Introduction
The placenta ensures fetal development and maternal adaptations via regulation of nutrient and waste transfer, endocrine function, and immunological response. Placental dysfunction can result in poor fetal outcomes such as fetal growth restriction (FGR), which affects 5%–10% of pregnancies. Reference Gardosi, Madurasinghe, Williams, Malik and Francis1 However, the ramifications of inadequate placental function extend far beyond short-term health outcomes. Epidemiological studies show that reduced placental weight and surface area are associated with low birth weight and an increased risk of hypertension, heart failure, coronary heart disease and sudden cardiac death in adult life. Reference Barker, Osmond and Grant2–Reference Barker, Thornburg, Osmond, Kajantie and Eriksson4 Yet despite the critical importance of the placenta, its structural and functional integrity as well as response to environmental challenges remains under-researched and poorly understood.
One of the key functions of the placenta is to transfer nutrients and waste between the mother and the fetus. Underpinning this exchange function is the architecture of the placental vasculature. The human placenta is haemochorial, with fetal (feto-placental) vasculature surrounded by trophoblast and bathed in maternal (utero-placental) blood. An elaborate feto-placental vascular tree is critical for optimal fetal growth, yet this elaboration is particularly sensitive to environmental stressors. Reference Burton, Fowden and Thornburg5 This is exemplified by the characterisation of placentas from FGR babies: there are significant reductions in vascular volume and structural changes throughout the human feto-placental vascular tree from the chorionic plate to the capillaries. Reference Junaid, Bradley, Lewis, Aplin and Johnstone6–Reference Langheinrich, Vorman and Seidenstucker8
Reduced placental vascular complexity has implications beyond impaired nutrient and waste exchange, and it is emerging that poor placental vascularity alters how blood flows to the developing heart and fetal vascular system. These changes in blood flow (haemodynamics) alter the mechanical forces exerted on endothelial cells, thus altering endothelial cell function and blood vessel remodelling. Reference Fisher, Chien, Barakat and Nerem9–Reference Langille11 Moreover, the developing heart beats directly against the resistance of the placental vascular bed, Reference Rudolph12 and therefore, changes in mechanical signals have potent and enduring effects on cardiac development and structure. Reference Courchaine, Rykiel and Rugonyi13 Recent recharacterisation of mouse mutants known to be lethal in gestation revealed striking, and previously unappreciated, placental defects. Reference Perez-Garcia, Fineberg and Wilson14 Notably, strong correlations between placental defects (many of which involved feto-placental vascular malformation) and heart pathology or abnormal fetal vascular development were observed. Reference Perez-Garcia, Fineberg and Wilson14 Other genetically modified mouse experimental models (which include ablation of genes such as PPARγ, Wnt, Hex and Islet-1) have also demonstrated the necessity of an elaborate feto-placental vasculature for optimal fetal heart development, although none have established the consequences for later cardiovascular health. Reference Maslen15–Reference Barak, Nelson and Ong25 Furthermore, severe placental insufficiency in humans results in increased loading of the right ventricle. Reference Kiserud, Ebbing, Kessler and Rasmussen26 However, how alterations in feto-placental vascular structure associate with adult cardiovascular health remains relatively unexplored.
To comprehensively understand the emerging idea of a placenta-cardiovascular axis, and the implications for adult health, it is important for experimental studies to adequately image feto-placental vasculature. To assist researchers with considering the best options for their research question, this review will present current methodologies and considerations for imaging of feto-placental vasculature ex vivo and in vivo in human and experimental models. The review will also provide examples of studies that have implemented the various imaging approaches and the insight they have provided into placental function and fetal outcomes.
Feto-placental vascular structure and function
Fetal blood travels to the human placenta via the umbilical arteries, which upon reaching the placenta diverge and branch into large vessels known as chorionic plate arteries. Reference Burton, Fowden and Thornburg5 These vessels radiate across the fetal surface of the placenta, before plunging into the placenta where they branch again into multiple, elaborately branched villous trees, which arise from a stem villus in the chorionic plate.5 Several villous trees may occupy a lobe (also known as a cotyledon) which is distinguished by septa on the maternal side of the placenta. The finest branches of the villous trees, known as terminal villi, form grape-like structures. Reference Kaufmann, Bruns, Leiser, Luckhardt and Winterhager27 The walls of these terminal villi are extremely thin, making them ideal for maternal-fetal exchange. Reference Kaufmann, Sen and Schweikhart28,Reference Sen, Kaufmann and Schweikhart29 By term, the terminal villi make up almost 40% of the villous volume of the placenta with the feto-placental capillary network greater than 500 km in length and 12–14 m2 in surface area. Reference Burton and Fowden30 Once the fetal blood has travelled through the capillary networks within villi, fetal blood is drained through a venule at the base of each villus, and these venules will then converge into the umbilical vein which returns blood to the fetal circulation. Reference Burton and Fowden30,Reference Wang and Zhao31
Given the inherent ethical and logistical issues of obtaining and imaging human placentas, it is imperative that experimental models are utilised to increase our understanding of placental development and function and consequences for later health outcomes. No commonly used animal model is a perfect model for human placentation, and excellent extensive reviews on comparative placentation have been published by others. Reference Wooding and Burton32–Reference Leiser and Kaufmann34 Here, we restrict our review to focus predominantly on imaging of feto-placental vasculature on human, mouse and rat placentas as the imaging performed in other species is currently limited in comparison. Furthermore, with regard to consideration of the role of feto-placental vasculature in driving fetal cardiovascular development and subsequent health, mice and rats are particularly useful models due to ease of genetic manipulation, short lifespan and the vascular structure of the fetal side of the placenta. Like the human, the mouse and rat placenta is a discoid, haemochorial organ, Reference Vercruysse, Caluwaerts, Luyten and Pijnenborg35,Reference Georgiades, Ferguson-Smith and Burton36 with the rat placenta more invasive when compared to mouse. Reference Vercruysse, Caluwaerts, Luyten and Pijnenborg35 The rodent placenta consists of three distinct regions: (i) the maternal portion (the decidua basalis and underlying myometrium), (ii) junctional zone and (iii) labyrinth zone. The junctional zone (equivalent to the human basal plate) consists of spongiotrophoblast and trophoblast giant cells, which synthesise hormones crucial for fetal development and maternal adaptations to pregnancy. Reference Georgiades, Ferguson-Smith and Burton36–Reference Rossant and Cross38 The labyrinth zone is the site of maternal-fetal exchange and the labyrinth vasculature is argued to be analogous to a single chorionic villus in the human placenta. Reference Georgiades, Ferguson-Smith and Burton36,Reference Rossant and Cross38–Reference Pijnenborg, Robertson, Brosens and Dixon41 In rodents, arterioles from the umbilical artery divide and branch before travelling to the maternal border of the labyrinth zone where they then form a dense capillary bed. Reference Wang and Zhao31,Reference Adamson, Lu and Whiteley40
Imaging feto-placental vasculature
Due to the intricate structure of feto-placental vasculature, it is imperative that imaging approaches capture the correct context for the experimental hypothesis. Biological imaging approaches are diverse and are developing at a rapid rate to provide previously unimaginable perspectives. Analysis of feto-placental vasculature in humans and experimental models has been carried out through a range of two-dimensional (2D) and three-dimensional (3D) imaging techniques both ex vivo and in vivo. These imaging approaches include stereology, electron microscopy, confocal microscopy, micro-computed tomography (micro-CT), light-sheet microscopy, ultrasonography and magnetic resonance imaging (MRI). Each of these approaches provides benefits and limitations for understanding how feto-placental vascular structure informs placental and fetal development and thus, future health outcomes.
Stereology
Stereology enables 3D interpretation of 2D sections of tissue. In essence, random systematic sampling and measurement of tissue cross-sections can provide unbiased and quantitative data of 3D structures such as volume, surface area, length and number. Undoubtedly, stereology has been a prevalent imaging technique to characterise placental structure, particularly in the rodent. Stereology has been used to assess placental morphology in humans, Reference Higgins, Felle, Mooney, Bannigan and McAuliffe42–Reference Jackson, Mayhew and Boyd47 mice, Reference Wyrwoll, Noble and Thomson18,Reference Nuzzo, Camm and Sferruzzi-Perri48–Reference Coan, Ferguson-Smith and Burton61 and rats. Reference Napso, Hung, Davidge, Care and Sferruzzi-Perri62–Reference Hewitt, Mark and Waddell64 Via unbiased randomised sampling of 2D histological images, structures of interest can be quantitated and mathematically extrapolated to infer 3D structure. In the placenta, stereology is commonly used not only to evaluate information of placental vascular length, diameter, surface area, volume and density Reference Wyrwoll, Noble and Thomson18,Reference Higgins, Felle, Mooney, Bannigan and McAuliffe42–Reference Mayhew, Ohadike, Baker, Crocker, Mitchell and Ong44,Reference Wyrwoll, Seckl and Holmes54,Reference Serman, Zunic and Vrsaljko63,Reference Hewitt, Mark and Waddell64 but also to estimate its theoretical diffusion capacity by measuring capillary interhaemal membrane thickness and volumes of placental components, that is, labyrinth zone, spongiotrophoblast and decidual volumes. Reference Coan, Vaughan and Sekita53–Reference Coan, Angiolini, Sandovici, Burton, Constancia and Fowden55,Reference Coan, Ferguson-Smith and Burton59,Reference Coan, Ferguson-Smith and Burton61
Stereology has been implemented to assess placental structure in human pregnancy complicated by FGR, pre-eclampsia and diabetes. Reference Higgins, Felle, Mooney, Bannigan and McAuliffe42–Reference Mayhew, Ohadike, Baker, Crocker, Mitchell and Ong44 FGR placentas exhibited thicker trophoblastic epithelium, a reduction in peripheral villous surface areas and a reduction in volume of peripheral villi and intervillous space. Reference Mayhew, Manwani, Ohadike, Wijesekara and Baker43 In contrast, there were no significant differences in the volume of intervillous space and all types of villi in pre-eclampsia, when compared to normal pregnancy. Reference Mayhew, Manwani, Ohadike, Wijesekara and Baker43,Reference Mayhew, Ohadike, Baker, Crocker, Mitchell and Ong44 These changes support the notion that feto-placental vascular complexity is an important determinant of fetal growth. Higgins and colleagues utilised stereological analysis to examine human placentas from diabetic pregnancies which exhibited increased terminal villous volume, capillary volume and capillary length. Reference Higgins, Felle, Mooney, Bannigan and McAuliffe42 Interestingly, maternal gestational hyperglycaemia affects capillary, but not stromal, development, and these changes may increase specifically the surface areas for maternal-fetal exchange and potentially represent a structural mechanism which accounts for the overgrowth of fetus and abnormally high birth weight. Alternatively, hyperglycaemia elicits excessive insulin secretion which promotes abnormal vascular proliferation, causing downstream abnormalities. Overall, these findings suggest differential condition-specific effects on placental integrity: FGR (but not pre-eclampsia) leads to reduction in structural size and complexity, while gestational diabetes leads to changes in terminal structures.
The early elegant stereological assessments by Coan and colleagues established the developmental dynamics of C57BL/6J mouse placental morphology. Reference Coan, Ferguson-Smith and Burton59,Reference Coan, Ferguson-Smith and Burton61 Maternal blood spaces were shown to expand rapidly between E14.5 and 16.5, whereas fetal capillary development continuously increased in volume and surface area right up to late gestation. Reference Coan, Ferguson-Smith and Burton61 These findings highlight the importance for feto-placental capillary expansion to meet increased fetal demand for nutrients in late gestation when fetal growth is rapid. These initial assessments have been extended to the comparison of different mouse strains, revealing differences in feto-placental structure including growth of feto-placental capillaries and interhaemal membrane thickness, Reference Rennie, Detmar, Whiteley, Jurisicova, Adamson and Sled52 an important consideration when selecting experimental models. In well-established mouse models of developmental origins of health and disease (DOHaD) such as maternal protein restriction Reference Rutland, Latunde-Dada, Thorpe, Plant, Langley-Evans and Leach58 and maternal undernutrition, Reference Coan, Vaughan and Sekita53 stereology has demonstrated changes in placental vascular morphology. Moreover, stereological investigations of knockout-mouse models have given important insights into the dynamic nature of the placental function, especially into the co-ordination of maternal and fetal signals to in part compensate for an adverse environment in utero. Reference Nuzzo, Camm and Sferruzzi-Perri48–Reference Sferruzzi-Perri, Lopez-Tello, Fowden and Constancia50,Reference Coan, Fowden, Constancia, Ferguson-Smith, Burton and Sibley57,Reference Sibley, Coan and Ferguson-Smith60,Reference Napso, Hung, Davidge, Care and Sferruzzi-Perri62 Placental structural development in rats has also been examined by stereology and revealed greater feto-placental complexity in comparison to mice. Reference Serman, Zunic and Vrsaljko63,Reference Hewitt, Mark and Waddell64 In a rat model of glucocorticoid (‘stress’ hormone) excess in pregnancy, the reduction in fetal weight is accompanied by markedly reduced placental weight and feto-placental vascular volume, length and diameter, although not maternal blood spaces. Reference Hewitt, Mark and Waddell64 Similar stereological outcomes have been obtained in other mouse studies of glucocorticoid excess Reference Vaughan, Sferruzzi-Perri, Coan and Fowden51 including 11β-hydroxysteroid dehydrogenase 2 knockout (11β-HSD2-/-) mice which also exhibit reduced cardiac function. Reference Wyrwoll, Noble and Thomson18,Reference Wyrwoll, Seckl and Holmes54 Interestingly, administration of pravastatin to stimulate placental vascular growth factor A and enhance feto-placental angiogenesis in 11β-HSD2-/- fetuses also ameliorated fetal cardiac function, Reference Wyrwoll, Noble and Thomson18 highlighting the likely direct influence of feto-placental vascular structure on fetal organ development. Reference Wyrwoll, Noble and Thomson18,Reference Wyrwoll, Seckl and Holmes54 However, none of the studies mentioned have shown a conclusive role of feto-placental vasculature directly informing adult cardiovascular health.
Stereology is a well-established and low-cost method, albeit laborious, with the advantage of encompassing the entire placental vasculature. Stereology has also yielded important insights into placental vascular morphology in human, mouse and rat alike. However, stereology approaches necessitate dismembering the structural integrity and connectivity of placental vascular networks. Thus, this approach cannot be used to evaluate placental vascular tree topology. To overcome these limitations, high-resolution contrast-enhanced micro-CT (discussed below) may well replace stereology in years to come.
Electron microscopy
Electron microscopy is a 2D imaging technique which includes transmission electron microscopy (TEM) and scanning electron microscopy (SEM). TEM creates high-resolution and high-magnification images of fine structural characteristics by passing electron beams through tissues. However, TEM destroys the connectivity of the placental vascular networks due to the necessity of tissue-sectioning during sample preparation. SEM scans the surface of a tissue specimen and also generates a 2D image of a 3D surface via interaction of high energy electron beam with a prepared specimen. However, SEM destroys surrounding tissues due to the requirements of sample preparation and the high electron beams. In 1955, Wislocki and Dempsey implemented electron microscopy to investigate placental morphology in rats, Reference Wislocki and Dempsey65 pigs Reference Dempsey, Wislocki and Amoroso66 and humans. Reference Wislocki and Dempsey67 Thereafter, electron microscopy has been used by other investigators to delineate fine detail in the placental vasculature of monkeys, Reference Lee and Yeh68 minks, Reference Krebs, Winther, Dantzer and Leiser69,Reference Pfarrer, Winther, Leiser and Dantzer70 horses, Reference Macdonald, Chavatte and Fowden71 rodents Reference Adamson, Lu and Whiteley40,Reference Rennie, Detmar, Whiteley, Jurisicova, Adamson and Sled52,Reference Coan, Ferguson-Smith and Burton59,Reference Detmar, Rennie and Whiteley72–Reference Cahill, Rennie and Hoggarth74 and humans. Reference Lee and Yeh68,Reference Bobinski, Pielesz and Waksmanska75–Reference Cronqvist, Tannetta and Morgelin86 Demir and colleagues have used TEM to investigate the development of feto-placental villous trees during the early period of ectopic human pregnancy compared to normal pregnancy. Reference Demir, Demir, Ustunel, Erbengi, Trak and Kaufmann80 A decade later, Coan and colleagues investigated the mouse placental exchange region, which included characterising cytoplasmic bridges attached to several areas of the interhaemal membrane. Reference Coan, Ferguson-Smith and Burton59 This was the first study that combined TEM and SEM to determine ultrastructural characteristics of mouse placental development. Reference Coan, Ferguson-Smith and Burton59 Additionally, TEM has contributed to the understanding of placental cellular mechanisms in gas exchange surrounding capillaries, Reference Coan, Ferguson-Smith and Burton59,Reference Sooranna, Oteng-Ntim, Meah, Ryder and Bajoria85 as well as the interior of feto-placental endothelial cells. Reference Cronqvist, Tannetta and Morgelin86 Moreover, TEM has been used to inspect the maldevelopment of the trophoblast and placental terminal villous compartment in human preterm growth-restricted Reference Krebs, Macara, Leiser, Bowman, Greer and Kingdom77 and pre-eclamptic pregnancies, Reference Abdel Salam, Alam, Ahmed and Al-Sherbeny87 illustrating irregular trophoblast surface and elongated villi in preterm intrauterine growth restriction, Reference Krebs, Macara, Leiser, Bowman, Greer and Kingdom77 and excessive villous arborisation with attenuated villi in pre-eclampsia. Reference Abdel Salam, Alam, Ahmed and Al-Sherbeny87
Vascular corrosion casting of feto-placental vasculature can be visualised using SEM. Reference Adamson, Lu and Whiteley40,Reference Whiteley, Pfarrer and Adamson73,Reference Leiser, Krebs, Ebert and Dantzer84 Briefly, a vascular cast is generated with a polymer (i.e. Batson’s casting compound) and after polymerisation, the surrounding tissue is removed with a corrosive agent to reveal the surface of the vascular cast. Reference Adamson, Lu and Whiteley40,Reference Whiteley, Pfarrer and Adamson73,Reference Leiser, Krebs, Ebert and Dantzer84,Reference Sricharoenvej, Tongpob, Lanlua, Piyawinijwong, Roongruangchai and Phoungpetchara88,Reference Bamroongwong, Somana, Rojananeungnit, Chunhabundit and Rattanachaikunsopon89 SEM has been utilised to reveal the intricate connectivity of feto-placental vasculature of healthy pregnancies Reference Detmar, Rennie and Whiteley72,Reference Burton78,Reference Burton79 and complicated pregnancies such as FGR, whereby pronounced variations in placental surface were observed. Reference Detmar, Rennie and Whiteley72,Reference Bobinski, Pielesz and Waksmanska75 SEM has also been used to provide anatomical comparison between humans and rodents, as well as a deeper understanding of how placental structure associates with fetal outcomes. Reference Adamson, Lu and Whiteley40,Reference Rennie, Detmar, Whiteley, Jurisicova, Adamson and Sled52,Reference Detmar, Rennie and Whiteley72–Reference Krebs, Macara, Leiser, Bowman, Greer and Kingdom77 For example, SEM has revealed that the human placentas of FGR pregnancies exhibit abnormalities in their terminal villi compartments, including reductions in numbers of capillary loops, branches and coiled vessels. Reference Krebs, Macara, Leiser, Bowman, Greer and Kingdom77 SEM has been applied to image the transition from arterioles to capillaries in the mouse feto-placental tree and revealed that expansion of the feto-placental vasculature in late gestation is strain dependent. Reference Rennie, Detmar, Whiteley, Jurisicova, Adamson and Sled52 Moreover, SEM revealed that chronic hypoxia during pregnancy in mice caused expansion of the feto-placental capillary bed and thinning of the interhaemal membrane, Reference Cahill, Rennie and Hoggarth74 possibly as a compensatory adaptation to ensure maternal-fetal exchange.
Even though electron microscopy has obvious advantages, especially visualising the feto-placental vasculature (particularly capillary and terminal villi) at extremely high resolutions and high magnifications, it only provides 2D images and also a very restricted field of view of a small segment over the entire vascular network. Moreover, each examination requires demanding sample preparation which destroys the connectivity of the placental vascular networks and the surrounding tissue. Other 3D imaging modalities such as confocal microscopy, light-sheet microscopy and micro-CT may overcome these limitations of electron microscopy.
Confocal microscopy
The development of 3D imaging techniques has altered the scope to study microvasculature with greater accuracy and precision. Confocal microscopy relies on point illumination; the technique utilises a confocal pinhole and a photomultiplier detector to exclude out-of-focus light surrounding the focal plane. This generates a series of 2D optical sections with enhanced contrast, precision and definition. These 2D optical sections can then be combined and reconstructed into a 3D representation which results in high-contrast and high-resolution images. Reference Liu and Chiang90
Confocal microscopy has been applied to human placentas to visualise the architecture of chorionic villous vascularisation in the first trimester, Reference Lisman, van den Hoff, Boer, Bleker, van Groningen and Exalto91 second trimester Reference Merz, Schwenk, Shah, Necaise and Salafia92 and at term. Reference Palaiologou, Etter and Goggin81,Reference Cronqvist, Tannetta and Morgelin86,Reference Plitman Mayo, Abbas, Charnock-Jones, Burton and Marom93,Reference Perazzolo, Lewis and Sengers94 It has also been used to examine the spatial arrangement of the capillary bed. Reference Jirkovska, Janacek, Kalab and Kubinova95,Reference Plitman Mayo, Charnock-Jones, Burton and Oyen96 Confocal has been utilised to investigate the distribution of villous capillaries in human diabetic placentas, including the surface and volume ratios between the fetal capillaries and the villus. It has also been implemented to provide a structural basis for computational modelling of oxygen flux in human placenta. Reference Plitman Mayo, Charnock-Jones, Burton and Oyen96,Reference Jirkovska, Kucera and Kalab97 Recently, Sargent detailed methods of confocal microscopy in rhesus macaques placentas. Reference Sargent, Roberts, Gaffney and Frias98
Although confocal microscopy can be used to visualise the architecture of chorionic villous vascularisation, a major limitation is the maximum depth of focus (~1 mm3), Reference Merz, Schwenk, Shah, Necaise and Salafia92 which means that a full-specimen coverage of the placental vasculature is currently impossible using this technique. Nonetheless, 3D confocal microscopy is still a useful approach for quantifying fine details of placental structure.
Micro-computed tomography
In recent decades, X-ray micro-CT has become a valuable and increasingly popular technique for non-destructive ex vivo imaging and has been used extensively to visualise placental vasculature in 3D, Reference Junaid, Bradley, Lewis, Aplin and Johnstone6–Reference Langheinrich, Vorman and Seidenstucker8,Reference Rennie, Detmar, Whiteley, Jurisicova, Adamson and Sled52,Reference Detmar, Rennie and Whiteley72,Reference Cahill, Rennie and Hoggarth74,Reference De Clercq, Persoons and Napso99–Reference Langheinrich, Wienhard, Vormann, Hau, Bohle and Zygmunt111 as well as other organs including brain, Reference Vasquez, Gao and Su112–Reference Chugh, Lerch and Yu114 heart, Reference Vasquez, Gao and Su112,Reference Jorgensen, Demirkaya and Ritman115 lung, Reference Yang, Yu, Rennie, Sled and Henkelman105,Reference Vasquez, Gao and Su112,Reference Kampschulte, Schneider and Litzlbauer116 liver Reference Op Den Buijs, Bajzer and Ritman117–Reference Kline, Zamir and Ritman119 and kidney. Reference Vasquez, Gao and Su112,Reference Jorgensen, Demirkaya and Ritman115,Reference Nordsletten, Blackett, Bentley, Ritman and Smith120–Reference Marxen, Thornton and Chiarot123 While micro-CT scanning is clearly suitable for imaging bone, blood vessels with low-inherent contrast properties cannot be directly visualised. To overcome this issue, a radio-opaque compound, such as Microfil® (Flow Tech Inc., Carver, MA, USA), Batson’s No.17 (Polysciences Inc., Warrington, PA, USA), BriteVu® (Scarlet Imaging, Murray, UT, USA) or barium sulphate, is perfused into blood vessels to heighten the contrast of the vessel lumen against surrounding tissues. This delineates the vascular structures for qualitative and quantitative analysis. Reference Junaid, Bradley, Lewis, Aplin and Johnstone6,Reference Junaid, Brownbill, Chalmers, Johnstone and Aplin7,Reference Bentley, Ortiz, Ritman and Romero121 In recent years, this approach has been successfully applied to examine placental vascular integrity in mice, Reference Rennie, Detmar, Whiteley, Jurisicova, Adamson and Sled52,Reference Detmar, Rennie and Whiteley72,Reference Cahill, Rennie and Hoggarth74,Reference De Clercq, Persoons and Napso99–Reference Conroy, Silver and Zhong106 rats Reference Bappoo, Kelsey and Parker107,Reference Tongpob, Xia, Wyrwoll and Mehnert108 and humans. Reference Junaid, Bradley, Lewis, Aplin and Johnstone6–Reference Langheinrich, Vorman and Seidenstucker8,Reference Pratt, Hutchinson and Melbourne109–Reference Langheinrich, Wienhard, Vormann, Hau, Bohle and Zygmunt111 Briefly, ex vivo micro-CT of vasculature consists of four main steps: (i) generation of vascular casts using a radio-opaque compound; (ii) ex vivo micro-CT scanning and 3D image reconstruction; (iii) vascular network segmentation and (iv) visualisation and characterisation of vascular network. Reference Tongpob, Xia, Wyrwoll and Mehnert108 Importantly, the field of view using micro-CT is sufficiently large to capture the entire span of feto-placental vasculature in rodents and humans. Moreover, casting approaches for micro-CT can be modified to distinguish arterial and venous networks within the placenta, and the non-destructive nature of the imaging means the same sample can be preserved for follow-up histological assessments.
In mice, the innovative work of Rennie and colleagues using Microfil® and micro-CT has added considerable depth of understanding in the structural changes of feto-placental vasculature. Reference Rennie, Detmar, Whiteley, Jurisicova, Adamson and Sled52,Reference Rennie, Cahill, Adamson and Sled100–Reference Rennie, Detmar and Whiteley102 The developmental course of mouse feto-placental vasculature has been captured, Reference Rennie, Detmar, Whiteley, Jurisicova, Adamson and Sled52,Reference Rennie, Cahill, Adamson and Sled100–Reference Rennie, Detmar and Whiteley102 and comparison between mouse feto-placental arterial and venous trees has been drawn. Reference Rennie, Cahill, Adamson and Sled100 Again, mouse strain differences have been revealed using this technique, such as differences in the expansion of the feto-placental arterial and capillary vasculature in late gestation. Reference Rennie, Detmar, Whiteley, Jurisicova, Adamson and Sled52 Experimental models have also been characterised using this technique. As such, endothelial nitric oxide synthase deficiency reduced feto-placental vascular diameter and lengths in peripheral vessels. Reference Rennie, Rahman, Whiteley, Sled and Adamson101 Also, maternal chronic hypoxic mice showed a significant reduction in the absolute volume of feto-placental arterial vasculature and the total number of vessel branches (−44% and −30%, respectively), when compared to controls. Reference Cahill, Rennie and Hoggarth74 Moreover, environmental pollutant exposure polycyclic aromatic hydrocarbons in C57Bl/6J mice reduced the volume and surface area of the feto-placental arterial vasculature, Reference Detmar, Rennie and Whiteley72 as well as resulting in significantly increased feto-placental arterial vascular tortuosity and reduced intra-placental arteriole vessels. Reference Rennie, Detmar and Whiteley102 These alterations can cause reduced placental blood flow, leading to ineffective maternal-fetal exchange as well as potentially impaired fetal development and outcomes. Further investigations are required however to clarify these associations, as well as the consequences for adult health.
In humans, Pratt and colleagues have evaluated different methodologies in placental preparation and imaging. Reference Pratt, Hutchinson and Melbourne109 They attempted to optimise the application of contrast agent, perfusion pressure, perfusion location and perfusion vessel in tissue preparation. Reference Pratt, Hutchinson and Melbourne109 As such, they found the quality of the feto-placental cast from Microfil® more superior than barium sulphate as well as the contrast injection through umbilical artery more effective than via the umbilical vein or chorionic vessels. Reference Pratt, Hutchinson and Melbourne109 Aughwane and colleagues recently investigated the degree of heterogeneity in human feto-placental vasculature using multiscale micro-CT and histological analysis. Reference Aughwane, Schaaf and Hutchinson110 They found that the vascular density varied throughout placentas, and the patterns of vascular branching differed across a number of normal placentas. Reference Aughwane, Schaaf and Hutchinson110 Langheinrich and colleagues have also analysed the vascular density and surface area of human feto-placental vasculature using micro-CT with barium sulphate perfusion. Reference Langheinrich, Wienhard, Vormann, Hau, Bohle and Zygmunt111 Furthermore, they quantitated feto-placental vasculature in FGR and found arterial vascular volume in FGR was significantly lower than healthy controls. Reference Langheinrich, Vorman and Seidenstucker8 Junaid and colleagues combined two techniques [corrosion casting (Batson’s No.17) and micro-CT] to image the whole human placental vascular morphology in FGR. Reference Junaid, Bradley, Lewis, Aplin and Johnstone6,Reference Junaid, Brownbill, Chalmers, Johnstone and Aplin7 Notably, this study was the first to evaluate the whole human placenta. They detected an alteration of chorionic arterial branching patterns and a reduction in capillary network density in FGR placentas, Reference Junaid, Brownbill, Chalmers, Johnstone and Aplin7 as well as shorter median vessel length density in arteries but longer median vessel length density in veins. Reference Junaid, Bradley, Lewis, Aplin and Johnstone6
We have recently established micro-CT methodology in our laboratory to characterise mouse and rat feto-placental vascular structures. Reference Bappoo, Kelsey and Parker107,Reference Tongpob, Xia, Wyrwoll and Mehnert108 The qualitative assessments of mouse and rat feto-placental vascular structures at the end of gestation revealed striking species differences. Compared to mouse, rat feto-placental vasculature exhibits far greater branching complexity, vascular tree span and tree depth (Fig. 1). While differences in vascular structure are readily apparent qualitatively, obtaining quantitative data has proven more challenging. Despite the excellent work of others, quantitation of these vascular trees has been limited by costly software needed for such analyses. Indeed, Aughwane and colleagues recently also highlighted these methodological issues and barriers in the analysis of human feto-placental vasculature. Reference Aughwane, Schaaf and Hutchinson110 To assist with this, we have recently developed an end-to-end methodology for 3D quantitative characterisation of feto-placental arterial vascularity. Reference Tongpob, Xia, Wyrwoll and Mehnert108 The methodology enables quantification of numerous parameters including tortuosity, vessel diameter, number and order of vessel segments. Reference Tongpob, Xia, Wyrwoll and Mehnert108
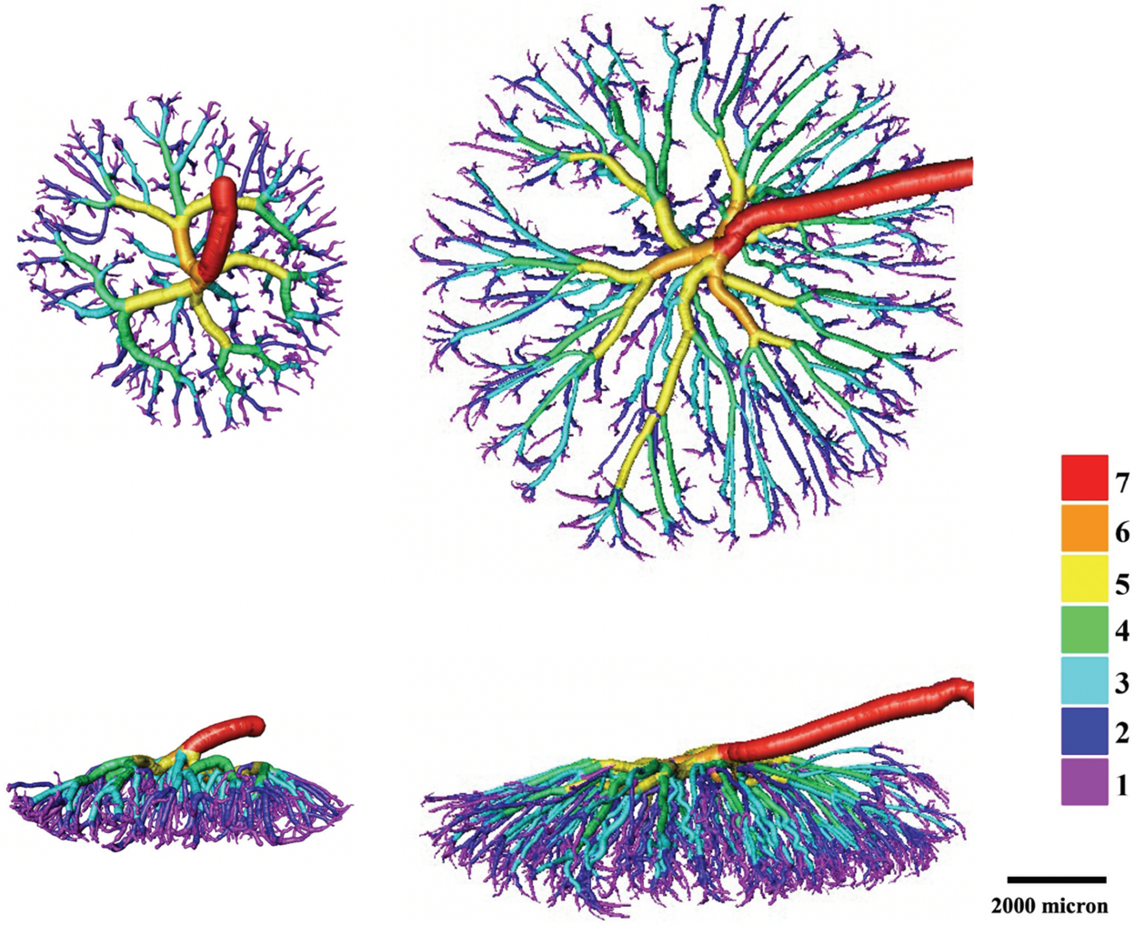
Fig. 1. Visualisation of a feto-placental arterial network at the end of gestation in a mouse (left) and a rat (right). Colour rendering represents different levels of the feto-placental vascular tree. Top row, superior view; bottom row, lateral view; scale bar, 2000 µm.
Beyond assessing the broad expanse of the feto-placental vascular tree, higher resolution scans with a narrower field of view can reveal the capillary structure of the placenta. We have recently assessed this in a rat placenta at the end of gestation, and the capillary tuft can be distinguished in exquisite detail (Fig. 2). Thus, micro-CT approaches may be able to replace electron microscopy and enable investigators a non-destructive way to image capillary networks in their experimental models. Moreover, contrast-enhanced micro-CT (work in progress in our laboratory) can provide potential novel opportunities to visualise greater detailed structures in placental vascular architecture. Iodine Reference Zhou, Cahill, Wong, Seed, Macgowan and Sled124 and zirconium-substituted Keggin polyoxometalate Reference De Clercq, Persoons and Napso99 have also been used on mouse placenta and gross structures can be clearly visualised. However, further work still needs to be developed to explore the full potential of contrast-enhanced micro-CT techniques for assessing placental vasculature.

Fig. 2. High-resolution visualisation of the rat feto-placental capillary tuft at the end of gestation.
Micro-CT is a quantitative 3D imaging technique that is advantageous for investigating 3D placental vascular networks. Evidently, the combination of the contrast agent casting technique and the micro-CT scanning can generate striking images of feto-placental vascular geometry and connectivity in 3D. However, the methodology does involve technical challenges: the casting technique can be difficult, visualisation of data requires considerable computing power and quantitation can be challenging.
Light-sheet microscopy
Light-sheet microscopy has the potential to be a powerful tool for investigating feto-placental vasculature at a macro and micro scale, using either contrast agents or antibodies. However, to our knowledge, little work has been published on this with regard to the placenta. Light-sheet microscopes are traditionally built in-house, require significant computing power for visualisation of the images and optical clearing of the tissue (for a comprehensive review, see Reference Power and Huisken125,Reference Reynaud, Peychl, Huisken and Tomancak126 ). Optical clearance can be challenging for a structure such as the mouse labyrinth zone which is dense with blood vessels. Light-sheet microscopes also traditionally have a relatively small field of view. However, there have been recent exciting developments for tissue clearance and the use of light-sheet microscopy in whole mice, Reference Cai, Pan and Ghasemigharagoz127 mouse eyes, Reference Henning, Osadnik and Malkemper128 mouse hearts, Reference Ding, Bailey and Messerschmidt129 mouse colons, Reference Li, Hui, Hu, Ma, Yang and Tian130 mouse uterine walls, Reference Kagami, Shinmyo, Ono, Kawasaki and Fujiwara131 human brains Reference Mano, Albanese and Dodt132 and human fetal urogenital organs, Reference Isaacson, McCreedy and Calvert133 as well as comparison between different tissue types (i.e. bone, muscle and brain). Reference Buytaert, Goyens, De Greef, Aerts and Dirckx134 With these recent developments, there comes demands for new ways to visualise and quantitate these images. This is a rapidly emerging field and we anticipate that this method will add substantially to our understanding of placental vascular development.
Ultrasonography
None of the imaging modalities discussed thus far are able to assess the feto-placental unit in real time. Indeed, a key challenge for placental research is the ability to accurately monitor placental blood flow in real time, and in particular feto-placental blood flow in vivo with sufficient resolution to yield predictive and diagnostic data. Conventional 2D ultrasonography has been widely used as a primary imaging modality for the assessment of human placental structure and haemodynamics during pregnancy. Reference Abramowicz and Sheiner135–Reference Kingdom, Audette, Hobson, Windrim and Morgen137 Utero-placental vasculature can be identified towards the end of the first trimester, Reference Collins, Birks, Stevenson, Papageorghiou, Noble and Impey138 and there have been recent striking developments in 3D ultrasonography to obtain human placental volumes and overlaying power Doppler ultrasound of the major vasculature. Reference Mathewlynn and Collins139 Moreover, 3D ultrasound has been used to reveal placental volume and vascularity. Reference Mulcahy, Mone and McParland140–Reference Looney, Stevenson and Nicolaides144 These approaches provide not only the opportunity for a screening test for growth restriction but also novel insight into how placental vasculature informs future health outcomes.
With regard to experimental models, preclinical micro-ultrasound has provided useful insight into placental haemodynamics in models commonly used to understand early life origins of adult disease. Reference Wyrwoll, Noble and Thomson18,Reference Rennie, Detmar, Whiteley, Jurisicova, Adamson and Sled52,Reference Rennie, Rahman, Whiteley, Sled and Adamson101,Reference Rennie, Detmar and Whiteley102,Reference Lin, Mauroy, James, Tawhai and Clark145–Reference Lopez-Tello, Barbero and Gonzalez-Bulnes152 For example, using preclinical high-resolution ultrasound, there is evidence for perturbed umbilical cord blood flow and velocity in 11β-HSD2-/- mice. Reference Wyrwoll, Noble and Thomson18 However, this was ameliorated by pravastatin administration and also improved fetal cardiac function. Reference Wyrwoll, Noble and Thomson18 Current resolution of the conventional 2D ultrasound is not adequate for evaluating 3D vascular complexity and fine branching structure. Therefore, the technique cannot investigate the entire extent to which vascular networks are altered in FGR and the downstream effects on fetal outcomes. However, colour Doppler and photoacoustics are emerging as valuable tools for understanding placental haemodynamic changes and structure in real time. At E14.5 in mouse pregnancy, the utero-placental and feto-placental blood supply can be visualised including chorionic plate vessels and intra-placental arterioles. Reference Foster, Hossack and Adamson153 Moreover, these assessments can be taken in conjunction with Doppler measures of fetal heart and other aspects of the fetal circulation including the aorta, inferior vena cava, ductus arteriosus, ductus venosus, inferior vena cava, carotid and pulmonary arteries. Reference Foster, Hossack and Adamson153 Recently, photoacoustic technology has been utilised to image the human placenta in normal and pathologic pregnancy Reference Maneas, Aughwane and Huynh154,Reference Yamaleyeva, Brosnihan, Smith and Sun155 including maternal hypoxia and hyperoxygenation, Reference Arthuis, Novell and Raes156 and pre-eclampsia. Reference Lawrence, Huda and Bayer157 These approaches will provide invaluable insight into how placental and fetal haemodynamic parameters in early life associate and inform adult cardiovascular outcomes.
Magnetic resonance imaging
The technology of MRI has advanced over the past few years to enable assessment of placental structure, blood flow and oxygenation. MRI is a non-invasive, in vivo 3D imaging modality that has been used for fetal and placental investigations and is emerging as a possible clinical tool. For instance, using MRI in obstetrics to assess human placental abnormalities Reference Otake, Kanazawa, Takahashi, Matsubara and Sugimoto158,Reference Allen and Leyendecker159 and to predict fetal volume and birth weight. Reference Damodaram, Story and Eixarch160–Reference Damodaram, Story and Eixarch162 MRI has the advantage of capturing a large field of view, and thus full-specimen coverage, and, in the case of rodent models, multiple placentas. Reference Avni, Neeman and Garbow163 However, in comparison to ultrasound and photoacoustics, there are significant limitations with translation of MRI studies to a clinical setting. These include machine accessibility outside of large clinical research centres, logistics for obese pregnant women undergoing an MRI and feasibility of using MRI to assess fetal oxygenation in high-risk pregnancies. The low resolution of MRI is also not suitable for detailed analysis of placental microvasculature and microcirculation.
Experimentally, MRI is used to reveal placental and fetal development across gestation. Blood oxygen level-dependent MRI (BOLD-MRI) can be used to evaluate changes in feto-placental oxygenation detecting changes in deoxyhaemoglobin concentration during a respiration challenge (i.e. T2*). For example, BOLD-MRI has been used to measure changes in human placental oxygenation during maternal hyperoxia in normal pregnancies Reference Sorensen, Peters, Frund, Lingman, Christiansen and Uldbjerg164,Reference Sorensen, Sinding and Peters165 and FGR pregnancies. Reference Ingram, Morris, Naish, Myers and Johnstone166 Moreover, in rat models of FGR, there is a lower feto-placental response to maternal hyperoxygenation. Reference Aimot-Macron, Salomon and Deloison167,Reference Chalouhi, Alison and Deloison168 Furthermore, dynamic contrast-enhanced MRI (DCE-MRI) with contrast agent intravenous injection (i.e. gadolinium chelate) has been used to quantify placental function such as blood volume, blood flow, oxygenation and perfusion rate in different placental compartments. Reference Kording, Forkert and Sedlacik169 Recently, DCE-MRI has been used to investigate placental perfusion in hypoxic-induced FGR rodents, Reference Alison, Quibel and Balvay170–Reference Deloison, Siauve and Aimot172 pre-eclamptic rats (induced by continuous delivery of L-nitro-arginine methyl ester to chronically inhibit nitric oxide synthase) Reference Lemery Magnin, Fitoussi and Siauve173 and prenatal alcohol exposure in monkeys. Reference Lo, Schabel and Roberts151 Placental blood flow and perfusion rate were reduced significantly in the animal models of hypoxia and ethanol exposure Reference Lo, Schabel and Roberts151,Reference Alison, Quibel and Balvay170,Reference Tomlinson, Garbow, Anderson, Engelbach, Nelson and Sadovsky171 but not in a model of pre-eclampsia. Reference Lemery Magnin, Fitoussi and Siauve173 A combination of DCE-MRI and fluorescence imaging has also been used to delineate and quantify the volume of maternal and fetal compartments in mouse placenta. Reference Solomon, Avni and Hadas174 Therefore, both BOLD-MRI and DCE-MRI can be used for in vivo 3D assessment of placental and fetal function, blood flow and oxygenation. While these approaches are highly useful in an experimental context, it remains to be seen if MRI will transition to being standard use clinically.
Future considerations and opportunities
The capacity to image the placenta, and in particular the feto-placental vasculature, brings opportunities to reconsider models of developmental programming from a different perspective. Blood flow plays a critical role in determining heart and vascular development, yet haemodynamic placental influences have been neglected in consideration as a determinant of adult cardiovascular disease. Through imaging of established experimental models, the development of more specific models to tease out the placental-cardiac axis, and modelling approaches, new context for the early life origins of adult cardiovascular disease should emerge in the next few years. This work should also provoke possibilities of whether placental blood flow can be modified to attenuate cardiovascular disease.
There is no doubt that the various imaging approaches to visualise feto-placental vasculature have yielded useful perspectives for placental and fetal biology. However, with the rapid advances in imaging techniques, there comes the need for computational power and technology to acquire, store, display, visualise and analyse such complex images. To advance this field, it is critical that an interdisciplinary research community is developed to collaborate not only on imaging techniques but also on software and computation resources. Alongside reaching consensus on best practice and standardisation, making open-source software available to the whole research community will be pivotal.
Though beyond the scope of this review, it is important to highlight the rapid emergence of modelling approaches in feto-placental vasculature. These have been made possible due to the technical advances in imaging techniques and have enabled several groups to utilise either engineering or mathematical modelling approaches in order to couple structure with function. Reference Rennie, Detmar, Whiteley, Jurisicova, Adamson and Sled52,Reference Cahill, Rennie and Hoggarth74,Reference Perazzolo, Lewis and Sengers94,Reference Plitman Mayo, Charnock-Jones, Burton and Oyen96,Reference Rennie, Cahill, Adamson and Sled100–Reference Rennie, Detmar and Whiteley102,Reference Bappoo, Kelsey and Parker107,Reference Lin, Mauroy, James, Tawhai and Clark145,Reference Erlich, Nye, Brownbill, Jensen and Chernyavsky175–Reference Clark, Lin, Tawhai, Saghian and James185 The ex vivo dual perfusion of the human placenta, in vivo MRI and ultrasonography (including photoacoustics) being developed in parallel will provide rich means of validation for computational models in predicting placental function, particularly oxygen transfer. This interdisciplinary placental research community is thus at a juncture of developing some important modelling tools for clinical application to help predict and manage complicated pregnancy, including FGR. Moreover, these tools will be invaluable in understanding the influence of early life environment on development, in particular the haemodynamic implications of altered placental vascular structure on fetal tissues, and how adverse health outcomes in later life may be prevented.
Conclusions
Placental function and structure in relation to DOHaD are still poorly understood, particularly in how feto-placental vascular structure affects placental function and fetal cardiovascular development. Although multiple imaging modalities can be used to assess feto-placental structure, each has its limitations. With the new array of imaging techniques, technology, software, computation development and modelling approaches becoming available, the field can move forward at a rapid pace. However, the speed of progress will rely on interdisciplinary and cross-research group collaborations, particularly in establishing open access ways to visualise and analyse data. Through this, the field is poised to deepen the understanding of placental development and function, and fetal health outcomes in early and adult life.
Acknowledgements
The authors thank Tim Crough for the 3D feto-placental capillary imaging.
Financial Support
Micro-CT work was supported by the Raine Medical Research Foundation and Western Australian Department of Health. YT is supported by a Naresuan Scholarship and a Thai Government Science and Technology Scholarship. The authors acknowledge the facilities and scientific and technical assistance of the National Imaging Facility and Microscopy Australia, both National Collaborative Research Infrastructure Strategy (NCRIS) capabilities, at the Centre for Microscopy, Characterisation and Analysis, The University of Western Australia.
Conflict of Interest
The authors declare no conflict of interest.
Ethical Standards
The animal experimental procedures which resulted in the feto-placental arterial vascular casts were approved by the Animal Ethics Committee of the University of Western Australia (UWA): approval numbers RA/3/100/1600 and RA/3/100/1393.