rainbow and Snake Pit vent sites. No other species was found in our material of the Shinkailepadinae from MAR.
The colourless, multispiral protoconchs of ‘S.’ briandi were fairly constant in size (mean and standard deviation of the diameter: 706 ± 8 µm; range: 695–720 µm). No significant difference was observed among the populations of Lucky Strike (704 ± 7 µm), Logatchev (710 ± 5 µm) and Ashadze (710 ± 9 µm) by one-way ANOVA (P = 0.43). The shell diameter at hatching was estimated to be 170–180 µm, according to the sizes of 24 ready-to-hatch veligers in five egg capsules (two with 180-μm diameter and 22 others with 170 µm).
DISCUSSION
The present study first revealed the genetic population structure of a gastropod species endemic to deep-sea hydrothermal vents along the Mid-Atlantic Ridge. Our analyses based on 1.3-kbp mitochondrial DNA sequences from a total of 34 specimens of ‘Shinkailepas’ briandi showed a panmictic population in the Menez Gwen, Lucky Strike, Logatchev and Ashadze vent fields. These vent fields are separated by up to 3150-km distances and distributed in a depth range of 814–4090 m, collectively covering the geographic and bathymetric distributions of the species (Figure 1). The mismatch distribution analysis and the values of Tajima's D and Fu's Fs suggested that the panmictic population of ‘S.’ briandi has experienced a sudden demographic expansion, which apparently followed a bottleneck event in the last half a million years. A similar demographic history along MAR has previously been suggested for the alvinocaridid shrimp Rimicaris exoculata, with an estimate time of population expansion of 250,000 years ago (Teixeira et al., Reference Teixeira, Serrão and Arnaud-Haond2012). Such bottlenecks could have resulted from unstable and ephemeral hydrothermal activities (Holm & Hennet, Reference Holm, Hennet and Holm1992), although no significant geological record exists for a plausible explanation for either of the limpet or shrimp species (see Teixeira et al., Reference Teixeira, Serrão and Arnaud-Haond2012). Two more species of alvinocaridid shrimps exhibit genetic panmixia along MAR (Teixeira et al., Reference Teixeira, Olu, Decker, Cunha, Fuchs, Hourdez, Serrão and Arnaud-Haond2013).
The present limpet species develops as a long-lived planktotrophic larva (Warén & Bouchet, Reference Warén and Bouchet2001; Fukumori & Kano, Reference Fukumori and Kano2014), as alvinocaridid shrimps do (Ramirez Llodra et al., Reference Ramirez Llodra, Tyler and Copley2000; Young, Reference Young and Tyler2003). Whereas in-situ sampling has never been conducted to elucidate the larval behaviour of the limpet, an unidentified, vent-endemic alvinocaridid shrimp has been collected as a pelagic larva at a depth of 800–1000 m, which is ~1000 m above nearby vent sites (Herring, Reference Herring2006). Stable isotopic analyses of post-settlement larvae of confamilial shrimps likewise suggest dependence on photosynthetic nutrition in their pelagic life (Stevens et al., Reference Stevens, Limén, Pond, Gélinas and Juniper2008). The alvinocaridid larvae are thus thought to feed and disperse in mid-water, far above the influence of a hydrothermal plume, which then might have resulted in their high population connectivity and wide geographic distributions (Teixeira et al., Reference Teixeira, Cambon-Bonavita, Serrão, Desbruyéres and Arnaud-Haond2011; see also Beedessee et al., Reference Beedessee, Watanabe, Ogura, Nemoto, Yahagi, Nakagawa, Nakamura, Takai, Koonjul and Marie2013).
The high genetic connectivity of ‘S.’ briandi among the horizontally and vertically scattered vent habitats most probably resulted from a similar large-scale dispersal of larvae in a water column extraneous to hydrothermal vents. Most interestingly, their newly settled juveniles bear a pair of black eyespots, which are lost in larger individuals (Warén & Bouchet, Reference Warén and Bouchet2001). The retention of eyes in the juveniles of deep-sea gastropods might suggest their vertical migration as swimming larvae to the photic zone (Bouchet & Warén, Reference Bouchet, Warén, Young and Eckelbarger1994). Such vertical migration has actually been demonstrated – by collecting larvae from the surface water – for the confamilial Thalassonerita naticoidea (= Bathynerita naticoidea; Fukumori et al., in preparation) that inhabits cold methane seeps at depths from 400 to 1700 m in the Caribbean region (Arellano et al., Reference Arellano, Van Gaest, Johnson, Vrijenhoek and Young2014). Converging evidence from larval behaviour, temperature optimum and population genetics indicates that this is also the case for a vent-endemic species of the family. Shinkailepas myojinensis in the north-west Pacific (442–1227 m depth) arguably takes advantage of richer nutrition and stronger currents in the surface water (Yahagi et al., Reference Yahagi, Kayama Watanabe, Kojima and Kano2017).
The planktotrophic larvae of T. naticoidea and S. myojinensis are thought to spend at least 7–12 months before settlement (Arellano et al., Reference Arellano, Van Gaest, Johnson, Vrijenhoek and Young2014; Yahagi et al., Reference Yahagi, Kayama Watanabe, Kojima and Kano2017). The settlement size of planktotrophic neritimorphs is remarkably stable within a species (Kano, Reference Kano2006) and it is probably not a coincidence that these phenacolepadids with such a long larval phase are larger at settlement than most other members of the subclass (Fukumori & Kano, Reference Fukumori and Kano2014: table 1). The diameter of their shells increases significantly from 170 µm at hatching to 670–680 μm at settlement in T. naticoidea (Arellano et al., Reference Arellano, Van Gaest, Johnson, Vrijenhoek and Young2014: figure 1) or from 140–160 µm to 720 µm in S. myojinensis (Fukumori & Kano, Reference Fukumori and Kano2014; Yahagi et al., Reference Yahagi, Kayama Watanabe, Kojima and Kano2017). ‘Shinkailepas’ briandi has comparable sizes at both hatching (170–180 µm) and settlement (695–720 µm), implying a similarly long pelagic period for this species. It is also crucial to note that neritimorph larvae have an ability to remain pelagic for a few or several months after attaining the settlement size, if a suitable settlement cue is not found (Scheltema, Reference Scheltema1971; Lesoway & Page, Reference Lesoway and Page2008; Fukumori & Kano, Reference Fukumori and Kano2014). The panmictic population of ‘S.’ briandi along MAR has resulted from effective dispersal by their long-lived planktotrophic larvae, which likely migrate to and grow in the surface water, regardless of the deeper occurrences of their adults, hence a longer distance and duration of vertical migration than T. naticoidea and S. myojinensis.
Growing evidence suggests that other vent endemic animals, including at least some alvinocaridid shrimps and bythograeid crabs, also migrate not just to mid-water column but to the euphotic layer as planktotrophic larvae (Yahagi et al., Reference Yahagi, Kayama Watanabe, Kojima and Kano2017). On the other hand, not all planktotrophs experience the warm, phytoplankton-rich surface water (see Young, Reference Young and Tyler2003 for review). The planktotrophic mussels of the genus Bathymodilus on MAR, and their commensal annelid with lecithotrophic development, are each composed of multiple lineages that are divided by a dispersal barrier (Jollivet et al., Reference Jollivet, Empis, Baker, Hourdez, Comtet, Jouin-Toulmond, Desbruyères and Tyler2000; O'Mullan et al., Reference O'Mullan, Maas, Lutz and Vrijenhoek2001; Breusing et al., Reference Breusing, Biastoch, Drews, Metaxas, Jollivet, Vrijenhoek, Bayer, Melzner, Sayavedra, Petersen, Dubilier, Schihabel, Rosentiel and Reusch2016). The vertical migration to the surface might perhaps be hampered by the physiological preference and/or tolerance of larvae in Bathymodilus. Planktotrophic larvae of another bathymodioline species in the Gulf of Mexico have been experimentally shown to have a lower thermal tolerance than those of the phenacolepadid T. naticoidea (Arellano et al., Reference Arellano, Van Gaest, Johnson, Vrijenhoek and Young2014). Future studies on larval behaviour and temperature optimum for more numerous species would enable to evaluate this hypothesis and shed further light on the biogeography of the vent endemic fauna.
ACKNOWLEDGEMENTS
We thank M. Segonzac and R. Vrijenhoek, as well as chief scientists who led French expeditions to MAR, for providing the specimens of ‘S.’ briandi. We are also grateful to P. Bouchet and V. Héros for assisting us in the study of the material deposited in MNHN. Invaluable comments on the manuscript were made by C. Young and an anonymous reviewer.
FINANCIAL SUPPORT
This work was supported financially by JSPS KAKENHI (grant numbers 15J08646 and 15H04412).