Introduction
A tooth trace on the surface of a fossil bone is merely evidence that an interaction took place between the two. However, the behavior that such a trace may represent is up for interpretation. In general, tooth–bone contacts may result from various behaviors. Fighting carnivores may bite hard enough to gouge the bones of their adversaries during nonpredatory biting. Such fight-induced bites may occur in particular areas, such as the head and tail (see Webb et al., Reference Webb, Manolis and Buckworth1983; Tanke and Currie, Reference Tanke and Currie1998; Avilla et al., Reference Avilla, Fernandes and Ramos2004; Katsura, Reference Katsura2004; Bell and Currie, Reference Bell and Currie2010; Zammit and Kear, Reference Zammit and Kear2011). Signs of tissue recovery (healing) may aid nonfeeding interpretation of such bite traces (see, e.g., Tanke and Currie, Reference Tanke and Currie1998; Drumheller et al., Reference Drumheller, Stocker and Nesbitt2014, Reference Drumheller, McHugh, Kane, Riedel and D'Amore2020).
Tooth traces may form on bones due to predatory behaviors, mainly prey capturing and killing (see, e.g., Schaller and Vasconcelos, Reference Schaller and Vasconcelos1978; Njau and Blumenschine, Reference Njau and Blumenschine2006). However, many traces on bones represent postmortem feeding behaviors associated with various phases of food processing, such as gross reduction of the corpse (dismembering) and bone defleshing (Njau and Blumenschine, Reference Njau and Blumenschine2006; D'Amore and Blumenschine, Reference D'Amore and Blumenschine2009; Westaway et al., Reference Westaway, Thompson, Wood and Njau2011; Njau and Gilbert, Reference Njau and Gilbert2016).
Therefore, the teeth may gouge the bones during sarcophagy, and by escaping the swallowing and subsequent ingestion, some of the tooth-marked bones may be abandoned. Those have a potential to preserve bite traces and convey behavioral information into the fossil record (see, e.g., Erickson and Olson, Reference Erickson and Olson1996; Boaz et al., Reference Boaz, Ciochon, Xu and Liu2000; Rogers et al., Reference Rogers, Krause and Rogers2003; Njau and Blumenschine, Reference Njau and Blumenschine2006; Pobiner, Reference Pobiner2008; Jacobsen and Bromley, Reference Jacobsen and Bromley2009; Bianucci et al., Reference Bianucci, Sorce, Storai and Landini2010; Bell et al., Reference Bell, Currie and Lee2012).
Although ichnology has experienced progress in the naming of fossilized bite traces on bones (e.g., Mikuláš et al., Reference Mikuláš, Kadlecová, Fejfar and Dvořák2006; Jacobsen and Bromley, Reference Jacobsen and Bromley2009) and in their behavioral interpretations (see, e.g., Bell and Currie, Reference Bell and Currie2010; Longrich et al., Reference Longrich, Horner, Erickson and Currie2010; Hone and Chure, Reference Hone and Chure2018; Scheyer et al., Reference Scheyer, Delfino, Klein, Bunbury, Fleischer-Dogley and Hansen2018; Drumheller et al., Reference Drumheller, McHugh, Kane, Riedel and D'Amore2020), there have been no similar advances in the ichnological scheme of their ethological classifications. Therefore, despite the interpretative uncertainties associated with ethology of such trace fossils, all have been traditionally classified as predichnia—traces of predation (see definition in Ekdale, Reference Ekdale1985; see Vallon et al., Reference Vallon, Rindsberg and Bromley2016 and references therein). Such classification of a bite trace implies it is evidence of a predator–prey interaction. However, this is not necessarily the case.
The present paper discusses examples of bite traces preserved on bone fragments recovered from the continental Upper Triassic (Norian) Grabowa Formation from southern Poland and provides two new terms to aid in future ethological classification of such bite traces (sarcophagichnia and necrophagichnia). The analysis and discussion provided in the present paper focus on morphological features (serration/striations in Linichnus) of the ichnites, their potential trace makers, feeding strategies, and postmortem food-processing behaviors that the trace fossils may represent.
Geological settings
Location and stratigraphy
The bite traces described in this paper occur on fragmentary fossil bones recovered from the Upper Triassic deposits exposed in a site close to Zawiercie, a city in southern Poland (Fig. 1, Table 1). The area was part of the Late Triassic sedimentary basin located on the northern shore of the Tethys Ocean (see Szulc, Reference Szulc2007b; McKie and Williams, Reference McKie and Williams2009; Jewuła et al., Reference Jewuła, Matysik, Paszkowski and Szulc2019).

Figure 1. The Zawiercie site in southern Poland. The figure shows generalized stratigraphy of the Upper Triassic strata in the area—grayed members of the Grabowa Formation constitute the Norian profile of Zawiercie (based on Szulc et al., Reference Szulc, Gradziński, Lewandowska and Heunisch2006, Reference Szulc, Racki and Jewuła2015; Szulc and Racki, Reference Szulc and Racki2015).
Table 1. Summary of studied bite traces.
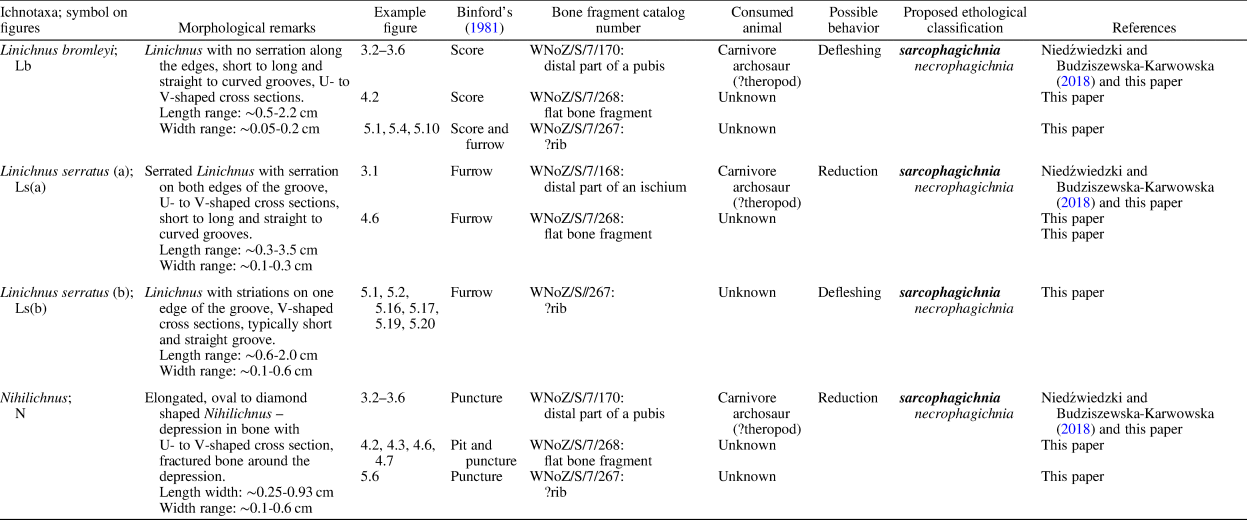
The deposits at the site in Zawiercie represent the continental Grabowa Formation (Fig. 1), which is sandwiched between two informal units (both in a rank of formation): “Bolesław” (below) and “Połomia” (above; see Szulc and Racki, Reference Szulc and Racki2015; Szulc et al., Reference Szulc, Racki and Jewuła2015). The lower part of the sequence is mud dominated and represents the Patoka Member. This unit grades upward into the Woźniki Limestone Member, which is distinguished by the presence of thicker carbonate beds (see Szulc and Racki, Reference Szulc and Racki2015; Szulc et al., Reference Szulc, Racki and Jewuła2015). The sedimentary sequence at the site is dominated by freshwater calcareous gray and reddish mudstones. The sequence comprises also subordinate, coarser grained deposits, including sand- and gravel-grade facies (see Szulc et al., Reference Szulc, Gradziński, Lewandowska and Heunisch2006; Szulc and Racki, Reference Szulc and Racki2015; Szulc et al., Reference Szulc, Racki and Jewuła2015).
The deposits at the site in Zawiercie comprise microfossils characteristic for the Polish Upper Triassic, namely components of the palynological zone IVb (see Orłowska-Zwolińska, Reference Orłowska-Zwolińska1983 for details). This assemblage and correlation with other sites in the formation indicate that the sediments from Zawiercie are Norian (see e.g., Szulc et al., Reference Szulc, Gradziński, Lewandowska and Heunisch2006; Szulc, Reference Szulc, Szulc and Becker2007a; Fijałkowska-Mader et al., Reference Fijałkowska-Mader, Heunisch and Szulc2015; Szulc et al., Reference Szulc, Racki and Jewuła2015).
Paleoenvironment
Central Europe experienced pluvialization during the Carnian–Norian transition, and that climatic shift reflected in the dominant facies: evaporates (representing playas of Carnian age) were replaced by sediments of fluvial channels and lakes, all deposited in Norian wetlands (Szulc et al., Reference Szulc, Gradziński, Lewandowska and Heunisch2006). The latter were formed under the seasonal semi-arid to subhumid Norian climate (see Szulc et al., Reference Szulc, Gradziński, Lewandowska and Heunisch2006; Szulc, Reference Szulc2007b; Gruszka and Zieliński, Reference Gruszka and Zieliński2008; McKie and Williams, Reference McKie and Williams2009; Fijałkowska-Mader et al., Reference Fijałkowska-Mader, Heunisch and Szulc2015; Szulc et al., Reference Szulc, Racki and Jewuła2015; Jewuła et al., Reference Jewuła, Matysik, Paszkowski and Szulc2019).
The fine, mud-dominated facies exhibit the deposition of particles suspended within still waters. However, the coarser facies (sand and gravel) had to be deposited by flowing water and some—especially gravel-grade deposits—indicate flooding events (see, e.g., Szulc et al., Reference Szulc, Gradziński, Lewandowska and Heunisch2006; Jewuła et al., Reference Jewuła, Matysik, Paszkowski and Szulc2019; Sadlok, Reference Sadlok2020).
Materials and methods
The following subsections describe the type and provenience of the fossilized material and methods utilized in the present study, including details of cut tests (see Fig. 2).

Figure 2. The cut tests: resulting trace morphologies versus blade kinetics. (1) Serrated blade representing a tooth in this test (blade was hand held). (2–13) Results for different blade kinetics. (2, 5, 8, 11) Kinetics of blade in respect to the substrate (shown in gray): side and top views during interaction are illustrated. Two types of substrates, differing in plasticity, were used with comparable results: (3, 6, 9, 12) polyvinyl chloride (plasticized); (4, 7, 10, 13) polypropylene (not plasticized). (3, 4) Cut morphologies resulting from kinetics depicted in (2). (6, 7) Cut morphologies resulting from kinetics depicted in (5). (9, 10) Cut morphologies resulting from kinetics depicted in (8). (12, 13) Cut morphologies resulting from kinetics depicted in (11). nst = no striations; com = chipped-off material; st = striations; rm = removed material.
Materials
All the illustrated specimens (Figs. 3–5) were collected at the pail of sediments left after the construction of a local city dump (see also Budziszewska-Karwowska et al., Reference Budziszewska-Karwowska, Bujok and Sadlok2010; Niedźwiedzki and Budziszewska-Karwowska, Reference Niedźwiedzki and Budziszewska-Karwowska2018; Sadlok, Reference Sadlok2020).

Figure 3. Bite trace fossils on pelvic girdle components: traces of feeding on carnivorous archosaurs. (1) An ischium fragment (distal part; specimen WNoZ/S/7/168) of a carnivorous archosaur (?theropod; see Niedźwiedzki and Budziszewska-Karwowska, Reference Niedźwiedzki and Budziszewska-Karwowska2018). (2) Pubic bone fragment (distal part; specimen WNoZ/S/7/170) of a carnivorous archosaur (?theropod; see Niedźwiedzki and Budziszewska-Karwowska, Reference Niedźwiedzki and Budziszewska-Karwowska2018). (3–6) Magnified details of pubic bone surface from (2): (3–5) Linichnus bromleyi; (6) Nihilichnus. Ls(a) = Linichnus serratus (a); Lb = Linichnus bromleyi; N = Nihilichnus; fr = bone fracturing around the trace.

Figure 4. Multiple bite trace fossils on a flat bone fragment (specimen WNoZ/S/7/268). (1, 5) Two opposite sides of the bone specimen (dashed lines around the fragment depict those parts of its margins where the bone is missing). (2, 3) Enlarged views of areas shown in (1). (4) Sectional view of the specimen (along the fracture); light-colored calcite cementation is visible. (6) Enlarged view of area shown in (5). (7) Enlarged area from (6). Note various sizes of the bites (compare (2) and (6)). Lb = Linichnus bromleyi; Ls(a) = Linichnus serratus (a); N = Nihilichnus; cem = cement (calcite); fr = bone fracturing around the trace; s = sediment-infilled breakage of bone (?natural).

Figure 5. Multiple bite trace fossils on a ?rib fragment (specimen WNoZ/S/7/267). (1–15) Various views (360° rotation) of the specimen (bite traces occur on all surfaces). Short dashed lines used in places illustrate axes of the traces (parallel axes may result from a single bite). Note various sizes and orientations of the bite traces and their cross cutting. (16, 17) Two views (rotation applied to illuminate/shadow two sides of the plasticine cast made of trace boxed in (2)). (18) Drawing based on (16, 17) (dashed line depicts the trace axis). (19, 20) Two views (rotation applied to illuminate/shadow two sides of the plasticine cast made of trace boxed in (2)). (21) Drawing based on (19, 20) (dashed line depicts the trace axis). Ls(b) = Linichnus serratus (b); Lb = Linichnus bromleyi; N = Nihilichnus; x = cross-cutting bite traces.
The bones
The bite-bearing bones are fragmentary, and their proper identification is problematic (see Table 1). The taxonomy of specimens illustrated in Figure 3 has been studied by Niedźwiedzki and Budziszewska-Karwowska (Reference Niedźwiedzki and Budziszewska-Karwowska2018). Those authors concluded that the bones likely represented carnivorous archosaurs—potentially theropods (compare Dzik et al., Reference Dzik, Sulej and Niedźwiedzki2008; Niedźwiedzki et al., Reference Niedźwiedzki, Sulej and Dzik2011; Niedźwiedzki and Budziszewska-Karwowska, Reference Niedźwiedzki and Budziszewska-Karwowska2018). According to Niedźwiedzki and Budziszewska-Karwowska (Reference Niedźwiedzki and Budziszewska-Karwowska2018), these bone fragments were parts of pelvic girdles, namely, a distal part of an ischium shaft (Fig. 3.1) and a pubic boot (Fig. 3.2–3.6).
Figures 4 and 5 show fragmentary bones of unknown affinities (see Table 1); one is a flat bone fragment (Fig. 4) and the other may represent a short fragment of a rib (Fig. 5).
Methods
The analyzed bite traces were measured with a caliper to provide a range of observed sizes (lengths and widths; see Table 1 and supplementary materials). Bone specimens bearing the bite traces were photographed with a Canon DS126491 (18-55 lens) and presented in grayscale in Figures 3–5. A few close-up photographs were taken with a Leica Wild L10 microscope equipped with a NikCam Pro camera to compose Figure 5.16, 5.17, 5.19, 5.20.
No heavy preparatory work was done in the field or laboratory that could end in formation of artificial tool-induced bone surface modifications. Also, the Triassic age of the material excluded the need for cut–bite mark differentiation (compare Blumenschine et al., Reference Blumenschine, Marean and Capaldo1996). All the figures were prepared with Gimp and Inkscape software (Kimball et al., Reference Kimball and Mattis2008; Albert et al., Reference Albert2010).
Cutting tests were performed with a serrated steel blade and two types of polymeric substrate: plasticized (polyvinyl chloride) and nonplasticized (polypropylene). The knife was handheld during the tests (see inset drawing in Fig. 2.1). The cuts were easily produced in room temperature, and no excessive force was required to mark the substrates (polypropylene was harder to cut with the blade than polyvinyl chloride). Figure 2 summarizes the information on used substrates, blade size (see Fig. 2.1), applied kinetics during the blade–substrate contacts (see Fig. 2.2, 2.5, 2.8, 2.11), and resulting cut-mark morphologies (see Fig. 2.3, 2.4, 2.6, 2.7, 2.9, 2.10, 2.12, 2.13).
The aim of the test was to approximate the possible tooth kinetics responsible for bite formations. Therefore, only a simply flat blade with a well-defined edge morphology was used during the tests (9 serrations/cm). One blade was used in all the tests (Fig. 2.1), and only a single sequence of movements (without repetition) was used to obtain each cut (as shown on Fig. 2.2, 2.5, 2.8, 2.11). The test results were particularly useful in analysis and discussion of the modes that serration and striations could form along Linichnus bite traces studied in the present work (Fig. 2; see also Bianucci et al., Reference Bianucci, Sorce, Storai and Landini2010; Muñiz et al., Reference Muñiz, Belaústegui, Toscano, Ramirez-Cruzado and Vintaned2020).
Repository and institutional abbreviation
The material is part of a collection hosted by the Museum of Earth Sciences at the University of Silesia in Sosnowiec, Poland (see Table 1 and figure captions for specimen catalog numbers; collection abbreviation: WNoZ).
Results
The following subsections describe the bite traces encountered on the analyzed fossil bones.
The bite traces on bones
The studied bite traces display morphologies falling into four main categories of bone modifications recognized by Binford (Reference Binford1981) in his taphonomic studies: pits and scores (shallow surficial modifications) and punctures and furrows (deeper modifications; see Binford, Reference Binford1981; D'Amore and Blumenschine, Reference D'Amore and Blumenschine2009). These terms are widely used in taphonomic literature (see, e.g., Njau and Blumenschine, Reference Njau and Blumenschine2006; D'Amore and Blumenschine, Reference D'Amore and Blumenschine2009; Drumheller and Brochu, Reference Drumheller and Brochu2014, Reference Drumheller and Brochu2016; Njau and Gilbert, Reference Njau and Gilbert2016; Drumheller et al., Reference Drumheller, McHugh, Kane, Riedel and D'Amore2020; Drymala et al., Reference Drymala, Bader and Parker2021). Therefore, the following descriptions and Table 1 include them to indicate the relationships between the taphonomic terminology and the ichnotaxa.
Linichnus ichnogenus
A single, elongated biogenic groove (score or furrow) on skeletal material (e.g., bones, teeth). The groove is U- or V-shaped in its transverse section. It may be shallow, affecting only the bone surface, or deeper, cutting the bone-fibers, which may become recurved or broken (see Jacobsen and Bromley, Reference Jacobsen and Bromley2009). The original diagnosis of this ichnogenus also contained information on serrated edges of the groove (see Jacobsen and Bromley, Reference Jacobsen and Bromley2009). However, since Muñiz et al. (Reference Muñiz, Belaústegui, Toscano, Ramirez-Cruzado and Vintaned2020) established Linichnus bromleyi, the edge serration has become a distinguishing feature at the ichnospecific rather than ichnogeneric level. Currently, there are two ichnospecies within Linichnus: L. serratus (Jacobsen and Bromley, Reference Jacobsen and Bromley2009) and L. bromleyi (Muñiz et al., Reference Muñiz, Belaústegui, Toscano, Ramirez-Cruzado and Vintaned2020). The first one has serrated edges, whereas the edges of L. bromleyi are smooth (compare Jacobsen and Bromley, Reference Jacobsen and Bromley2009; Muñiz et al., Reference Muñiz, Belaústegui, Toscano, Ramirez-Cruzado and Vintaned2020).
Description of Linichnus traces
Table 1 provides a brief descriptive summary for the studied Linichnus traces. The scores and furrows on bone ascribed herein to Linichnus (Figs. 3–5) are variably elongated, straight to curved. In some instances, they appear to form sets of parallel traces (marked with dashed lines in Fig. 5). Their cross sections are U- to V-shaped (observed on casts made with modeling clay/plasticine). The sizes are also variable. Lengths range from a few millimeters to ~3.5 cm (measured on a convex bone surface). Widths range from submillimeter to ~5.6 mm. The depths vary as well and range from submillimeter to ~1 mm (estimated from plasticine casts). The wider grooves are also deeper (e.g., compare Figs. 3.1 and 4.2). Two ichnospecies of the ichnogenus are present in the material. For example, Figures 3.1 and 4.6 show L. serratus whereas Figures 3.2–3.5 and 4.2 illustrate L. bromleyi.
The studied L. serratus comprises two morphological variants, labeled here as L. serratus (a) (e.g., Figs. 3.1, 4.6) with serration along both edges and L. serratus (b) (Fig. 5.1, 5.16–5.21) with striations along one edge only (compare this morphology with the Type I bite trace of Collareta et al., Reference Collareta, Lambert, Landini, Di Celma, Malinverno, Varas-Malca, Urbina and Bianucci2017). The latter variant is distinct and deserves a more detailed description.
These traces are straight and typically short with their sectional views resembling a wide-gaping “V”; both sides of the “V” sink into the bone at slight angles. However, one of the sides is typically steeper than the other and shows some fine, short striations emanating from the groove (see Fig. 5.1, 5.2, 5.19–5.21). These fine features are approximately perpendicular to the summit line between the two V-forming surfaces (see Fig. 5.16–5.21). The overall outline of these L. serratus (b) traces may resemble a teardrop, with one end of the groove being slightly wider than the other (see Fig. 5.16–5.18). The overall morphology (short, straight grooves having V-shaped cross sections) of these traces resembles the so-called edge marks that Komodo monitors may produce on bone edges (D'Amore and Blumenschine, Reference D'Amore and Blumenschine2009). The edge marks, contrary to the present L. serratus (b), rarely display striations. They occur on narrow edges of bones, being approximately transverse to those edges (see D'Amore and Blumenschine, Reference D'Amore and Blumenschine2009, Reference D'Amore and Blumenschine2012).
Nihilichnus ichnogenus
Triangular to ovoid holes or external pits or punctures. They occur as individual traces or may form groups; the grouping may be a recurring pattern. The margin of individual pits/punctures shows irregular jags, resulting from a brittle deformation (see Mikuláš et al., Reference Mikuláš, Kadlecová, Fejfar and Dvořák2006). The ichnogenus comprises one ichnospecies, Nihilichnus nihilicus Mikuláš et al., Reference Mikuláš, Kadlecová, Fejfar and Dvořák2006, sharing the diagnostic features with the ichnogenus (Mikuláš et al., Reference Mikuláš, Kadlecová, Fejfar and Dvořák2006).
Description of Nihilichnus traces
Table 1 provides a brief description summary for the studied Nihilichnus. The trace fossils ascribed here to the ichnogenus (Figs. 3.2, 3.6, 4.1, 4.3, 4.6, 4.7, 5.4, 5.6) are pits/punctures made in bone surfaces. Their outlines are elongated, oval to slightly diamond-like in shape (compare Fig. 3.6 with 4.3, 5.4). The maximal measured dimensions of the traces range from ~2.5 to ~9.3 mm. The marginal fracturing (jags) is well visible in some of the traces (see Figs. 3.6, 4.4). The sections of Nihilichnus traces are U- to (Fig. 5.14) slightly V-shaped (Figs. 4.1, 5.4; profiles observed with casts of modeling clay/plasticine); some traces are partially filled with sediment that masks their full sectional morphology (e.g., Fig. 4.3).
Results of cutting tests
The cutting tests were conducted with a serrated blade and two types of synthetic substrates (see Methods). The results of these tests are shown in Figure 2. Only the blade edge–substrate contacts were studied, and therefore the results may be relevant only for some of the studied Linichnus traces (see Serration and striations of Linichnus).
The cuts illustrated in Figure 2.3, 2.4 resulted from the blade edge penetrating the substrate and passing through (Fig. 2.2). Figure 2.6, 2.7 shows cuts that resulted from the blade entering the substrate (parallel to denticulated edge), stopping within the substrate, and then lifting off, without further surface modification (Fig. 2.5). The cuts in Figure 2.9, 2.10, 2.12, 2.13 resulted from the blade entering the substrate as in Figure 2.3, 2.4, 2.6, 2.7. The blade stopped in these cases (see Fig. 2.9, 2.10, 2.12, 2.13) within the substrate (as in Fig. 2.6, 2.7) but then left it with a sideways movement (Fig. 2.8, 2.11; perpendicular to the denticulated edge). The cuts from Figure 2.9, 2.10, 2.12, 2.13 differ from each other in the angles at which the blade entered and left the substrate. Figure 2.12, 2.13 depicts lower angles (see also Erickson and Olson, Reference Erickson and Olson1996 for comments on tooth–bone contact angle).
Discussion
Serration and striations of Linichnus
Jacobsen and Bromley (Reference Jacobsen and Bromley2009) used the term “serration” to describe both uneven edges in Linichnus and parallel grooves forming Knethichnus. D'Amore and Blumenschine (Reference D'Amore and Blumenschine2009, Reference D'Amore and Blumenschine2012) used the term “striations” to label grooves of Knethichnus-like traces. They did not provide any specific term to describe uneven edges of Linichnus-like traces. D'Amore and Blumenschine (Reference D'Amore and Blumenschine2012) used “striation” also to label sets of grooves associated with short Linichnus-like traces (their edge marks). Forrest (Reference Forrest2003) used the term “striations” to name grooves covering the surface of purported tooth trace left by marine crocodile Metriorhynchus. Those grooves, however, were thought to match ridges running from tooth tip toward its base (see Forrest, Reference Forrest2003). Therefore, Forrest's (Reference Forrest2003) usage of “striations” was different from that of D'Amore and Blumenschine (Reference D'Amore and Blumenschine2009, Reference D'Amore and Blumenschine2012), and their usage did not match exactly with that of Jacobsen and Bromley (Reference Jacobsen and Bromley2009).
Although the meanings of “serration” and “striations” overlap to some degree, these are not complete synonyms. Therefore, I use both terms in the following. However, my usage of “serration” is restricted to uneven edges of Linichnus (as in Jacobsen and Bromley, Reference Jacobsen and Bromley2009) whereas “striations” applies to sets of elongated grooves (as in D'Amore and Blumenschine, Reference D'Amore and Blumenschine2009).
Jacobsen (Reference Jacobsen1998, Reference Jacobsen2003) showed that serration and striations of bite traces and tooth denticles may be matched (in terms of size and density), in some cases aiding in identification of carnivorous species that could have made the bite traces. This link between the tooth denticles and serration/striations of a trace has been highlighted also in other works (e.g., Alexander and Burger, Reference Alexander, Burger and Gunnell2001; Jacobsen and Bromley, Reference Jacobsen and Bromley2009; Bell et al., Reference Bell, Currie and Lee2012; see also D'Amore and Blumenschine, Reference D'Amore and Blumenschine2012; Drumheller et al., Reference Drumheller, McHugh, Kane, Riedel and D'Amore2020).
D'Amore and Blumenschine (Reference D'Amore and Blumenschine2009) showed that scores were the most frequent (81%) type of bone modifications by Komodo monitors (reptile with ziphodont teeth) in their actualistic study. They concluded also that only 5% of bite traces had striations. Komodo monitors do have distinct tooth denticles on both mesial and distal cutting edges of their teeth. The strong backward tooth curvature makes the contact of their mesial carinae with bone surface very likely; indeed, denticles in these parts of carinae appear particularly worn down (see D'Amore and Blumenschine, Reference D'Amore and Blumenschine2009, Reference D'Amore and Blumenschine2012). Interestingly, this actualistic study showed that individual bite traces made with denticulated ziphodont teeth are likely to show no striations at all, and a larger sample may be required to detect their presence. D'Amore and Blumenschine (Reference D'Amore and Blumenschine2009, e.g., fig. 6A) illustrated some scores with uneven edges, features resembling serration of Linichnus trace fossils (sensu Jacobsen and Bromley, Reference Jacobsen and Bromley2009), but no details were provided on frequency of those features.
Muñiz et al. (Reference Muñiz, Belaústegui, Toscano, Ramirez-Cruzado and Vintaned2020) contributed experimental data and showed that when a denticulated tooth edge cuts through the substrate (moving along its denticulated edge), irregular serration forms on both sides of the emerging Linichnus-like trace. Muñiz et al. (Reference Muñiz, Belaústegui, Toscano, Ramirez-Cruzado and Vintaned2020) illustrated results of cutting tests performed with one kinetic (edge-parallel) shark tooth and plaster serving as a substrate. Bianucci et al. (Reference Bianucci, Sorce, Storai and Landini2010) used plasticine instead of plaster and different tooth kinetics, including one that was edge perpendicular.
The results presented herein (Fig. 2) match those of Bianucci et al. (Reference Bianucci, Sorce, Storai and Landini2010) in terms of: (1) missing striation in a trace produced via a tooth edge-parallel kinetics and (2) striations/irregular grooves formed within the trace made with the edge-perpendicular tooth kinetics; denticles are not mandatory to form such irregular grooves as the tooth edge imperfections may cause them to form (see Bianucci et al., Reference Bianucci, Sorce, Storai and Landini2010).
The results presented here match also with the results of D'Amore and Blumenschine (Reference D'Amore and Blumenschine2009, Reference D'Amore and Blumenschine2012) where the striations required a deviation from the tooth-edge-parallel kinetics to form in bite traces of Komodo monitors. The study of bite traces made by Komodo monitors showed also that smooth transition from the tooth-edge-perpendicular to tooth-edge-parallel kinetics results in a set of distinct striations converging into a single score, and an overall branching pattern is the result (see D'Amore and Blumenschine, Reference D'Amore and Blumenschine2012).
The edge-parallel tooth kinetics (Fig. 2.2–2.7) failed to replicate serration observed by Muñiz et al. (Reference Muñiz, Belaústegui, Toscano, Ramirez-Cruzado and Vintaned2020). This is most likely due to different substrates applied in both their tests and those in the present work. Overall, the blade traces obtained during this study are similar (Fig. 2) for both applied types of substrates, despite the differences in their plasticity. Muñiz et al. (Reference Muñiz, Belaústegui, Toscano, Ramirez-Cruzado and Vintaned2020) used plaster, and therefore it is likely that the serration formed in their tests along edges of Linichnus-like groove due to the brittle nature of the substrate they used.
Although it may be unclear how the denticulated edge creates serration along the sides of Linichnus during tooth edge–bone contact (as in Muñiz et al., Reference Muñiz, Belaústegui, Toscano, Ramirez-Cruzado and Vintaned2020), the way denticles modify the bone is well understood in Knethichnus (see D'Amore and Blumenschine, Reference D'Amore and Blumenschine2009, Reference D'Amore and Blumenschine2012; Jacobsen and Bromley, Reference Jacobsen and Bromley2009; Drumheller et al., Reference Drumheller, McHugh, Kane, Riedel and D'Amore2020). It is a trace fossil formed by dragging a tooth along the bone surface; the tooth movement must not be parallel to its denticulated edge (see D'Amore and Blumenschine, Reference D'Amore and Blumenschine2012). This way a characteristic regular morphology forms: densely spaced grooves of comparable sizes (see Rogers et al., Reference Rogers, Krause and Rogers2003; D'Amore and Blumenschine, Reference D'Amore and Blumenschine2009, Reference D'Amore and Blumenschine2012; Jacobsen and Bromley, Reference Jacobsen and Bromley2009). Fine striations may form in bone due to similar kinetics of denticle-free teeth (result of edge defects), but they are irregular and thus distinct from Knethichnus (see Bianucci et al., Reference Bianucci, Sorce, Storai and Landini2010). In the following, I consider two distinct types of tooth–bone interactions that may lead to Linichnus formation: tooth tip–bone contacts and tooth edge–bone contacts.
Tooth tip–bone contact
Some authors have depicted strongly curved (U-shaped grooves), elongated bite traces (hook scores) made by recent crocodylian species (see what are crocodylian taxa in Brochu, Reference Brochu2003, and for their various bit traces, see Njau and Blumenschine, Reference Njau and Blumenschine2006; Drumheller and Brochu, Reference Drumheller and Brochu2014; Njau and Gilbert, Reference Njau and Gilbert2016). The curved morphology of those hook scores is analogical to that of Linichnus serratus holotype, which is a groove following a U-shaped trajectory (see Jacobsen and Bromley, Reference Jacobsen and Bromley2009, fig. 2B, C). The teeth making those crocodylian traces (or the one that produced the Linichnus holotype) had to change movement direction when gouging the bone to follow their U-like trajectories. A plausible interpretation is that those crocodylian traces (and the holotype of Linichnus) might have resulted from an interaction between the tooth tip and bone.
Tooth tip–bone contact would most likely grant higher freedom of movement than the tooth edge–bone contact, for which any deviation from tooth edge-parallel movement would result in Knethichnus morphology rather than Linichnus (see D'Amore and Blumenschine, Reference D'Amore and Blumenschine2012). Therefore, Linichnus resulting from tooth tip–bone contact could display more curved or even winding trajectories of the groove (see Fig. 4.2).
The U-shaped cross section should also aid in differentiation between traces made with tooth tip and those produced with a tooth's narrow cutting edge (see Fig. 4.6). This is because a sharp-edged tool cuts bone forming a narrow groove (V-shaped cross sections), whereas the action of a blunt cutting tool results in a wider groove (U-shaped cross section; Fig. 6.1; see Mate-Gonzalez et al., Reference Mate-Gonzalez, Palomeque-Gonzalez, Yravedra, Gonzalez-Aguilera and Dominguez-Rodrigo2016).

Figure 6. Tooth kinetics and plausible bite trace morphologies. (1) Linichnus may form due to both tooth tip–bone and tooth edge–bone interaction. Curved versus straight Linichnus trajectories and their cross-sectional geometries (see cut cross sections in Mate-Gonzalez et al., Reference Mate-Gonzalez, Palomeque-Gonzalez, Yravedra, Gonzalez-Aguilera and Dominguez-Rodrigo2016) may aid in differentiation between the two contact scenarios. The edge mark is a type of short Linichnus (typically without striations) formed due to a distal carina of tooth contacting narrow edge of bone (see D'Amore and Blumenschine, Reference D'Amore and Blumenschine2012). (2) Possible configurations are shown of tooth–bone contacts involving mesial, distal cutting edges (carinae) and tooth tips (teeth in all cases shown in side views). Feasibility of distal cutting-edge contact is lower for curved tooth. Low- and high-curvature teeth and two bone elements differing in cross-sectional areas are considered. Note that adjacent teeth are ignored here, but dental spacing would allow only bones of certain maximal sizes to slip between the teeth. This could limit the mesial and distal cutting-edge contacts to those bone elements that fit in between. (3) Linichnus serratus (b): formation and morphology (“In” and “Out” refer to the tooth movement in respect to the bone). Top and side views are illustrated for the tooth–bone interaction. The trace is thought to form due to tooth edge–bone contact. (4) Tooth kinetics and morphology of Nihilichnus with a zone of fractured bone around. Dashed arrows in all depict the general tooth kinetics, and block diagrams show simplified morphologies of resulting traces and their sectional views. Circles with dots are movement vectors (arrow heads are directed toward the page), perpendicular (in (1) and (2)) and parallel (in (3)) to the cutting edges of the teeth (or tooth symmetry plain). Abbreviations used in the figures: Lb = Linichnus bromleyi; Ls(a) = Linichnus serratus (a); Ls(b) = Linichnus serratus (b); N = Nihilichnus.
The hook scores and other scores made by extant crocodylians display commonly irregular serration along their edges (see Njau and Blumenschine, Reference Njau and Blumenschine2006, fig. 6A; Drumheller and Brochu, Reference Drumheller and Brochu2014, fig. 3). Those crocodylian traces resemble the Linichnus holotype in this respect (see Jacobsen and Bromley, Reference Jacobsen and Bromley2009) and the traces from the Grabowa Formation illustrated in Figure 4.6. Serrations along fossil Linichnus edges are interpreted as traces of denticles (see, e.g., Jacobsen and Bromley, Reference Jacobsen and Bromley2009). Extant crocodylians, however, do not have denticulated teeth (see Erickson et al., Reference Erickson, Lappin and Vliet2003; Njau and Blumenschine, Reference Njau and Blumenschine2006) but still produce serrated scores and furrows. Therefore, the origin of serrated edges of those traces is likely unrelated to the tooth-edge morphology, in contrast to bisected pits, punctures, or striations in scores and furrows; their morphology may owe its origin to carinae or denticles of ziphodont teeth (see, e.g., Njau and Blumenschine, Reference Njau and Blumenschine2006; D'Amore and Blumenschine, Reference D'Amore and Blumenschine2009, Reference D'Amore and Blumenschine2012; Vasconcellos and Carvalho, Reference Vasconcellos and Carvalho2010; Njau and Gilbert, Reference Njau and Gilbert2016). In addition, there is no Knethichnus-like component associated with the crocodylian-made traces of Linichnus-type morphology or the Linichnus holotype itself (see Jacobsen and Bromley, Reference Jacobsen and Bromley2009; compare D'Amore and Blumenschine, Reference D'Amore and Blumenschine2012). For this reason, tooth denticulated edges most likely did not participate in the production of any of those bone modifications, and the tooth tip–bone contact is the likely scenario (compare Njau and Blumenschine, Reference Njau and Blumenschine2006; D'Amore and Blumenschine, Reference D'Amore and Blumenschine2009, Reference D'Amore and Blumenschine2012; Jacobsen and Bromley, Reference Jacobsen and Bromley2009; Drumheller and Brochu, Reference Drumheller and Brochu2014; Njau and Gilbert, Reference Njau and Gilbert2016).
The ”tooth tip contact” interpretation could be applied to winding Linichnus bromleyi from Figure 3.2 and Linichnus serratus (a) from Figures 3.1 and 4.6. If those bite traces indeed originated due to tooth tip–bone contacts, how then can the serration along edges in Linichnus serratus (a) traces be explained?
Associations of pits (snags) and scores made by crocodylians (see Njau and Blumenschine, Reference Njau and Blumenschine2006; Drumheller and Brochu, Reference Drumheller and Brochu2014; Njau and Gilbert, Reference Njau and Gilbert2016) may offer an explanation applicable to tooth tip-made curved, winding traces and/or those having U-shaped cross sections (see Fig. 4.6). Those crocodylian pits (Nihilichnus-like traces) are superimposed over scores (Linichnus-like traces) and may form a serration-like pattern along the trace edges if their spacing is dense enough. The pattern occurs on both sides of the main groove and is comparable to serration of Linichnus holotype (Jacobsen and Bromley, Reference Jacobsen and Bromley2009). Some of those superimposed pits show bisected morphologies indicating that indeed it was the tooth tip that punctured and gouged the bone (compare Njau and Blumenschine, Reference Njau and Blumenschine2006; Njau and Gilbert, Reference Njau and Gilbert2016).
It is proposed here, from comparison with crocodylian bites, that the serration along the edges of Linichnus serratus (a) from the Grabowa Formation (Figs. 3.1, 4.6) might have resulted from interrupted movement of the tooth along its path during a tooth tip–bone interaction (Fig. 6.1). The shallow repetitive pits making the serrated edges would represent short pauses in the tooth movement during which the vertical force(s) would temporarily prevail over those trying to pull the tooth along the bone surface (see Figs. 4.6, 6.1). According to the proposed interpretation, the smooth edges of L. bromleyi (Fig. 4.2) may suggest that the vertical force component could be negligible (no impact on trace morphology) and that dragging forces dominated the tooth when gouging the bone. In conclusion, crocodylian-made associations of scores and pits (see Binford, Reference Binford1981; Njau and Gilbert, Reference Njau and Gilbert2016) support the view that L. serratus (a) from the Grabowa Formation may result from a trade between bone-vertical and bone-parallel tooth kinetics.
Tooth edge–bone contact
Tooth edge cutting the bone typically forms a straight groove (following the shape of the edge) with or without serration along its edges (see Fig. 2.1; D'Amore and Blumenschine, Reference D'Amore and Blumenschine2009, Reference D'Amore and Blumenschine2012; Bianucci et al., Reference Bianucci, Sorce, Storai and Landini2010; Muñiz et al., Reference Muñiz, Belaústegui, Toscano, Ramirez-Cruzado and Vintaned2020). Some of the short and straight Linichnus traces (L. serratus (b); see Table 1 and Fig. 5.1, 5.16–5.21) show distinct V-shaped cross sections and fine, trace-axis-perpendicular striation along one of their edges (see Fig. 5.16–5.21; compare Jacobsen and Bromley, Reference Jacobsen and Bromley2009, fig. 2A). These are likely to have formed through cutting-edge contact with bone. Ziphodont tooth may contact bone with mesial or distal cutting edge (carina). D'Amore and Blumenschine (Reference D'Amore and Blumenschine2009, Reference D'Amore and Blumenschine2012) illustrated short bite traces of Komodo monitors (edge marks) they thought almost certainly formed when the distal carina of the last tooth contacted the edge of bone (e.g., rib) or when a thin bone element slipped between adjacent teeth.
The teeth of the Komodo monitor get shorter toward the back of its jaws. Those at the very back are short and strongly curved backward. Their distal cutting edges have almost no straight parts, making the contact with bone difficult (see D'Amore and Blumenschine, Reference D'Amore and Blumenschine2009, Reference D'Amore and Blumenschine2012). The lodging of those rear teeth against the bone appears an unlikely scenario or at least a rare event. However, formation of clusters of parallel edge marks (see D'Amore and Blumenschine, Reference D'Amore and Blumenschine2009, fig. 7A) would require repetitive tooth–bone contacts. Longer teeth that Komodo monitors have in the middle of their snouts seem to be better suited to produce such clusters in repetitive bites ; their distal cutting edges have straight parts to modify the bone.
Figure 6.2 shows how tooth curvature and size of bone (in relation to the tooth's size and its curvature) may affect the feasibility of mesial and distal carina contacts. The low-curvature teeth are likely to easily engage in tooth-tip contacts (the bone does not need to fit between adjacent teeth to make this contact). Mesial- and distal-edge contacts may easily take place for low-curvature teeth as long as the bone fragment is small enough to slip between the adjacent teeth. The relatively large size of bone when compared with the tooth may hinder distal cutting-edge contacts in high-curvature teeth where, due to a backward movement of the head, tooth tip may contact bone surface first and prevent subsequent proper contact with the tooth cutting edge. The tooth-tip contacts are thought to be hindered for high-curvature teeth. Their tips are directed backward, not ventrally. The high-curvature teeth may easily contact bone with their mesial cutting edges, most likely with the backwardly curved part of their mesial carinae. The curvature brings that part of the mesial cutting edge to a more ventral position. Ziphodonts, strongly curved teeth of Komodo monitors, may be good examples of this; the teeth are heavily worn down in respective parts of their mesial carinae (see D'Amore and Blumenschine, Reference D'Amore and Blumenschine2012). In general, distal carina contacts require bone to fit between adjacent teeth or to contact the distal carina of the last tooth in the row (see D'Amore and Blumenschine, Reference D'Amore and Blumenschine2009, Reference D'Amore and Blumenschine2012). Contact between bone and distal tooth carina is thought to become increasingly less feasible than that with mesial carina with increasing tooth backward curvature (Fig. 6.2).
Entrapment of a bone element between adjacent teeth should result in a set of bite traces on both sides of that element: one resulting from bone contact with distal and one with mesial carina of adjacent teeth. However, no such set of L. serratus (b) is distinguishable in the studied material (see Fig. 5).
The straight shape of the grooves ascribed to L. serratus (b) and their striation pattern (including its presence along one edge of the trace) could be explained by the tooth-edge contact scenario (see Fig. 5.16–5.21). Results of the performed cutting tests (Fig. 2) may help to match the bite morphology with the tooth kinetics.
Cuts illustrated in Figure 2.9, 2.10, 2.12, 2.13 show morphologies comparable to L. serratus (b); the cuts display striation along one of their edges emanating from the axis toward the edge of each cut (trace-axis perpendicular). Morphologies of cuts from Figure 2.12, 2.13, due to their distinct V-shaped cross sections, are especially similar to the studied L. serratus (b). Kinetics similar to those from Figure 2.8, 2.11 are proposed herein as models for tooth kinetics that produced L. serratus (b) from the Grabowa Formation (see Fig. 5.1, 5.16–5.21).
D'Amore and Blumenschine (Reference D'Amore and Blumenschine2012, fig. 3D) illustrated a cast of Komodo monitor-made edge mark with striations. The pattern, as in L. serratus (b), resembled grooves of Knethichnus. Therefore, formation of the edge marks likely required not only that the tooth edge contact the bone (see D'Amore and Blumenschine, Reference D'Amore and Blumenschine2009, see fig. 10C therein) but also that the tooth kinetic include a movement component at an angle to the denticulated tooth edge (see Fig. 6.1; compare D'Amore and Blumenschine, Reference D'Amore and Blumenschine2012). The denticles on the distal cutting edge of a tooth would most likely produce Knethichnus-like striations in an edge mark during the initial phase of tooth–bone contact, when the tooth exerted force on the bone due to the head being pulled backward. This kinetics seems to be different from the one proposed here for L. serratus (b). Most of the edge marks made by Komodo monitors show no striations (see D'Amore and Blumenschine, Reference D'Amore and Blumenschine2009). Thus, detailed interpretation and comparison is hindered for the tooth–bone interactions and the tooth kinetics.
Potential tracemakers
Morphologically fairly uniform conical teeth, like those filling jaws of extant and extinct carnivorous reptiles (including reptilian ancestors of mammals; see, e.g., Benton, Reference Benton2005), might contact bones and modify their surfaces through a tooth tip–bone or a tooth edge–bone contact, leaving various traces (see, e.g., D'Amore and Blumenschine, Reference D'Amore and Blumenschine2009, Reference D'Amore and Blumenschine2012; Njau and Gilbert, Reference Njau and Gilbert2016). In contrast to bites made by mammalian carnivores, those of reptiles are typically morphologically plain, representing pits, punctures, scores, and furrows not unique enough to allow easy identification of the tracemakers (see Murmann et al., Reference Murmann, Brumit, Schrader and Senn2006; Smits and Evans, Reference Smits and Evans2012; Evans and Pineda-Munoz, Reference Evans, Pineda-Munoz, Croft, Su and Simpson2018).
However, a study by Aramendi et al. (Reference Aramendi, Maté-González, Yravedra, Ortega, Arriaza, González-Aguilera, Baquedano and Domínguez-Rodrigo2017) shows that even pits made by various reptilian and mammalian carnivores may be distinguished with dedicated three-dimensional modeling and visualization techniques (see also Pante et al., Reference Pante, Muttart, Keevil, Blumenschine, Njau and Merritt2017; Souron et al., Reference Souron2019). Bite traces made by recent large carnivorous reptiles on bones match the general morphologies of traces studied in the current fossil sample comprising Linichnus and Nihilichnus ichnogenera from the Grabowa Formation (compare Njau and Blumenschine, Reference Njau and Blumenschine2006; D'Amore and Blumenschine, Reference D'Amore and Blumenschine2009; Westaway et al., Reference Westaway, Thompson, Wood and Njau2011; Baquedano et al., Reference Baquedano, Domínguez-Rodrigo and Musiba2012; Drumheller and Brochu, Reference Drumheller and Brochu2014; Njau and Gilbert, Reference Njau and Gilbert2016).
The Grabowa Formation has provided bone fragments of various reptiles, including carnivorous archosaurs and herbivores—dicynodontids (see, e.g., Niedźwiedzki et al., Reference Niedźwiedzki, Sulej and Dzik2011; Sulej et al., Reference Sulej, Bronowicz, Tałanda and Niedzwiedzki2011; Niedźwiedzki and Budziszewska-Karwowska, Reference Niedźwiedzki and Budziszewska-Karwowska2018; Sulej and Niedźwiedzki, Reference Sulej and Niedźwiedzki2018). The skeletal remains recovered from the formation included teeth of semiaquatic and fully terrestrial carnivorous archosaurs, such as phytosaurs and plausible large theropods, respectively (see Niedźwiedzki et al., Reference Niedźwiedzki, Gorzelak and Sulej2010; Sulej et al., Reference Sulej, Bronowicz, Tałanda and Niedzwiedzki2011; Niedźwiedzki and Budziszewska-Karwowska, Reference Niedźwiedzki and Budziszewska-Karwowska2018). Fossilized tracks from the Grabowa Formation render a fauna composition image matching the one emerging from the bone findings, which indicates the presence of archosaurs and dicynodontids (see Sadlok and Wawrzyniak, Reference Sadlok and Wawrzyniak2013). Overall, the makers of studied bite traces are likely to be found among carnivorous archosaurs whose fossil remains and footprints have been recovered from the deposits of the unit (including plausible theropods; see also Niedźwiedzki et al., Reference Niedźwiedzki, Gorzelak and Sulej2010).
Carnivorous feeding strategies and bite traces
A predator kills its prey to feed. Therefore, it may put selective pressure on the population of its prey species by removing some genes from the pool (see, e.g., Berryman, Reference Berryman1992; Lima, Reference Lima1998). Scavengers employ an opportunist strategy by feeding on carcasses found in the environment. A scavenger, in contrast to a predator, does not exert selective pressure on the population of animals it feeds on. This highlights the importance, especially in the paleoecological context, of distinguishing between true predichnia (see Carpenter, Reference Carpenter1998) and postmortem feeding tooth traces, including scavenging (compare Vallon et al., Reference Vallon, Rindsberg and Bromley2016).
Despite the fundamental differences between predatory and nonpredatory carnivorous ethologies, predation and scavenging may be practiced by the same species (see, e.g., Jędrzejewska and Jędrzejewski, Reference Jędrzejewska and Jędrzejewski2001; Ordiz et al., Reference Ordiz, Milleret, Uzal, Zimmermann, Wabakken, Wikenros, Sand, Swenson and Kindberg2020). Not surprisingly, one carnivore may produce predichnia and/or nonpredatory, postmortem feeding bites, depending on the chosen strategy and performed behavior.
Disentanglement between the bio- and ichnotaxa is a well-known phenomenon in the realm of trace fossils (compare Bromley, Reference Bromley1990). Traces of predator(s) are not necessarily traces of predation as a predator may kill and then feed on the pray, leaving bite traces one could associate with predatory and with postmortem feeding behaviors, respectively. The general feeding strategy a carnivore applies may depend on the prey/carcass availability in the environment; increased mortality may favor scavenging, as carcasses could be a predictable food source and are certainly easier to catch than living prey (see, e.g., Henschel and Skinner, Reference Henschel and Skinner1990; Jędrzejewska and Jędrzejewski, Reference Jędrzejewska and Jędrzejewski2001).
Ethology of bite traces from the Grabowa Formation.—Tooth-marked bones from the Grabowa Formation have previously been reported and provided some data on trophic interactions. Work by Budziszewska-Karwowska et al. (Reference Budziszewska-Karwowska, Bujok and Sadlok2010) and the subsequent paper by Niedźwiedzki et al. (Reference Niedźwiedzki, Gorzelak and Sulej2010) both assessed bite traces on bones assignable exclusively to the first-order consumers of this Late Triassic habitat, the so-called dicynodontids, which were unique large-bodied reptiles (see Cox and Parrington, Reference Cox and Parrington1965; Thulborn and Turner, Reference Thulborn and Turner2003; Wawrzyniak, Reference Wawrzyniak and Nowakowski2010).
Niedźwiedzki et al. (Reference Niedźwiedzki, Gorzelak and Sulej2010) postulated that the bite marks they studied represented predichnia left by large theropods on dicynodontid bones (see also Sulej and Niedźwiedzki, Reference Sulej and Niedźwiedzki2018). However, supporting evidence of predation was missing. Moreover, the circular outline of some bites (Nihilichnus; see Niedźwiedzki et al., Reference Niedźwiedzki, Gorzelak and Sulej2010, fig. 1H) was not typical of the trace made by narrow teeth of theropods (see Avilla et al., Reference Avilla, Fernandes and Ramos2004; Smith et al., Reference Smith, Vann and Dodson2005), including teeth from the same formation (see Niedźwiedzki and Budziszewska-Karwowska, Reference Niedźwiedzki and Budziszewska-Karwowska2018).
Budziszewska-Karwowska et al. (Reference Budziszewska-Karwowska, Bujok and Sadlok2010) made a detailed size analysis of various bite traces and their spacing on a single dicynodontid limb to infer a minimal number of tracemakers involved. They analyzed the possible impact of jaw–bone kinetics on the inferred dental spacing and concluded that the specimen could have been scavenged as bite traces suggested that more than one size class of carnivores likely fed on the reptile corpse.
Hone and Chure (Reference Hone and Chure2018) conducted a similar analysis of spacing between tooth traces and jaw–bone kinetics in their study whereas D'Amore and Blumenschine (Reference D'Amore and Blumenschine2012) applied similar geometrical analysis in their actualistic studies of tooth–bone kinetics and its impact on resulting spacing of striations in bite traces (see also Buffetaut, Reference Buffetaut1983; Erickson, Reference Erickson1984; Cisneros, Reference Cisneros2005; Hone and Watabe, Reference Hone and Watabe2010; Noto et al., Reference Noto, Main and Drumheller2012; Casal at al., Reference Casal, Martínez, Ibiricu, Riga and Foix2013; Botfalvai et al., Reference Botfalvai, Prondvai and Ősi2014; Hone and Chure, Reference Hone and Chure2018). Works of Budziszewska-Karwowska et al. (Reference Budziszewska-Karwowska, Bujok and Sadlok2010) and D'Amore and Blumenschine (Reference D'Amore and Blumenschine2012) show that spacing of tooth traces and spacing of striations in most cases are poor indices of interdental and interdenticle spacing. Both works showed also that spacing of tooth traces and striations may be used as proxies of minimal interdental and minimal interdenticle spacing, respectively.
Sarcophagichnia.—The present report includes two tooth-marked bone fragments assignable to carnivorous archosaurs (see Fig. 3.1, 3.2; Table 1; see also Niedźwiedzki and Budziszewska-Karwowska, Reference Niedźwiedzki and Budziszewska-Karwowska2018). These fragmentary bones are the first direct indication of second- or third-order consumers serving as a food source for other meat eaters in that Late Triassic habitat.
According to Niedźwiedzki and Budziszewska-Karwowska (Reference Niedźwiedzki and Budziszewska-Karwowska2018), the two bone fragments represent elements of archosaurian pelvic girdles. The belly sides of the animals could be accessible to the carnivores most likely after they had died and were rested on their sides. The location of the bites suggests they are probably not predichnia (killing bite traces) and that the traces were most likely inflicted postmortem due to feeding behavior rather than inter- or intra-specific fights (compare Webb et al., Reference Webb, Manolis and Buckworth1983; Jacobsen, Reference Jacobsen1998; Tanke and Currie, Reference Tanke and Currie1998; Avilla et al., Reference Avilla, Fernandes and Ramos2004; Katsura, Reference Katsura2004; Longrich et al., Reference Longrich, Horner, Erickson and Currie2010; Zammit and Kear, Reference Zammit and Kear2011; Drumheller et al., Reference Drumheller, McHugh, Kane, Riedel and D'Amore2020).
The upper parts of hindlimbs and tail bases of archosaurs’ bodies would be associated with considerable mass of edible muscle tissues (see, e.g., Carrano and Hutchinson, Reference Carrano and Hutchinson2002; Schachner et al., Reference Schachner, Manning and Dodson2011). Easily accessible body parts of high muscle content would represent high nutrition value to carnivores (see, e.g., Blumenschine, Reference Blumenschine1986) and would be likely targeted early during the carcass consumption (see Longrich et al., Reference Longrich, Horner, Erickson and Currie2010; Drumheller et al., Reference Drumheller, McHugh, Kane, Riedel and D'Amore2020). However, muscles originating on distal pubis and ischium could be less attractive due to hindered access (hidden under shallower upper hindlimb musculature; see, e.g., pelvis and hindlimb myological reconstruction of archosaur in Schachner et al., Reference Schachner, Manning and Dodson2011).
Data provided by Drumheller et al. (Reference Drumheller, McHugh, Kane, Riedel and D'Amore2020, table 4) showed that pectoral and pelvic girdle elements of various tetrapods (including theropods) provided consistently fewer tooth traces than easily accessible ribs of the same tetrapod group—the other high-economy element. A size comparison of muscle origin points on distal pubis and ischium in crocodiles, theropods, and birds (see, e.g., Carrano and Hutchinson, Reference Carrano and Hutchinson2002) may also suggest that muscle nutrition value could be low for these distal elements.
The other two bone fragments (Figs. 4, 5) display bite traces of variable sizes, occurring on all the available (preserved) surfaces. Some of the traces (see Fig. 5.4–5.7, 5.11–5.14) appear to form sets of parallel traces (see dashed lines in Fig. 5). Thinner traces (e.g., Fig. 5.5) also display denser packing (smaller trace spacing) than do thicker traces (e.g., Fig. 5.14). It seems reasonable to assume that at least some of those surfaces would be inaccessible for carnivores during lifetimes of the bone-source animals. Those fragments would be still embedded within soft tissues and/or articulated with other bones. Seemingly unrestricted access may suggest that at least some of the bite traces represent wounds inflicted postmortem (compare Forrest, Reference Forrest2003), perhaps even after the bone-containing part of the carcass had been detached from the rest of a corpse, e.g., because of ongoing dismembering that could be due to activity of predator(s) that made the kill and/or scavengers (see comments on carcass reduction in Jacobsen, Reference Jacobsen1998).
The bite traces on the bone fragments studied herein (Figs. 3–5) most likely represent a record of feeding by carnivorous archosaurs. A new ethological category is proposed herein for these traces to not classify them as predichnia. The category is sarcophagichnia (traces of feeding on a body). The category includes predichnia as a subcategory and is supposed to provide a more neutral way of classifying tooth traces on bones than the predichnia used to (no distinction between predatory and nonpredatory tooth traces is needed at this point).
Predichnia
It is extremely challenging to distinguish between true predatory traces (predichnia) with no signs of healing and postmortem bites (see also Vallon et al., Reference Vallon, Rindsberg and Bromley2016). However, it is worth the effort even though classifying the bite traces can be problematic (see Avilla et al., Reference Avilla, Fernandes and Ramos2004).
Positive identification of a fatal bite is not trivial, even when it concerns forensic analysis of recent cases (see Chattopadhyay et al., Reference Chattopadhyay, Shee and Sukul2013), let alone the fossil material (see Hone and Chure, Reference Hone and Chure2018). The bite location may aid in predichnia identification. Predators may target the throat, neck, head (e.g., felids), abdomen, or tail fin (e.g., sharks) to neutralize the prey, overcome its defensive adaptations, and kill (see Schaller and Vasconcelos, Reference Schaller and Vasconcelos1978; Steklis and King, Reference Steklis and King1978; Seidensticker and McDougal, Reference Seidensticker and McDougal1993; Clevenger et al., Reference Clevenger, Campos and Hartasanchez1994; Antón and Galobart, Reference Antón and Galobart1999; Martin et al., Reference Martin, Hammerschlag, Collier and Fallows2005; Bianucci et al., Reference Bianucci, Sorce, Storai and Landini2010; Milàn et al., Reference Milàn, Kofoed and Bromley2010; Antón et al., Reference Antón, Siliceo, Pastor, Morales and Salesa2019). None of the studied bite-trace-bearing bone fragments represent the head or neck area, a plausible target for terrestrial predators using killing bites. Therefore, the location requirements are not met for predichnia.
Another way of identifying predichnia would be to look for signs of bone tissue recovery. Healing is evidence that the bite was not lethal and was inflicted antemortem. Such bone modifications would represent good candidates for true predatory ichnites (see, e.g., Erickson, Reference Erickson1984; Carpenter, Reference Carpenter1998; Lebedev et al., Reference Lebedev, Mark-Kurik, Karatajūtė-Talimaa, Lukševičs and Ivanov2009; DePalma et al., Reference DePalma, Burnham, Martin, Rothschild and Larson2013; Drumheller et al., Reference Drumheller, Stocker and Nesbitt2014). However, the present material shows no sign of bone tissue regrowth. Therefore, the supportive evidence for predatory interpretation (predichnia) is missing here.
Necrophagichnia
The consumption of a small animal does not present any difficulties for carnivorous reptiles, especially if the prey constitutes a food item small enough to be swallowed in one piece. However, larger corpses may require some preswallowing processing, including reduction and defleshing. Those take place postmortem due to activity of the predator(s) and/or scavenger(s) (Njau and Blumenschine, Reference Njau and Blumenschine2006; D'Amore and Blumenschine, Reference D'Amore and Blumenschine2009; Westaway et al., Reference Westaway, Thompson, Wood and Njau2011). The fossilized tooth traces that originated on bones during those activities are a record of postmortem feeding behaviors. The proposed ethological category for such trace fossils is necrophagichnia (traces of feeding on an already dead body). The relations between the newly proposed ethological categories/subcategories and predichnia are as follows: sarcophagichnia = predichnia (made by predators only) + necrophagichnia (made by predators and scavengers). The studied bite traces on bones from the Grabowa Formation are found on elements that could represent low nutrition value to carnivores (distal pubis and ischium; compare, e.g., Longrich et al., Reference Longrich, Horner, Erickson and Currie2010; Drumheller et al., Reference Drumheller, McHugh, Kane, Riedel and D'Amore2020) and on all preserved surfaces, likely also including those hardly accessible during the animal life (compare, e.g., Forrest, Reference Forrest2003; Longrich et al., Reference Longrich, Horner, Erickson and Currie2010). Therefore, the bite traces are interpreted as postmortem feeding and for this reason are classified as necrophagichnia.
Food-processing behavioral patterns and bite traces
Behavior is a complex and hierarchical phenomenon, and trace fossil morphology may, in some cases, reflect aspects of that hierarchy (see Fürsich, Reference Fürsich1974; Miller, Reference Miller2003). Bite trace fossils are not different. Such ichnites result from tooth and bone interaction, and they may convey a good deal of information on food-processing behavioral patterns that manifested during trace production. Those patterns (e.g., related to teeth and jaw kinetics) may be deciphered from the fossilized material (see, e.g., Fig. 7.1–7.3) with the knowledge of food-processing behavior of recent carnivores and associated tooth traces. Reptiles, such as crocodylians and large varanids, process large food items before swallowing and their teeth leave traces on bones during dismembering and defleshing (see, e.g., Njau and Blumenschine, Reference Njau and Blumenschine2006; D'Amore and Blumenschine, Reference D'Amore and Blumenschine2009; Westaway et al., Reference Westaway, Thompson, Wood and Njau2011).

Figure 7. Food-processing behavioral pattern and resulting trace fossil. (1) Linichnus serratus (a) and L. bromleyi as results of dismembering (reduction) and defleshing, respectively; cartoon bone = trace-bearing specimen; cartoon skull = carnivorous archosaur. (2) Linichnus serratus (b) as a result of defleshing of a corpse (cartoon bone) by a carnivorous archosaur (cartoon skull). (3) Nihilichnus as a result of dismembering (reduction behavior) of a corpse (cartoon bone) by a carnivorous archosaur (cartoon skull). The fractured zones visible around some Nihilichnus traces suggest a large force applied to close the jaw (solid and firm grip on the bone). Arrows show generalized head movement (and bone movement in (3)). Note that in (3), the skull and the bone move as a unit (no bone gouging; compare with (1)).
Extant large carnivore archosaurs swallow food items with bones via the so-called inertial feeding mechanism. They execute repetitive cycles of jaw closing–opening and head rising–lowering to pass the food item down the esophagus (see Cleuren and De Vree, Reference Cleuren and De Vree1992, Reference Cleuren, De Vree and Schwenk2000). It is likely that some bite traces form as the food item is repositioned in jaws during inertial feeding. It would be difficult to discern morphologically between such bite traces and those made during dismembering or defleshing. However, it is very likely that bite traces of archosaurs dismembering or defleshing the corpse and those originating during the inertial feeding would have different preservation potential in natural settings and hence representation in fossil material. This is because, contrary to mammalian carnivores, large extant archosaurs have very aggressive stomach acids and food passes slower through their digestive tracts (see Fisher, Reference Fisher1981). They tend to completely digest bones they managed to swallow before they pass stomach content further down into intestines (compare Fisher, Reference Fisher1981; Schmitt and Juell, Reference Schmitt and Juell1994; Milàn, Reference Milàn2012). As a result, no bone fragments are to be found in extant crocodylian feces (see Milàn, Reference Milàn2012), and any potential bite traces on swallowed and digested bones are lost, including those made during inertial feeding. No edge rounding or polishing of bone fragments is observed in studied examples to provide evidence for swallowing and subsequent partial digestion (compare Schmitt and Juell, Reference Schmitt and Juell1994; Chin et al., Reference Chin, Tokaryk, Erickson and Calk1998). Moreover, some of the studied thin and shallow surficial bite traces (Fig. 4.2) would likely be easily destroyed by the action of digestive acids.
Still, bite traces resulting from inertial feeding could potentially be preserved on abandoned bone elements (if somehow they avoided swallowing). Positive identification of such traces would pose a major challenge in fossil material. As the purpose of inertial feeding is to swallow the food, not abandon it, such instances should be rare. Therefore, a parsimonious approach is taken here, and studied tooth-marked bone fragments are thought to have been abandoned during dismembering and defleshing rather than resulting from inertial feeding.
Nihilichnus from the Grabowa Formation as a trace of dismembering
Data on feeding behaviors of recent large meat-eating reptiles, such as crocodylian species and Komodo monitors (see Njau and Blumenschine, Reference Njau and Blumenschine2006; D'Amore and Blumenschine, Reference D'Amore and Blumenschine2009; Westaway et al., Reference Westaway, Thompson, Wood and Njau2011; Baquedano et al., Reference Baquedano, Domínguez-Rodrigo and Musiba2012; Drumheller and Brochu, Reference Drumheller and Brochu2014; Njau and Gilbert, Reference Njau and Gilbert2016) suggest that the studied Nihilichnus (Figs. 3.6, 4.1–4.3) was likely the result of tooth-tip pushing with a vertical force on the bone surface (see also Mikuláš et al., Reference Mikuláš, Kadlecová, Fejfar and Dvořák2006). Such traces, especially those with fractured bone margins (Figs. 3.6, 4.3), may indicate that a significant force was applied to close the jaws (compare Mikuláš et al., Reference Mikuláš, Kadlecová, Fejfar and Dvořák2006).
A forceful bite on the bone could lead to a strong and firm grip on the prey/carcass body; the teeth would be well immobilized (locked) inside the pits they had formed in bone surface, preventing them from slipping off and gouging the bone surface. This way, relative tooth–bone movements are inhibited; teeth (jaws) and the bone move as a single unit (Fig. 7.3). This is a condition mandatory for a successful reduction, a behavior performed to tear the corpse apart and ultimately bring the food item sizes down to manageable pieces, small enough for swallowing and ingestion (see Njau and Blumenschine, Reference Njau and Blumenschine2006).
L. serratus (a) from the Grabowa Formation as a trace of failed dismembering
Linichnus serratus (a) in the present sample (Figs. 3.1, 4.6) also could have formed during reduction behavior. However, contrary to the Nihilichnus case, the tooth tips had to grip the bone with insufficient force (compare, e.g., Njau and Gilbert, Reference Njau and Gilbert2016). In other words, to form Linichnus serratus (a), the teeth (jaws) and bone must not move as a single unit (see Fig. 7.1, 7.2). A grip that is too weak would be necessary to allow the teeth to leave the pits they had formed in the bone (compare Taylor, Reference Taylor1987; Njau and Blumenschine, Reference Njau and Blumenschine2006; Njau and Gilbert, Reference Njau and Gilbert2016). Therefore, fossil Linichnus serratus (a) (e.g., Fig. 4.6) may represent a failed grip during a dismemberment attempt (see also “puncture and pull” traces in Erickson and Olson, Reference Erickson and Olson1996).
In crocodylians, jaw-closing force could be exceeded by torsional forces appearing during death rolls or due to forces resulting from side-to-side head shakes; both are examples of behavioral patterns associated with reduction. The resulting bite traces on bones resemble Linichnus trace fossils, including some (Figs. 3.1, 4.6) from the Grabowa Formation (see Taylor, Reference Taylor1987; Njau and Blumenschine, Reference Njau and Blumenschine2006; Baquedano et al., Reference Baquedano, Domínguez-Rodrigo and Musiba2012; Njau and Gilbert, Reference Njau and Gilbert2016).
Unlike terrestrial carnivores, crocodiles typically reduce their food items in water where they must deal with corpse buoyancy (see Taylor, Reference Taylor1987). They may use forceful side-to-side head shakes or death rolls to reduce a buoyant corpse. The latter behavior is sometimes executed with a kind of cooperation between carnivores; two animals may catch the large corpse on its two ends and execute their death rolls in opposite directions (see Taylor, Reference Taylor1987; Njau and Blumenschine, Reference Njau and Blumenschine2006). Reduction of a corpse resting on land is an easier task than reducing one in water as the land-dwelling carnivores may utilize their limbs and the inertial mass of a corpse itself to stabilize it during reduction and counteract their own pulling forces.
Varanus komodoensis Ouwens, Reference Ouwens1912 is an example of a large carnivorous reptile that feeds and processes the corpse on land (see Moreno et al., Reference Moreno, Wroe, Clausen, McHenry, D'Amore, Rayfield and Cunningham2008; D'Amore and Blumenschine, Reference D'Amore and Blumenschine2009). This carnivore uses its broad snout and sharp, narrow teeth to execute a specific type of medial–caudal strikes on the carcass it feeds upon (see D'Amore and Blumenschine, Reference D'Amore and Blumenschine2009). The reptile repeats this behavior and removes chunks of flesh using mainly the corpse's weight to counteract pulling forces generated by the muscle apparatus of its own body (see Moreno et al., Reference Moreno, Wroe, Clausen, McHenry, D'Amore, Rayfield and Cunningham2008; D'Amore and Blumenschine, Reference D'Amore and Blumenschine2009; D'Amore et al., Reference D'Amore, Moreno, McHenry and Wroe2011). Most of the resulting bite traces resemble Linichnus and Knethichnus, but only a few are like Nihilichnus (see D'Amore and Blumenschine, Reference D'Amore and Blumenschine2009, Reference D'Amore and Blumenschine2012). This may indicate a true behavioral pattern (avoiding strong biting), but it may also be the result of experimental settings in which Komodo monitors were fed parts of goat prepared (already dismembered to some degree) by researchers (see D'Amore and Blumenschine, Reference D'Amore and Blumenschine2009). It is also likely that if multiple individuals were feeding on one goat at the same time (compare D'Amore and Blumenschine, Reference D'Amore and Blumenschine2009), then dismembering could result from competition between them, and perhaps more bite traces of this sort would be observed as a result.
L. serratus (b) from the Grabowa Formation as a trace of defleshing
The analysis of V. komodoensis bite traces by D'Amore and Blumenschine (Reference D'Amore and Blumenschine2009, Reference D'Amore and Blumenschine2012) clearly showed that some Linichnus-type traces on fossil bones may result from accidental tooth–bone interactions during defleshing (compare also theropod behavior in Fiorillo, Reference Fiorillo1991; Jacobsen, Reference Jacobsen1998). The L. serratus (b) traces (Fig. 5.1, 5.16–5.20) with their V-shaped cross sections resemble (in terms of their general morphology) the so-called edge marks made by V. komodoensis (D'Amore and Blumenschine, Reference D'Amore and Blumenschine2009). The data available (see D'Amore and Blumenschine, Reference D'Amore and Blumenschine2009, Reference D'Amore and Blumenschine2012) on the internal morphology and striation pattern of those edge marks is sufficient at this stage to allow a gross comparison with the material from the Grabowa Formation. Although detailed interpretation of tooth kinetics for the edge marks is hindered as most of them have no striations, the rare instances where striations are preserved show that some lateral movements were taking place during defleshing effort (see D'Amore and Blumenschine, Reference D'Amore and Blumenschine2009, Reference D'Amore and Blumenschine2012). Therefore, this actualistic study showed that bite morphologies combining Linichnus (a groove in bone) with Knethichnus (striations on bone) may result from defleshing of bone with ziphodont teeth (D'Amore and Blumenschine, Reference D'Amore and Blumenschine2009, Reference D'Amore and Blumenschine2012). Similar interpretation may also apply to some bite traces from the Grabowa Formation, with L. serratus (b) being a good candidate morphology.
The results of cutting tests (Fig. 2) suggest the tooth making L. serratus (b) had to (Fig. 2.8, 2.11): (1) contact and penetrate the surface of bone with its denticulated edge and (2) leave the bone substrate with a sideways movement (compare Bianucci et al., Reference Bianucci, Sorce, Storai and Landini2010, fig. 1). This particular tooth kinetics may explain striation on one edge of L. serratus (b) from the Grabowa Formation and suggests that it may represent a side pull of jaws/head, likely taking place during defleshing behavior (compare Njau and Blumenschine, Reference Njau and Blumenschine2006; D'Amore and Blumenschine, Reference D'Amore and Blumenschine2012), when the carnivore was targeting soft tissues rather than the bone itself; the tooth–bone contact could be accidental (Fig. 7.2; compare Erickson and Olson, Reference Erickson and Olson1996).
L. bromleyi from the Grabowa Formation as a trace of defleshing
The lack of serrated edges and the curved to winding shape of these traces (Fig. 4.2) may suggest negligible vertical forces operated during trace formation, whereas the main force components dragged the tooth along the bone. This suggests that soft tissues could be the target (defleshing). These L. bromleyi traces are closely associated with Nihilichnus (Fig. 4.1–4.3). This close association may suggest that the L. bromleyi are shallow traces due to negligible vertical force gouging bone rather than suboptimal bone density (compare Bell and Currie, Reference Bell and Currie2010; Drumheller and Brochu, Reference Drumheller and Brochu2014).
Feeding strategies and paleohabitat
Crosscutting of bites (Fig. 5.1, 5.6, 5.11, 5.13) suggests time difference between the bites. It may be due to one individual biting the same spot in a repetitive manner (see D'Amore and Blumenschine, Reference D'Amore and Blumenschine2009) or more than one individual feeding on the corpse at different times (scavenging involved). The first interpretation may apply when crosscutting bites are of comparable widths (see, e.g., Fig. 5.5). The second interpretation may apply when the sizes of crosscutting bite traces (e.g., depths and widths) are different (see, e.g., Fig. 5.6) and therefore do not favor a multiple-bites scenario; size difference is likely not due to a variation in bone density as bites crosscut each other and modify the same substrate (compare Drumheller and Brochu, Reference Drumheller and Brochu2014).
Sets of parallel bite traces (see Fig. 5) could result from a single bite, and therefore they could convey information on spacing between the tracemaker's teeth. However, repetitive biting on the same spot (by one individual) may decrease the trace spacing below the interdental spacing of the tracemaker (see D'Amore and Blumenschine, Reference D'Amore and Blumenschine2009). Differentiation between sets emerging due to one bite and those from multiple bites could be difficult (a subtle angular difference could be used for that). Budziszewska-Karwowska et al. (Reference Budziszewska-Karwowska, Bujok and Sadlok2010) showed that spacing of parallel bite traces is very likely to be smaller than the actual spacing of teeth even if a set originated due to a single bite; the spacing was dependent on jaw-bone kinetics (see also Hone and Chure, Reference Hone and Chure2018).
One might consider both sizes of individual traces and spacing between them to aid interpretation. Thin, densely spaced bites (Fig. 5.5, 5.7) and thicker, less densely spaced traces (Fig. 5.5, 5.13) are very likely products of carnivores having different sizes of jaw apparatuses. If such bites co-occur on a small bone fragment, as in the present case (see Fig. 5), then the variation in sizes of individual bite traces (e.g., depths) is likely not due to variation in bone density (compare Drumheller and Brochu, Reference Drumheller and Brochu2014) but rather reflects different jaw force capabilities of involved carnivores.
The present results supplement previous data on carnivore (scavenger) activity in the Late Triassic paleoecosystem represented by the Grabowa Formation (Budziszewska-Karwowska et al., Reference Budziszewska-Karwowska, Bujok and Sadlok2010). A significant number of various bites on some of the specimens (Figs. 4, 5) and their variable sizes, crosscutting relationships, and spacing (e.g., in sets of parallel bite traces; see Fig. 5) may suggest that more than one size class of carnivores fed on and modified the bone surfaces (see Figs. 4, 5; compare Budziszewska-Karwowska et al., Reference Budziszewska-Karwowska, Bujok and Sadlok2010). This could reflect predators hunting and feeding in a pack (e.g., individuals at different ontogenetic stages). However, there are no data from the Grabowa Formation to support this possibility (no such potential carnivore recorded yet). Multiple bites on bone fragments also may form due to various carnivores utilizing the carcass for some time (scavenging involved). This scenario seems more likely in the present cases.
The Norian seasonal climate of the study area could periodically become harsh (e.g., during occasional droughts) and increase the mortality rates among vertebrate populations (see, e.g., Szulc et al., Reference Szulc, Gradziński, Lewandowska and Heunisch2006, Reference Szulc, Racki and Jewuła2015; Szulc, Reference Szulc2007b; Gruszka and Zieliński, Reference Gruszka and Zieliński2008; McKie and Williams, Reference McKie and Williams2009; Fijałkowska-Mader et al., Reference Fijałkowska-Mader, Heunisch and Szulc2015; Jewuła et al., Reference Jewuła, Matysik, Paszkowski and Szulc2019). Elevated death rates would result in many carcasses being available, making scavenging an easy and profitable way of subsisting for the carnivores (compare Jędrzejewska and Jędrzejewski, Reference Jędrzejewska and Jędrzejewski2001; Drumheller et al., Reference Drumheller, McHugh, Kane, Riedel and D'Amore2020).
Conclusions
This report describes bite traces on bone fragments recovered from the Upper Triassic (Norian) Grabowa Formation from southern Poland (Zawiercie site; Table 1). The bite morphologies represent two ichnogenera: Linichnus and Nihilichnus. These trace fossils have been analyzed and discussed in terms of their morphological details (serration of L. serratus (a) and striation of L. serratus (b)), the potential tracemakers, feeding strategies, and food-processing behavioral patterns).
Serration in some of the presented L. serratus (a) traces (Fig. 4.6) could be unrelated to denticles covering the cutting edges of the teeth making these traces. The serration may have resulted from tooth tip–bone contacts rather than a tooth edge–bone interaction (no involvement of denticulated tooth edge), possibly from interrupted movement of the tooth along its path when gouging the bone with the tip. Associations of pits and scores made by crocodylian carnivore species as illustrated by Njau and Gilbert (Reference Njau and Gilbert2016) may be recent analogs explaining the formation of such bite traces from the Grabowa Formation (see Fig. 4.6). However, L. serratus (b) displays a striation pattern (see Fig. 5.16–15.20) that is compatible with the tooth edge–bone contact scenario (Fig. 2.8–2.13).
The analyzed bite traces record meat-eating behaviors of Late Triassic archosaurs and are classified ethologically as sarcophagichnia (traces of feeding on a body). These bite traces were most likely induced postmortem and would represent feeding trace fossils, here classified as necrophagichnia (traces of feeding on an already dead body). In the proposed scheme, sarcophagichnia comprises both necrophagichnia and predichnia (with respective subcategories; see, e.g., Vallon et al., Reference Vallon, Rindsberg and Bromley2016).
Two fragments of pelvic girdle elements likely represent carnivore archosaurs (Niedźwiedzki and Budziszewska-Karwowska, Reference Niedźwiedzki and Budziszewska-Karwowska2018), and therefore the bite traces on them are likely from carnivores targeting the carcasses of second- or third-order consumers; this is the first such record from the Upper Triassic Grabowa Formation.
In addition, the studied Nihilichnus, unlike Linichnus, is thought to originate from a solid and a firm grip on the bone, sometimes resulting in fracturing of the bone surface around the trace (see Figs. 3.6, 4.3). The grip could be part of a reduction behavior (dismembering), when the food item had to be brought down to sizes manageable for swallowing and ingestion. Studied L. serratus (a) traces may represent insufficient strength of the grip during failed reduction (see, e.g., Figs. 3.1, 4.6). Other interpretations may apply to L. bromleyi from the Grabowa Formation (e.g., Fig. 4.2), where the lack of serration/striations and the curved to winding shape of some grooves may suggest the traces resulted from tooth tip–bone interaction but with a negligible contribution from forces acting vertically on the tooth pressing on bone (Fig. 4.2). The studied L. serratus (b) traces could have resulted from accidental tooth–bone contacts (see, e.g., Figs. 4.2, 5.3) and may represent defleshing behavior (e.g., targeting of soft tissues rather than the bone). Finally, the seasonal Norian climate could have favored scavenging as a feeding strategy. The periodic droughts could have stimulated the animals’ mortality rates and increased the number of carcasses available in the habitat, improving their predictability and usability as a source of nutrients.
Acknowledgments
I thank S.K. Drumheller (University of Tennessee, Tennessee, USA) and D.C. D'Amore (State University of New Jersey, New Jersey, USA) for their comments and suggestions—all significantly improved the final version of this work. I also thank Z. Wawrzyniak (University of Silesia in Katowice, Poland) for taking for me a few photos with a Leica Wild L10 microscope, which I used to prepare Figure 5 of this work. I thank A. Hastings (Science Museum of Minnesota, Minnesota, USA) for kindly providing me with the needed literature. Financial support from the University of Silesia in Katowice (Poland) made this work possible.
Data availability statement
Data available from the Dryad Digital Repository: https://doi.org/10.5061/dryad.3ffbg79kj
Competing interests
The author declares none.