Introduction
The term ‘extremophile’ usually evokes images of prokaryotes living in acidic hot springs or in the Antarctic Dry Valleys, environments very different from the human environment. However, the taxonomic range of extremophilic organisms spans all three domains, including multicellular organisms and vertebrates (Rothschild & Mancinelli Reference Rothschild and Mancinelli2001; Clegg & Trotman Reference Clegg, Trotman, Abatzopoulos, Beardmore, Clegg and Sorgeloos2002). For example, hydrothermally formed chimneys harbour dense and diverse microbial and multicellular communities, including luxuriant populations of clams (e.g. Calyptogena magnifica), mussels (e.g. Bathymodiolus thermophilus), tube worms (e.g. Riftia pachyptila, Tevnia jerichonana) and a variety of grazers, which are supported by the high chemosynthetic primary production of the hydrothermal vents (Turner Reference Turner1886). These systems are usually hosted in peridotites, an environment which is not only high in temperature and in pressure, but also very reducing and associated with acidic pH conditions. Other extreme environments useful to understand the range of environments in which terrestrial life can thrive include studies of winter sea-ice (Krembs et al. Reference Krembs, Deming, Junge and Eicken2002), active sulphide chimneys (Kelley et al. Reference Kelley, Baross and Delaney2002) and the acidic, iron-rich river, Rio Tinto, in southern Spain (Zettler et al. Reference Zettler, Gomez, Zettler, Keenan, Amils and Sogin2002). The study of these types of environments and an associated survey of the full biodiversity will provide an essential learning tool for the exploration of extraterrestrial life on other planetary bodies and shed light on evolutionary trajectories towards multicellular complexity (Schulze-Makuch & Irwin Reference Schulze-Makuch and Irwin2004). In addition, the study of extremophiles will contribute to our understandings of mechanisms of organismic survival, the origin of life and the limits for life in the universe (Rothschild Reference Rothschild, Pudritz, Higgs and Stone2006).
Adaptation strategies to environmental stresses by multicellular organisms
Adaptation in a biological sense is a genetically controlled characteristic that increases an organism's ability to survive and reproduce in its environment. Stresses to which organisms have evolved adaptations include dehydration (anhydrobiosis), extreme temperature (thermobiosis) including freezing temperatures (cryobiosis) and lack of oxygen (anoxia) (Hochachka & Somero Reference Hochachka and Somero1984, Reference Hochachka and Somero2002). In 1702 van Leeuwenhoek observed inactive ‘animalcules’ (tardigrades and rotifers) in dry sediments from the gutters of roofs of houses (Keilin Reference Keilin1959; Wright Reference Wright2001). Shortly after the organisms came into contact with water they started to move. A similar ability of organisms to tolerate dehydration was reported by Needham (Reference Needham1743), Spallanzani (Reference Spallanzani1776) and Doyère (Reference Doyère1842). This phenomenon is referred to here as cryptobiosis (latent or hidden life) and anhydrobiosis is a special case of adaptation to dehydration. It is defined as the state of an organism when the organism shows no visible signs of life and when its metabolic activity is hardly measurable or comes reversibly to a standstill (Keilin Reference Keilin1959; Takamatsu et al. Reference Takamatsu1997).
Cryptobiosis is a form of quiescence, which is induced and maintained directly by the occurrence of adverse conditions (such as desiccation, freezing and oxygen deficiency) for active life, and promptly broken once the adverse conditions are removed (Hand Reference Hand1991; Keilin Reference Keilin1959). Cryptobiosis is one particular adaptation against environmental stresses, but should not be confused with diapause. The latter at the larval, pupal and adult stages is arrested development resulting from reduced hormonal activities, which may persist even when favourable environmental conditions prevail (Tauber & Masaki Reference Tauber and Masaki1968; Danks Reference Danks1987).
Cryptobiosis can be used as a generic term for ametabolism, and further subdivided into five categories based on the factors that induce it (Fig. 1): cryobiosis (induced by freezing), thermobiosis (low and high temperatures), osmobiosis (extremely high osmolarity), anoxybiosis (lack of oxygen) and anhydrobiosis (lack of water) (Wright et al. Reference Wright, Westh and Ramløv1992; Takamatsu et al. Reference Takamatsu1997). At the organismic level, the distinction between anhydrobiosis, cryobiosis and osmobiosis is often unclear because more than one process may be involved in the entry of cryptobiosis. For instance, low temperatures inducing extracellular freezing in the organism will also have a desiccating effect on the cells (Jönsson Reference Jönsson2003).

Fig. 1. Classification of cryptobiosis based on environmental stresses to be overcome. Note that some other authors (Keilin Reference Keilin1959; Wright et al. Reference Wright, Westh and Ramløv1992; Takamatsu et al. Reference Takamatsu1997) list osmobiosis and cryobiosis as separate processes.
Anhydrobiosis
Anhydrobiosis is the most common form of cryptobiosis, and anhydrobiotic organisms are able to survive almost complete dehydration; 95–99% of the water content is lost in many cases (Drinkwater & Crowe Reference Drinkwater and Crowe1991; Danks Reference Danks2000). Desiccation tolerance is exhibited by many life forms and found in many taxa ranging from unicellular organisms to higher invertebrates and plants, such as plant seeds, vegetative tissues of higher plants (resurrection plants), yeasts, bacteria, fungal spores, protozoans, eggs of turbellaria, nematodes, rotifers, tardigrades, springtails, cysts of primitive crustaceans including the brine shrimp (Artemia salina) and larvae of a chironomid midge (e.g. Polypedilum vanderplanki); see Takamatsu et al. (Reference Takamatsu1997), Ricci (Reference Ricci.1998), Scott (Reference Scott2000), Clegg (Reference Clegg2001) and Wharton (Reference Wharton2002). Anhydrobiosis is commonly identified by morphological change. The coiling of the body of soil nematodes such as Scottnema lindsayae, Eudorylaimus antarcticus and Plectus antarcticus accompanying the physiological changes have been used to identify anhydrobiotic individuals in field studies (Townshend Reference Townshend1984; Freckman et al. Reference Freckman, Whitford and Steinberger1987; Treonis et al. Reference Treonis, Wall and Virginia2000) and in the laboratory (Higa & Womersley Reference Higa and Womersley1993a). One of the common features of anhydrobiotic stages is limited size, and most of the invertebrates are less than 1 mm in size. No anhydrobiotic species have been found in vertebrates (Takamatsu et al. Reference Takamatsu1997). However, in many anhydrobiotic plants, this stage is not restricted to small size.
Concerning the issue of size variations between anhydrobiotic animals and plants, Alpert (Reference Alpert2006) has demonstrated that desiccation-tolerant animals may be small in size because of physical stresses associated with drying. Animal cells shrink as a result of drying and the whole animal must shrink with it. All animals that tolerate anhydrobiosis or desiccation as adults adopt distinctive or curled shapes as they dry. Rigid external or internal skeletons would prevent the curling. Some tolerant animals have exoskeletons, but their tolerance is restricted to juvenile stages before skeletons form.
Plant communities have a greater ability than animals to combine tolerance and rigidity. The leaves of desiccation-tolerant plants often shrink or fold as they dry, but the length of the stems appears to remain unaffected. This is possible because each plant cell has its own exoskeleton and a rigid cell wall. Physical stress probably does not compound across groups of cells as readily in plants as in animals. Some tolerant plants perform various specialized traits that reduce the shrinkage of cells away from their walls or increase the ability of the wall to fold or bend as the cell shrinks (Schneider et al. Reference Schneider, Wistuba, Wagner, Thurmer and Zimmermann2000).
Longevity in the anhydrobiotic state varies with the type of organism, but many species can remain viable for significant time periods. For example, there have been reliable demonstrations of the longevity of anhydrobiotic invertebrates after laboratory preservation including a 39-year-survival of the nematode Tylenchus polyhypnus (Steiner & Albin Reference Steiner and Albin1946). The longest records of recovery from the anhydrobiotic state for insects is 17 years, for crustaceans 16 years, and for tardigrades and rotifers 9 years (Baumann Reference Baumann1922; Clegg Reference Clegg2001; Bertolani et al. Reference Bertolani, Guidetti, Jonsson, Altiero, Boschiini and Rebecchi2004). However, survival periods may be much longer. There are reports that tardigrades and rotifers were recovered from a dry moss sample taken from an Italian museum after 120 years of preservation, although the individuals underwent ‘quivers in several zones of their body’ (Franceschi Reference Franceschi1948; Jönsson Reference Jönsson2003).
Anhydrobiotic organisms can express extremely high tolerances against various kinds of stresses. Gavaret (Reference Gavaret1859) showed that rotifers and tardigrades could survive being kept in a vacuum. Artemia cysts are often preserved in a vacuum and nitrogen gas packs to maintain high recovery rates. Artemia cysts have also been exposed to the conditions of outer space with negligible mortality (Gaubin et al. Reference Gaubin, Planel, Gasset, Pianezzi, Delpoux, Clegg, Kovalev, Nevzgodina, Maximova and Miller1983). Anhydrobiotic tardigrades can tolerate extreme temperatures down to −270°C, immersion in saturated brine and organic solvents, and extremely high hydrostatic pressures (600 MPa) (Rahm Reference Rahm1921; Keilin Reference Keilin1959; Seki & Toyoshima Reference Seki and Toyoshima1998).
For more information and recent results on anhydrobiosis, the reader is referred to the published proceedings of a symposium on ‘anhydrobiosis’, which appeared in Integrative and Comparative Biology in 2005 (45, 683–820) with a total of 16 individual contributions.
Biochemical changes during anhydrobiosis
Cells of organisms that are naturally adapted to survive severe stress usually contain large amounts of low-molecular-mass ‘compatible solutes’ (Yancey et al. Reference Yancey, Clark, Hand, Bowlus and Somero1982; Somero & Yancey Reference Somero and Yancey1997), which will also play important protective roles. Among these solutes, trehalose, the disaccharide of glucose, plays a considerable role (Drinkwater & Crowe Reference Drinkwater and Crowe1991; Eleutherio et al. Reference Eleutherio, Araujo and Panek1993; Clegg Reference Clegg2001). Trehalose accumulates in anhydrobiotic organisms, such as unicellular organisms (bacteria, yeast and spores of fungi), invertebrates (chironomids, tardigrades, nematodes and encysted Artemia) and resurrection plants (Yancey et al. Reference Yancey, Clark, Hand, Bowlus and Somero1982; Ingram & Bartels Reference Ingram and Bartels1996; Takamatsu et al. Reference Takamatsu1997; Clegg Reference Clegg2001; Tunnacliffe & Lapinski Reference Tunnacliffe and Lapinski2003), whereas other disaccharides, mainly sucrose, are present in seeds and pollen grains of higher plants (Drinkwater & Crowe Reference Drinkwater and Crowe1991; Behm Reference Behm1997; Alpert Reference Alpert2000). Most of these organisms accumulate a large amount of trehalose during dehydration, about 10–20% of their dry body weight (Takamatsu et al. Reference Takamatsu1997).
It has been demonstrated that this sugar protects the desiccation-linked damage in organisms (Drinkwater & Crowe Reference Drinkwater and Crowe1991; Allison et al. Reference Allison, Chang, Randolph and Carpenter1999; O'Hara et al. Reference O'Hara, Watson, Srere, Kumar, Wiler, Welch, Bitting, Heller and Kilduff1999). Trehalose has a high solubility, a low reactivity and a low tendency to crystallize. It is a non-reducing sugar, and so is less harmful to cells and tissues than reducing sugars such as glucose, even at extremely high concentrations (Drinkwater & Crowe Reference Drinkwater and Crowe1991; Pannewitz et al. Reference Pannewitz, Schlensog and Green2003). It substitutes for bound and free water, and thus maintains the structures of cell membranes and proteins. At the same time trehalose leads to metabolic shutdown.
On the other hand, anhydrobiosis without trehalose has been reported in bdelloid rotifers by Tunnacliffe & Lapinski (Reference Tunnacliffe and Lapinski2003). The rotifers Philodina roseola and Adineta vaga gave surprising results as a response to desiccation stress concerning sugar accumulation. No trehalose at all was observed in the analytical results and no other simple sugar was produced in detectable quantities during dehydration. Even trehalose synthase genes were not found in the rotifer genomes. This first observation of animal anhydrobiosis without trehalose thus challenges our present understanding and requires a re-thinking of existing models.
Higa & Womersley (Reference Higa and Womersley1993b) reported that the accumulation of trehalose in itself is not sufficient for the induction of anhydrobiosis in Aphelenchus avenae. Also, species of Di. myceliophagus varying in trehalose content ranging from 3 to 16% dry weight were not able to survive direct exposure to low humidity (Womersley et al. Reference Womersley, Wharton, Higa, Perry and Wright1998).
Thus, it is likely that other or additional biochemical changes are required for anhydrobiosis. Heat shock proteins (Hsps) are good candidates, because they can serve as molecular chaperones. The Hsp70 family and the Hsp60 chaperon complexes (the classical molecular chaperones) are commonly perceived as ‘heat shock proteins’. They are up-regulated by heat stress, although they also play a vital role in folding nascent proteins under non-stress conditions (Ellis & Hartl Reference Ellis, Hartl and Cooper2003). These molecules were reported to participate in unfolding and relocalization of proteins damaged by stresses, to assist the folding of newly synthesized proteins, to protect them from denaturation and aggregation and aid in their renaturation, and to influence the final intracellular location of mature proteins (Ellis & Hartl Reference Ellis and Hartl1999; MacRae Reference MacRae2000). Hsps are also involved in tolerance against various other stresses such as low and high temperatures, oxidation, anoxia and heavy metals.
The function of Hsps as stress combatants is to potentiate refolding of polypeptide structures which have become partially denatured. Besides such ‘folding’ chaperones, both the eukaryotic and prokaryotic cytoplasm also contains ‘holding’ and ‘disaggregating’ chaperones. Holding chaperones include small Hsps which form large multimeric ‘windowed’ complexes capable of passively stabilizing protein species in a partially unfolded state, preventing aggregation until stress has abated, and refolding by Hsp70 and Hsp60 teams (e.g. Haslbeck et al. Reference Haslbeck, Walke, Stromer, Ehrnsperger, White, Chen, Saibil and Buchner1999). Disaggregating chaperones include the Hsp100 family, which is thought to disentangle protein aggregates and to serve as clients for folding chaperones (Ben-Zvi & Goloubinoff Reference Ben-Zvi and Goloubinoff2001).
In fact, one type of small Hsp, p26 and artemin, may be involved in stress resistance in Artemia cysts (Willsie & Clegg Reference Willsie and Clegg2002; Chen et al. Reference Chen, Amons, Clegg, Warner and MacRae2003; Collins & Clegg Reference Collins and Clegg2004; Warner et al. Reference Warner, Brunet, MacRae and Clegg2004), and p26 also appears to act synergistically with trehalose in vitro (Viner & Clegg Reference Clegg2001). Goyal et al. (Reference Goyal, Browne, Burnell and Tunnacliffe2005a, Reference Goyal, Walton, Browne, Burnell and Tunnacliffeb, Reference Goyal, Walton and Tunnacliffec) characterized genes in the nematode Aphelenchus avenae, which requires a period during which water loss is slow and limited before the animal becomes fully desiccation tolerant. This is probably to allow time to switch on a critical set of genes, which can be identified by their dehydration-responsive nature. During this preconditioning period, trehalose synthase genes are induced (Goyal et al. Reference Goyal, Browne, Burnell and Tunnacliffe2005a) and trehalose biosynthesis occurs (Madin & Crowe Reference Madin and Crowe1975). Of other up-regulated genes identified to date, two encode ‘hydrophilins’ (highly hydrophilic proteins; Garay-Arroyo et al. Reference Garay-Arroyo, Colmenero-Flores, Garciarrubio and Covarrubias2000): a novel protein called anhydrin, and a polypeptide, Aav-LEA-1, related to plant Group 3 late embryogenesis abundant (LEA) proteins (Browne et al. Reference Browne, Tunnacliffe and Burnell2002, Reference Browne, Dolan, Tyson, Goyal, Tunnacliffe and Burnell2004).
The widespread occurrence of LEA and other hydrophilic proteins in desiccation-tolerant or -resistant systems is consistent with combating damage due to water loss. Other functions for LEA proteins, besides that of preventing protein aggregation, are also possible and it has been suggested that they might be multi-functional, with one potential role as storage proteins in plants (Goyal et al. Reference Goyal, Walton, Browne, Burnell and Tunnacliffe2005b). Some LEA or LEA-like proteins are known to associate with cell membranes and have been shown to prevent liposome leakage on desiccation (Sales et al. Reference Sales, Brandt, Rumbak and Lindsey2000). LEA proteins could also act as ‘molecular shields’ to physically prevent or reduce the formation of protein aggregates (Goyal et al. Reference Goyal, Walton and Tunnacliffe2005c). It has been proposed that folding of LEA proteins on membranes occurs in a manner similar to that proposed for alpha-synuclein, which has a role in vesicle management (Koag et al. Reference Koag, Fenton, Wilkens and Close2003). LEA protein accumulation has been reported in plant seeds and tissues of resurrection plants, associated with the acquisition of desiccation tolerance during maturation (Ingram & Bartels Reference Ingram and Bartels1996; Cuming Reference Cuming, Shewry and Casey1999; Scott Reference Scott2000; Clegg Reference Clegg2001). Expression of LEA is induced by drought, low temperature and high salt content (Ingram & Bartels Reference Ingram and Bartels1996; Cuming Reference Cuming, Shewry and Casey1999), and confers increased osmotic or freezing tolerance to the yeast Saccharomyces cerevisiae (Imai et al. Reference Imai, Chang, Ohta, Bray and Takagi1996; Zhang et al. Reference Zhang, Ohta, Takagi and Imai2000). A barley LEA improves drought tolerance in transgenic plants including rice and wheat (Xu et al. Reference Xu, Duan, Wang, Hong, Ho and Wu1996; Sivamani et al. Reference Sivamani2000). A LEA-like protein (Hsp12) is also suggested to be involved in protection of membrane proteins (Sales et al. Reference Sales, Brandt, Rumbak and Lindsey2000). Group 3 LEA protein homologues were found in the nematodes Caenorhabditis elegans, Steinernema feltiae and A. avenae, and in the prokaryotes Deinococcus radiodurans, Bacillus subtilis and Haemophilus influenzae (Postnikova et al. Reference Postnikova, Tselikova, Kolaeva and Solomonov1999; Dure Reference Dure2001; Browne et al. Reference Browne, Tunnacliffe and Burnell2002).
All groups of LEA proteins (1, 2, 3 and 6) were suggested to act as molecular chaperones (Imai et al. Reference Imai, Chang, Ohta, Bray and Takagi1996; Wise & Tunnacliffe Reference Wise and Tunnacliffe2004). Wise & Tunnacliffe (Reference Wise and Tunnacliffe2004) proposed a possible function of LEA proteins (group 3) during induction of anhydrobiosis. Natively unfolded LEA proteins exhibit a strong alpha-helical component during desiccation. The superhelical structures form coils and filaments associated with the cytoskeleton, and finally generate intracellular filament networks within the dehydrating cells. This configuration might increase mechanical strength. LEA proteins also act synergistically with trehalose as an aggregation protectant for several kinds of enzymes (Goyal et al. Reference Goyal, Walton, Browne, Burnell and Tunnacliffe2005a, Reference Goyal, Browne, Burnell and Tunnacliffeb, Reference Goyal, Walton and Tunnacliffec). Hsps and LEA proteins have been found in broad taxa of anhydrobiotic organisms from plants to invertebrates, and seem to have important roles for the induction and maintenance of anhydrobiosis. However, the protective roles have not been demonstrated in invertebrates. Further analysis of the behaviour of proteins during water stress is required to elucidate the function of LEA proteins in vivo during anhydrobiosis. Thus, anhydrobiosis appears to be a complex physiological response requiring the interplay of several different gene products and metabolites, which needs to be further elucidated by future molecular studies.
Induction of anhydrobiosis
Although the brain is the common prime regulator of larval, pupal and adult diapause in insects (Denlinger Reference Denlinger, Kerkut and Gilbert1985), this does not seem to be the case for anhydrobiosis. In P. vanderplanki larvae, anhydrobiosis occurs without the brain: larvae without a head accumulate relatively large amounts of trehalose during desiccation and recover after rehydration like intact larvae (Takamatsu et al. Reference Takamatsu1997). Furthermore, body parts mainly consisting of fat body tissues can synthesize a large amount of trehalose during desiccation, and the fat body can recover after long-term dry preservation (Takamatsu et al. Reference Takamatsu1997). Therefore, the central nervous system is not involved in the induction of anhydrobiosis, and individual cells and tissues themselves could enter the anhydrobiotic state in the chironomid. Trehalose content in anhydrobiotic organisms ranges from 2% in tardigrades to 40% in yeast. Trehalose cannot easily enter cells and tissues because of its relatively large molecular size. Recently, it has been shown that the presence of intracellular trehalose at a high level contributes to an increase of desiccation tolerance in vertebrate cells: human primary fibroblasts intracellularly producing trehalose (due to a recombinant adenovirus vector) could be maintained in a completely dry state for 3 days (Guo et al. Reference Guo, Puhlev, Brown, Mansbridge and Levine2000). These cells accumulated only 1 mM of trehalose intracellularly. Freeze-dried human and mammalian platelets (apyrene) could recover after rehydration only when they were loaded intracellularly with a relatively high concentration of trehalose (20 mM) before freeze-drying (Wolkers et al. Reference Wolkers, Walkers, Tablin and Crowe2001, Reference Wolkers, Tablin and Crowe2002). On the other hand, mouse cells containing 10% trehalose, through expressing trehalose phosphate synthase intracellularly, could not survive complete desiccation, although they had increased tolerance to high osmolarity (Garcia-de-Castro & Tunnacliffe Reference Garcia-de-Castro and Tunnacliffe2000). It is still not determined whether the importance of intracellular trehalose reported in vertebrate cells is applicable to cells, tissues and individuals of invertebrates in the anhydrobiotic state. Physiological processes involved in the induction and emergence of anhydrobiosis in invertebrates are described in detail in Womersley et al. (Reference Womersley, Wharton, Higa, Perry and Wright1998) and Takamatsu et al. (Reference Takamatsu1997).
Ma et al. (Reference Ma2005) have compared cellular viabilities following drying and rehydration, and demonstrated that trehalose and p26 improved cellular viability. They reported that the 293H cells lacking p26 and trehalose indicated the greatest sensitivity to water loss, decreasing viability at high water content. E11'L cells expressing p26, but lacking trehalose, showed a negligible improvement at the lowest water content. The survival rate of trehalose-loaded 293H cells was higher at a lower water content. E11'L cells expressing p26 and loaded with trehalose indicated major improvement in survival (at least a 20% increase in viability) over all other treatments. This phenomenon positively indicated that both trehalose and p26 produce significant advantages in the ability of the cells to survive drying. Optimal protection was achieved in the presence of both trehalose and p26, suggesting a synergistic mechanism.
Resurrection plants as an example organism using anhydrobiosis
A group of higher plants known as poikilohydric or resurrection plants possesses a uniquely effective mechanism for coping with drought stress. All plant species are at least partially tolerant to desiccation (i.e. seeds and pollen; Bewley Reference Bewley1979), but the ability of mature tissue, such as roots and leaves, to survive virtually complete desiccation is very rare. Resurrection plants survive the loss of most of their tissue water content until a quiescent stage is achieved. Upon watering the plants rapidly revive and are restored to their former state (Fig. 2). This resurrection capacity is not restricted to the main meristems of these plants. Fully mature leaves can lose up to 95% of their water content and then, upon rewatering, are rehydrated and are fully photosynthetically active within 24 hours (Bernacchia et al. Reference Bernacchia, Salamini and Bartels1996). Tissue damage through this drying and rehydration process appears to be minimal to non-existent. The plant is preserved until water becomes available. At this point it is ready to take immediate advantage of the new conditions. Thus, a resurrection plant has a great competitive advantage over other species in certain ecological niches.

Fig. 2. Dehydration and rehydration phases of resurrection in Craterostigma plantagineum. On day 1 watering ceased (A). Photographs were taken of the same plant (Scott Reference Scott2000).
Most resurrection plant species are native to arid climates in the world such as southern Africa, southern America and western Australia (Gaff Reference Gaff1977, Reference Gaff1987). Resurrection plants inhabit ecological niches that are subjected to lengthy periods of drought with periods of rain during the year. They are frequently found as denizens of rocky outcrops and grow in shallow, sandy soils (Sherwin & Farrant Reference Sherwin and Farrant1996). Their habitats often take the form of depressions on rock outcrops which have the capacity to form ephemeral pools (Gaff Reference Gaff1971; Gaff & Giess Reference Gaff and Giess1986). The growth and reproduction of the plants occurs in rainy seasons, but upon drying the plants can remain dormant for considerable time periods. The aquatic plant Ch. intrepidus has been known to survive for up to 11 months in the dehydrated state (Hartung et al. Reference Hartung, Schiller and Karl-Josef1998). However, this period is short compared to species of Craterostigma, which have been known to last at least 2 years without water, and probably much longer (Gaff & Wood Reference Gaff and Wood1988; Gaff Reference Gaff, Kreeb, Richter and Hinckley1989). The survival mechanisms in these plants protecting them from dehydration are frequently also effective against other environmental stresses including high salt content (Gaff & Wood Reference Gaff and Wood1988; Gaff Reference Gaff, Kreeb, Richter and Hinckley1989) and heat (up to 60°C; Hartung et al. Reference Hartung, Schiller and Karl-Josef1998). Some other organisms specialized in utilizing anhydrobiosis are listed in Table 1.
Table 1. Some example organisms using anhydrobiosis as a survival mechanism

Sherwin & Farrant (Reference Sherwin and Farrant1996) have studied the mechanisms of avoidance and protection against light damage in the resurrection plants Craterostigma wilmsii and Xerophyta viscosa. In C. wilmsii, a combination of both physical and chemical changes appeared to afford protection against free radical damage. The leaf tissue became purple/brown in colour during dehydration, which coincided with a three-fold increase in anthocyanin content and a 30% decline in chlorophyll content. Thus, light-chlorophyll interactions were progressively reduced as chlorophyll became masked by anthocyanins in abaxial layers and shaded in the adaxial layers. Ascorbate peroxidase activity increased during this process but declined when the leaf was desiccated. During rehydration, superoxide dismutase and glutathione reductase activities increased markedly, possibly affording free radical protection until full hydration and metabolic recovery had occurred.
Scott (Reference Scott2000) demonstrated that the roots (being in the soil) are most likely to detect a drop in water availability first. Abscisic acid synthesis and release by roots is a common response in plants to drought stress. Once released, abscisic acid could activate batteries of genes required for metabolic processes such as the accumulation of sucrose from either stored carbohydrates or through an alteration in photosynthetic carbon partitioning. In addition, the synthesis of other proteins would be induced by dehydrins and LEAs, which could help to stabilize the plant cells as they lose water.
As the tissues dehydrate, leaves shrink, chlorophyll is degraded, sucrose accumulates, the xylem cavitates eventually and the plants become desiccated. On addition of water, the xylem refills and cells begin to take up water and expand. Enzymes present in the tissues are activated, sucrose is metabolized and chlorophyll is resynthesized. Within 24 hours the plant is restored and is reproductively active within 2 weeks.
Ndima et al. (Reference Ndima, Farrant, Thomson and Mundree2001) have studied Xerophyta viscosa, which is a monocotyledonous resurrection plant that is capable of tolerating extreme desiccation conditions. Upon rewatering, it rehydrates completely and assumes its full physiological activities. They conducted studies on changes in gene expression associated with dehydration stress tolerance. A cDNA library was constructed from mRNA isolated from dehydrated X. viscosa leaves. XVT8 represents one of 30 randomly selected clones that were differentially expressed when X. viscosa was dehydrated. Sequence analysis of XVT8 revealed that it exhibited 45% and 43% identity to dehydrin proteins from Rabidopsis thaliana and Pisum sativum, respectively, at the amino acid level. XVT8 encoded a glycine-rich protein (27 kDa), which is largely hydrophilic and contains a hydrophobic segment at the C-terminus. Southern blot analysis confirmed the presence of XVT8 in the X. viscosa genome. XVT8 transcripts accumulated in X. viscosa plants that were exposed to heat, low temperature and dehydration stresses, and to exogenous abscisic acid and ethylene. The high sequence identity observed with known dehydrins, the presence of the conserved serine- and lysine-rich motifs, and the hydropathy analysis data clearly supported the view that XVT8 is a dehydrin. These results provided direct evidence for the dehydration, low temperature, heat, abscisic acid and ethylene dependent regulation of the XVT8 gene in X. viscosa.
Osmobiosis (as a special case of anhydrobiosis)
Osmobiosis is a form of cryptobiosis induced by elevated osmotic pressures. Some intertidal marine species and euryhaline limno-terrestrial species can tolerate variations in salinity. However, most freshwater and terrestrial tardigrades form contracted tuns (barrel-shaped resistant forms) in various salt solutions (Wright et al. Reference Wright, Westh and Ramløv1992). Induction of the dormant state by evaporative dehydration is called ‘anhydrobiosis’ and when water is removed by osmotic dehydration it is called ‘osmobiosis’ (Perry Reference Perry, Glazer, Richardson, Boemare, Coudert and Reiman1998). Thus osmobiosis can be understood as a special case of anhydrobiosis. It also involves a cessation of metabolism.
Chen et al. (Reference Chen, Gollop and Glazer2005) studied the entomopathogenic nematode Steinernema feltia. They discovered a novel accumulated protein, GroEL, and also found that the protein GroES was up-regulated in the osmotically desiccated infective juveniles (IJs) compared with non-desiccated IJs under osmotic stress. These proteins may act to repair damaged proteins or assist in the maintenance of homeostasis under osmotic stress. This hypothesis is consistent with the previous observations that GroEL and GroES are members of the Hsp60 family of chaperons (Hennequin et al. Reference Hennequin, Collignon and Karjalainen2001). The osmobiotic state of the nematodes was associated with the accumulation of a significant amount of stress-related proteins. The results showed that all changes in protein abundance depended on their synthesis and breakdown, or on the up-regulation of the osmotic process. Genomic and proteomic tools need to be employed to decipher the induction process of osmobiosis.
The brine shrimp Artemia is the best example of an organism that can withstand large salt contents (Fig. 3). Artemia cysts will survive osmotic stress, ultraviolet radiation, temperature extremes and anoxia. Brine shrimps occur more or less in every continent except Antarctica. However, their habitat range is restricted. Since Artemia is highly vulnerable to predation, they are found in habitats where ionic concentrations are high enough to avoid fish and other invertebrates. The general habitats include terminal, inland salt lakes and coastal salterns associated with commercial salt production. Artemia sp. survive in a wider range of ionic concentrations than any other metazoan (Browne & MacDonald Reference Browne and MacDonald1982). Artemia's survival abilities are enhanced by a protective cyst wall, the accumulation of trehalose and glycerol, the protein artemin and the heat-stress proteins, which act as molecular chaperons (Wharton Reference Wharton2002). Encysted embryos of the primitive crustacean Artemia franciscana are among the most resistant of all multicellular eukaryotes to environmental stress, in part due to massive amounts of a small heat shock/a-crystallin protein (p26). These embryos also contain very large amounts of the disaccharide trehalose, well known for its ability to protect macromolecules and membranes against damage due to water removal and temperature extremes. Drinkwater & Crowe (Reference Drinkwater and Crowe1991) suggested that the carbohydrate metabolism, which involves the conversion of trehalose to glycerol and is required for hatching, responds to increasing salinity as reported in Artemia species. They concluded that increasing amounts of glycerol must be synthesized as salinity is raised.

Fig. 3. Artemia franciscana (from Artemia Reference Center, Ghent, Belgium).
Anoxybiosis
Oxygen (O2) is essential for multicellular life on Earth. Anaerobic energy sources can supply the requisite ATP and maintain cellular function for a limited time only, before substrate depletion, energy shortfall or end-product poisoning threaten the survival of the organism. Anoxia tolerance in most vertebrates is short, of the order of minutes, because of the immediate dependence of the heart and central nervous system for a continuous supply of O2. The brain of most vertebrates consumes a high intrinsic rate of oxygen and as a result the brain is generally the first organ to fail. However, remarkably, some species have adapted to survive several months in the absence of O2 and can recover their full functionality at the end of this time when O2 is available again.
Various aquatic species can survive anoxybiosis ranging from a few hours to 5 days. For example, intertidal Echiniscoides specimens have been shown to survive for months in vials of sea water with decaying barnacles (Wright et al. Reference Wright, Westh and Ramløv1992). Brine shrimp (Artemia) cysts have been stored in anoxic conditions for 4 years and then hatched successfully (Wharton Reference Wharton2002). Their food reserves, i.e. trehalose, glycogen and glycerol, were constant during this period, thus indicating a state of anoxybiosis (Wharton Reference Wharton2002). Other reports indicate that embryos of copepods were isolated and viable when retrieved from anoxic marine sediments after 40 years (Marcus et al. Reference Marcus, Lutz, Burnett and Cable1994) and from anoxic freshwater sediments after 332 years (Hairston et al. Reference Hairston, Robert, Brunt, Kearns and Engstrom1995). The resting embryos were assumed to be in the state of anoxybiosis (Clegg Reference Clegg2001). Encysted embryos (cysts) are encased in a hard capsule, or cyst, so that they are protected and can hatch later when conditions are better. Dry conditions cause the encysted embryo to enter a dormant state, which allows it to withstand complete drying, temperatures over 100°C or near absolute zero, high-energy radiation and a variety of organic solvents. The dehydrated cyst can be stored for months or years without loss of hatchability (Treece Reference Treece2000; Snell Reference Snell, Blaise and Ferard2005). While larvae are active, feeding stages in the development of many animals occur after birth or hatching and before the adult form is reached. Larvae and adults are motile and cannot reversibly dehydrate.
The ability of a limited number of ectothermal vertebrates and a substantial number of invertebrates to survive long bouts of continuous anoxia is very impressive. These animals are well-adapted to respond to anoxia by reducing their overall metabolic rates, commonly to between 1 and 10% of aerobic levels (Storey et al. Reference Storey, Baust and Beuscher1981; Hand & Podrabsky Reference Hand and Podrabsky2000). This response, called metabolic rate depression, has been well studied in sessile intertidal invertebrates and involves some interesting modifications in intermediary metabolism. Studies on the encysted Gastrula embryos of the brachiopod crustacean Artemia franciscana revealed no evidence of an ongoing metabolism during bouts of anoxia, which lasted for periods of years (Warner et al. Reference Warner, Jackson and Clegg1997; Clegg Reference Clegg2001). If metabolic activity occurred in Artemia cysts while in anoxybiosis, it was at least 50 000 times slower than the aerobic metabolic rate (Clegg Reference Clegg2001). Long-term anoxybiosis of a nematode species has been reported in which the metabolic rate was reduced to undetectable levels for over 3 months of continuous anoxia (Drinkwater & Crowe Reference Drinkwater and Crowe1991). Fresh-water sponge gemmules subjected to anoxia at room temperatures in water survived almost 4 months of continuous anoxia and most of these were still alive when the study was terminated (Reiswig & Miller Reference Reiswig and Miller1998).
Biochemical changes during anoxybiosis
While some adaptation mechanisms only slow down metabolism, others require a change in biochemistry. Massive concentrations of the protein p26 have been found in the encysted embryo stage in Artemia species (Jackson & Clegg Reference Jackson and Clegg1996; Liang & MacRae Reference Liang and MacRae1999). The protein p26 underwent extensive stress-induced translocation to nuclei and other sites, and these translocations exhibited strong pH dependence (Clegg Reference Clegg2001) in a fashion consistent with intracellular pH changes in vivo (Hand Reference Hand1998; van Breukelen et al. Reference van Breukelen, Maier and Hand2000). Although the participation of p26 as a major biochemical adaptation in anoxybiosis is now established (Clegg Reference Clegg2001), its participation in the embryos was anticipated earlier based on work on the guanine nucleotide pool (Stocco et al. Reference Stocco, Beers and Warner1972), which is unusually large and diverse (Warner Reference Warner and McLennan1992), suggesting that one of these Gp4G molecules (e.g. P1, P4-diguano-sine 5′-teraphosphate) might be utilized during anoxia (Stocco et al. Reference Stocco, Beers and Warner1972). Clegg (Reference Clegg2001) suggested that this nucleotide was indeed metabolized during prolonged anoxia, although extremely slowly. Therefore, it seems that the great majority of intermediary metabolism is indeed brought to a reversible standstill by anoxia, but that a specialized and limited guanine polynucleotide pathway continues to provide free energy for these embryos during anoxia.
Warner and Clegg (Reference Clegg2001) found that p26 is a GTPbinding protein and demonstrated that this protein is a GTPase. Anoxic embryos undergo a massive and almost complete metabolic shutdown, but have to support the energetic requirements of their molecular chaperone, p26, which they achieve by mobilizing Gp4G and eventually producing GTP and/or ATP. Other endergonic processes in anoxic embryos may exist as well.
Anoxic-tolerant vertebrates as example organisms using anoxybiosis
In aquatic environments, continuous access to oxygen cannot be taken for granted. Water with hypoxic and anoxic conditions includes both ice-covered lakes and ponds (Ultsch Reference Ultsch1989) and tropical freshwater systems (Chapman et al. Reference Chapman, Kaufman, Chapman and McKengie1995). Vertebrates living in habitats with these conditions have evolved physiological mechanisms to cope with such challenges (Lutz & Storey Reference Lutz, Storey and Danzler1997). These mechanisms often involve supplemental air breathing and the evolution of organs (including the brain), which are adapted to survive with little or no oxygen (Nilsson & Lutz Reference Nilsson and Lutz2004).
Turtles are either very anoxia tolerant or rather anoxia intolerant during anoxic submergence at low temperatures. A prominent example of a turtle extremely tolerant to anoxic conditions is the freshwater turtle, Chrysemys picta, a widely distributed resident of ponds and streams in the northern United States and southern Canada. This turtle relies on anaerobic glycolysis as an energy source in the anoxic state. Thus, it has to cope with the intrinsic inefficiency of this pathway, and face the dual challenges of depletion of substrate and accumulation of acid metabolites. The turtle spends long periods during the winter in ice-covered ponds without access to the surface, often in water or mud with little or no O2 (Ultsch Reference Ultsch1989). These turtles can survive continuous submergence in nitrogen-equilibrated water at 3°C for more than 4 months, as has been reported through a simulated hibernation study in the laboratory (Ultsch & Jackson Reference Ultsch and Jackson1982; Jackson et al. Reference Jackson, Crocker and Ultsch2000). The western painted turtle (Chrysemys picta bellii) has been shown to survive 4–5 months of anoxic submergence at 3°C during which high lactate levels were accumulated that resulted in significant acid-base disturbances buffered by mobilized carbonates from the skeleton. Skeletal tissue was also used as a depot for sequestering lactate (Ultsch Reference Ultsch1989).
The Crucian Carp Carassius carassius with its habitat in northern Europe is possibly the most extreme example of an anoxia tolerant vertebrate (Fig. 4). This fish inhabits small lakes and ponds of northern Europe, which become anoxic as standing water freezes during winter months and thick ice blocks oxygen diffusion and the light necessary for photosynthesis (Holopainen & Hyvarinen Reference Holopainen and Hyvarinen1985). Anoxic survival capacity in Crucian carp varies greatly with temperature. The Crucian carp can survive anoxia for several months at 0°C, but only a few days at room temperatures (Nilsson & Lutz Reference Nilsson and Lutz2004). Although some turtles such as those described above can survive without oxygen for long periods, they do so by going into ‘suspended animation’. The Crucian carp, however, has evolved in a unique way to remain active during anoxic conditions. When water temperatures are dropping, the carp begins to store vast amounts of glycogen in the brain, which enables it to make the switch to an anaerobic metabolism. Stored glycogen in the body of the carp, mostly in the liver, is then broken down into glucose and ethanol providing the Crucian carp with the energy to survive (Vornanen & Paajanen Reference Vornanen and Paajanen2006). The same study also showed that the sodium–potassium pump of the Crucian carp is responsible for keeping brain cells functioning, but that it reduced its activity ten-fold in lower water temperatures, decreasing the amount of energy needed for continued brain activity in anoxic conditions. With respect to ion channels, the Crucian carp appears to maintain a well-regulated ion-homeostasis during anoxia (in contrast to mammals) with only a negligible rise in extracellular [K+] and intracellular [Ca2+] (Sick et al. Reference Sick, Rosenthal, LaManna and Lutz1982; Nilsson Reference Nilsson2001). With regard to neurotransmitter activity, extracellular GABA (the major inhibitory neurotransmitter in the brain) levels rise in the brain of the Crucian carp (but less than in the turtle), while extracellular glutamate (the major excitatory neurotransmitter) remains low (Hylland & Nilsson Reference Hylland and Nilsson1999).

Fig. 4. Crucian carp (Carassius carassius; photo: J. Bohdal).
Thermobiosis
Thermobiosis is a form of cryptobiosis due to high or low temperatures. If the adaptation to cold temperatures involves a mechanism to prevent the formation of ice crystals in the organism, the term cryobiosis is used. For example, Antarctic notothenioids keep from freezing solid by secreting ‘antifreeze proteins’ that prevent their bodily fluids from turning into ice crystals. The extent to which Antarctic notothenioids have adapted to low, stable temperatures is revealed by their extreme stenothermy (Somero & DeVries Reference Somero and DeVries1967; Weinstein & Somero Reference Weinstein and Somero1998), the presence of antifreeze glycoproteins in their blood (Ruud Reference Ruud1954; DeVries Reference DeVries1988), the loss of haemoglobin (in icefishes, family Hannichthyidae; Cocca et al. (Reference Cocca, Ratnayake-Lecamwasam, Parker, Camardella, Ciaramella, di Prisco and Detrich1995), Ruud (Reference Ruud1954)) and myoglobin (Sidell et al. Reference Sidell, Vayda, Small, Moylan, Londraville, Yuan, Rodnick, Eppley and Costello1997), and the use of a variety of other physiological adaptations (di-Prisco et al. Reference di-Prisco, Condo, Tamburrini and Giardina1991).
In the Southern Ocean, seawater temperatures rarely rise above about −2°C, the freezing point of salt water, but the internal fluids of fish freeze at about −1°C. The water is often filled with tiny ice crystals, which the fish ingest as they eat and which could potentially freeze them from the inside out. Antarctic notothenioid fish survive freezing waters by producing natural antifreeze proteins in the pancreas as recently demonstrated by Cheng et al. (Reference Cheng, Cziko and Evans2006). The evolution of this adaptation was probably driven by the need to prevent the intestinal fluid from freezing, but has since allowed notothenioids to survive.
Adaptation to hot temperatures
Thermophilic (‘heat loving’) organisms have an affinity for temperatures above 40.5°C. Deep-sea hydrothermal vent organisms are often cited as examples of adaptation to extreme environmental conditions (Dalhoff et al. Reference Dalhoff, O'Brien, Somero and Vetter1991; Gaill et al. Reference Gaill, Wiedemann, Mann, Timpl and Engel1991; Desbruye′res et al. Reference Desbruye′res1998). Many thermophiles are microbes from the kingdom Archaea; some, however, belong to the other kingdoms and include multicellular organisms. The multicellular organism most often cited with thermobiosis is the Pompeii worm (Alvinella pompejana). The annelid A. pompejana (Fig. 5) inhabits hot parts of hydrothermal ecosystems with documented occurrences at active vent chimneys from 21°N to 32°S along the East Pacific Rise (Desbruye′res & Laubier Reference Desbruye′res and Laubier1980; Desbruye′res et al. Reference Desbruye′res, Gaill, Laubier and Fouquet1985, Reference Desbruye′res1998). In this environment pressures commonly reach 260 bar and temperatures reach 350°C. In addition, the hydrothermal fluids are acidic, anoxic and rich in metallic sulphides (Gaill Reference Gaill1993). The Pompeii worm is capable of withstanding temperatures as high as 105°C (Chevaldonne et al. Reference Chevaldonne, Desbruye′res and Childress1992), and is known as being the most eurythermal metazoan (Haddad et al. Reference Haddad, Camacho, Durand and Cary1995). Experiments carried out by Brisa et al. (Reference Brisa, Zbindenb and Gaillb2005) demonstrated that A. pompejana influences mineralization processes at the interface between the smoker wall and the ambient oceanic water. The study also indicated that A. pompejana exerts a primary control on its environment by structuring the thermal and chemical gradients, creating a mosaic of micro-environments by the construction of protective tubes. The supply of water through the tube prevents exposure to the extreme temperature spikes and high sulphide concentrations. Circumventing the large erratic changes generally associated with hydrothermal venting, A. pompejana tubes create more homogeneous chemical and thermal micro-niches and likely play a role in microbial diversity.
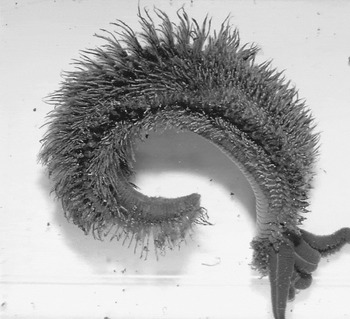
Fig. 5. Dorsal view of a specimen of A. pompejana. Filamentous bacteria are associated with cuticular secretions forming dense aggregates extending from the worm's integument (Haddad et al. Reference Haddad, Camacho, Durand and Cary1995).
Collagens are among the most ubiquitous proteins found in the animal kingdom and can be found in sponges as well as in vertebrates (Scandurra et al. Reference Scandurra, Consalvi, Chiaraluce, Politi and Engel2000). Collagens are extracellular proteins with triple-helical domains (vander-Rest & Garrone Reference vander-Rest and Garrone1991). Among collagens, the most homogeneous subfamily is that of fibrillar collagens (Wharton & Brown Reference Wharton and Brown1991). Fibrillar collagens possess a long central triple-helical domain of 330 to 340 GNN triplets, flanked by an amino-propeptide (N-pro) and a carboxyl-propeptide (C-pro). Collagens are of great importance, not only in performing cohesion between cells, but also in cell differentiation and migration (Wharton & Brown Reference Wharton and Brown1991; vander-Rest & Garrone Reference vander-Rest and Garrone1991). Whereas the interstitial collagen of coastal polychaete worms (e.g. Arenicola marina) is denatured at 28°C, the collagen of A. pompejana remains stable at 45°C and is thus the most thermostable fibrillar collagen known (Gaill et al. Reference Gaill, Mann, Wiedemann, Engel and Timpl1995). Its collagen is adapted to the hydrothermal vent environment by its stability at higher temperatures (Gaill et al. Reference Gaill, Mann, Wiedemann, Engel and Timpl1995) and high pressures (Auerbach et al. Reference Auerbach, Gaill, Jaenicke, Schulthess, Timpl and Engel1995), and by its associated enzymatic processes, which appear to be optimized under anoxic conditions (Kaule et al. Reference Kaule, Timpl, Gaill and Gunzler1998).
The molecular basis of this thermal behaviour includes the increase in proline content and in the number of stabilizing triplets, which correlate with the outstanding thermostability of the interstitial collagen of A. pompejana. Proline residues are of primary importance in performing cohesion between the chains of the triple helix of collagens (vander-Rest & Bruckner Reference vander-Rest and Bruckner1993; Prockop & Kivirikko Reference Prockop and Kivirikko1995). Biochemical analysis has so far been unable to explain Alvinella's collagen thermostability (Gaill et al. Reference Gaill, Mann, Wiedemann, Engel and Timpl1995). A molecular genetic approach has been used by Sicot et al. (Reference Sicot, Mesnage, Masselot, Exposito, Garrone, Deutsch and Gaill2000), who have cloned and sequenced a large cDNA molecule coding the fibrillar collagen of Alvinella, including one-half of the helical domain and the entire C-propeptide domain. For comparison, the group also cloned part of the homologous cDNA from Riftia (Pohlschröder et al. Reference Pohlschröder, Prinz, Hartmann and Beckwith1997). Comparison of the corresponding helical domains of these two species, together with that of the sequenced domain of the coastal lugworm Arenicola marina (Sicot et al. Reference Sicot, Exposito, Masselot, Garrone, Deutsch and Gaill1997), showed that the increase in proline content and in the number of stabilizing triplets correlates with the outstanding thermostability of the interstitial collagen of A. pompejana. Phylogenetic analysis showed that the triple helical and the C-propeptide parts of the same collagen molecule evolve at different rates, in favour of an adaptive mechanism at the molecular level.
Thermobiosis is not limited to hydrothermal vent faunas, but also occurs in terrestrial species. For example, the desert snail Sphicterochila boisseri can survive in the desert at temperatures of up to 50°C (Schmidt-Nielsen Reference Schmidt-Nielsen, Taylor and Shkolnik1971) and the desert ant Proformica longiseta forages on sand surfaces when temperatures reach as high as 60°C (Wehner et al. Reference Wehner, Marsh and Wehner1992).
Adaptation to cold temperatures
The biology of cold tolerance has a long history of scientific study, including pioneering works in the past centuries by Boyle (Reference Boyle1665), Dumeril (Reference Dumeril1849) and Reamur (Reference Reamur1736). The research has diversified into many fields such as cold acclimation, plant and animal cold hardiness, and hibernation studies (Storey et al. Reference Storey, Baust and Beuscher1981; Frank Reference Frank1985; Bowler & Fuller Reference Bowler and Fuller1987; Kamekura et al. Reference Kamekura, Seno, Holmes and Dyall-Smith1992). This type of work is also relevant for cryomedical purposes to preserve mammalian organs (Storey et al. Reference Storey, Baust and Beuscher1981).
A particular research emphasis for low-temperature adaptations is the study of insects. Insects from temperate, polar and high-altitude environments must adapt behaviourally, physiologically and biochemically to survive potentially lethal subzero temperatures (Danks Reference Danks1978; Kukal et al. Reference Kukal, Serianni and Duman1988; Block Reference Block1990) they encounter in their habitat, and a large literature has developed regarding this important component of the life histories of insects (Lee & Denlinger Reference Lee and Denlinger1991; Sømme Reference Sømme1999). Behavioural adaptations also include subzero avoidance by migration (Lee Jr Reference Lee1989) or by seeking sheltered microhabitats (Danks Reference Danks1978, Reference Danks, Lee and Denlinger1991). There are two basic strategies to adapt to cold temperatures. Freeze-tolerant species survive the formation of internal ice, while freeze-avoiding insects die upon freezing, and survive subzero temperatures by keeping their body fluids liquid (Sømme Reference Sømme1999). Despite variations in mechanisms and patterns of cold tolerance within each of these categories (Bale Reference Bale1996; Sinclair & Sjursen Reference Sinclair and Sjursen2001), it is accepted that freeze tolerance and freeze avoidance represent alternative strategies for survival in cold weather (Convey Reference Convey2000). Therefore, insects encounter subzero temperatures by the use of either one of these strategies (Storey et al. Reference Storey, Baust and Beuscher1981; Sinclair & Sjursen Reference Sinclair and Sjursen2001), although several species switch between them (Horwath & Duman Reference Horwath and Duman1984).
Beetle larvae D. canadensis can overwinter as either freeze-avoiding or freeze-tolerant insects; however, most individuals in the population are either one or the other at any given time (Horwath & Duman Reference Horwath and Duman1984; Olsen & Duman Reference Olsen and Duman1997). In the case of freeze avoidance, D. Canadensis maintains a metastable supercooled state. The larval supercooling points decrease from a summer maximum of −2 to −7°C to a winter minimum of −20°C or lower via the removal of highly active hemolymph and gut fluid ice nucleators along with the inhibition of residual nucleators by antifreeze protein (AFP; (Olsen & Duman Reference Olsen and Duman1997)). Dendroides canadensis (Pyrochroidae) overwinter as non-diapausing larvae (Horwath & Duman Reference Horwath and Duman1983) beneath the bark of decaying deciduous trees. Their hemolymph contains polyols and AFPs that increase seasonally in activity and concentration (Kukal et al. Reference Kukal, Serianni and Duman1988; Olsen & Duman Reference Olsen and Duman1997) cued by seasonal photo- and thermoperiodic cycles (Horwath & Duman Reference Horwath and Duman1983). Their hemolymph thermal hysteresis activity increases from 0.5°C in summer to 5°C or greater during midwinter (Kukal et al. Reference Kukal, Serianni and Duman1988; Olsen & Duman Reference Olsen and Duman1997). Immunofluorescent staining of transverse sections of cold-hardy D. canadensis larvae indicated that AFPs are found in the subcuticular epidermal layer (Kukal et al. Reference Kukal, Serianni and Duman1988); however, the role of these epidermal AFPs in inoculative freezing resistance is unknown. Olsen & Duman (Reference Olsen and Duman1997) found that seasonal modifications in epidermal and hemolymph AFPs in D. canadensis contributed most strongly to the inhibition of inoculative freezing. Winter integument patches (cuticle with epidermis) were more resistant to inoculative freezing than were summer integument patches. The results suggested that some integumentary ice nucleators were removed in cold-hardy larvae and that AFP promoted supercooling by inhibiting the activity of these nucleators.
Cryobiosis (as a special case of thermobiosis)
Over vast areas of the globe, temperatures drop during the winter season far below the freezing point of water. The formation of ice crystals within cells is uniformly damaging throughout the animal and plant kingdom, and organisms that inhabit seasonally cold areas of the Earth must have well-developed mechanisms for enduring or avoiding long-term exposure to temperatures below freezing (Storey et al. Reference Storey, Baust and Beuscher1981). Cryobiosis takes place in reaction to decreased temperature when freezing occurs. In cryobiosis, the organism freezes all of the water within its cells and becomes dormant until more hospitable conditions reappear. It has been indicated through various studies that the longer an organism remains in cryobiosis, the longer it takes for the organism to come out of cryobiosis (Keilin Reference Keilin1959). The organism has to use its own energy sources to come out of cryobiosis and the longer the organism remains in cryobiosis the less energy it has at its disposal. The common organisms of choice to study cryobiosis are tardigrades, which are known to survive freezing to near absolute zero. Cryobiosis, anhydrobiosis and osmobiosis all involve restructuring and/or removal of water from cells, and therefore their metabolic depressant effect may share a common mechanism (Keilin Reference Keilin1959).
Tardigrades as an example of thermobiosis and cryobiosis
Tardigrades are metameric invertebrates and are found worldwide in various habitats such as marine, freshwater and terrestrial ecosystems. The species inhabiting terrestrial habitats are active only when surrounded by at least a film of water. When deprived of their free water, they survive by entering the cryobiotic state. This capability allows tardigrades to colonize extreme environments such as deserts, high mountains and polar regions (Bertolani et al. Reference Bertolani, Guidetti, Jonsson, Altiero, Boschiini and Rebecchi2004). Together with nematodes and bdelloid rotifers, tardigrades are known to enter cryptobiosis at any stage of their life cycle, from egg to adult (Bertolani et al. Reference Bertolani, Guidetti, Jonsson, Altiero, Boschiini and Rebecchi2004). Tardigrades overcome adverse conditions also by forming cysts, for example, by reducing their metabolism even less than in the cryptobiotic state (Pigòn & Węglarska Reference Pigòn and Węglarska1953), without losing large quantities of water. This capability characterizes several limnic tardigrades, but also some soil and moss-dwelling tardigrades.
Tardigrades resemble closely Onychophora, Euarthropoda and Annelida. However, tardigrades are animals that have small body sizes. The traditional concept is that tardigrades had large body sizes in the ancestor of this common taxon (Articulata; Schmidt-Rhaesa (Reference Schmidt-Rhaesa2001)). If so, the small size and the absence of organs such as a dorsal heart, segmental coelomic cavities and metanephridia must be interpreted as specialized adaptations to their environment (Fig. 6; Schmidt-Rhaesa (Reference Schmidt-Rhaesa2001)). When exposed to cryptobiotic stresses, tardigrades contract into a so-called tun, and the permeability of the cuticle declines (Wright Reference Wright1989; Drinkwater & Crowe Reference Drinkwater and Crowe1991). This reduces the rate of transpiration and gives the animal time for synthesis of tissue protectants such as trehalose (Drinkwater & Crowe Reference Drinkwater and Crowe1991; Westh & Ramlùv Reference Westh and Ramlùv1991). These protectants replace the bound water in membranes and allow structural cell integrity to be maintained in completely dry or frozen organisms (Drinkwater & Crowe Reference Drinkwater and Crowe1991; Clegg Reference Clegg2001).

Fig. 6. Image of a tardigrade using a scanning electron microscope (photo: H. Greven).
In order to investigate cryobiotic effects, Bertolani et al. (Reference Bertolani, Guidetti, Jonsson, Altiero, Boschiini and Rebecchi2004) conducted a laboratory study with four species of eutardigrades belonging to two families and living on different substrates. Two species belonged to the family Hypsibiidae, Ramazzottius oberhaeuseri (lichen-dwelling) and Hypsibius dujardini (fresh water-dwelling); the other two species belonged to the family Macrobiotidae, Dactylobiotus parthenogeneticus (fresh water-dwelling) and Macrobiotus richtersi (leaf litter-dwelling). Active animals were frozen at different temperatures (−9, −20 and −80°C), kept frozen for 6 days, placed at −9°C overnight and then gradually thawed to 12°C. Survival was evaluated 24 hours after the beginning of thawing. The four species considered showed very different patterns of cryobiotic survival with the terrestrial species R. oberhaeuseri and M. richtersi showing the highest survival rates. Interestingly, the survival rates of R. oberhaeuseri showed very little dependence on the variation of the experimental temperatures.
Many other organisms exist that use cryobiotic adaptation mechanisms (Table 2). These adaptation mechanisms allow these organisms to persist in environments that most other species are excluded from.
Table 2. Some example organisms using cryobiosis as a survival mechanism

Hibernation
Hibernation is not a cryptobiotic state, because metabolism is maintained through the process but, owing to its importance to more complex organisms in particular, it will be discussed here. Hibernation is a state of inactivity in an animal lasting several days or weeks that allows animals to conserve energy during the long cold winter season when food is scarce or just difficult to find. The ability to hibernate is the most dramatic example of phenotypic plasticity displayed by mammals. Each year mammalian hibernators undergo a complex suite of morphological, physiological and behavioural changes in response to seasonal periods of high-energy demand coupled with reduced energy availability in the environment. The adaptive value of the hibernating phenotype is realized by eliminating the need to maintain a constant, high body temperature by entering torpor.
During hibernation, the body temperatures of mammalian hibernators drop to below 10°C or even 5°C, and the metabolic rate is reduced to only a few percent of the euthermic level. Heart rate and breathing rate also fall. However, unlike the lower vertebrate and invertebrate hibernators, mammalian hibernators maintain the thermoregulatory control with a lowered set point during hibernation and retain the capacity to arouse from hypothermia by physiological means. Mammalian hibernation accompanies various physiological changes (Wang Reference Wang1989). Several histological and morphological studies reveal that polyglandular involution takes place prior to the onset of hibernation and that endocrine functions are generally depressed during the first half of the hibernation season (Wang Reference Wang, Lyman, Willis, Malan and Wang1982). Also, the lymphoid organs undergo an involution during Fall, and the immune system is depressed during hibernation (Shivatcheva Reference Shivatcheva, Malan and Canguilhem1989). A number of molecular and cellular changes that occur during the preparation, entrance and torpor stages of hibernation have been defined (MacDonald & Storey Reference MacDonald and Storey1999; O'Hara et al. Reference O'Hara, Watson, Srere, Kumar, Wiler, Welch, Bitting, Heller and Kilduff1999). The mRNA translation process is conventionally divided into three distinct phases termed (peptide-chain) initiation, elongation and termination (Proud Reference Proud1992). The global rates of protein synthesis have been determined to be profoundly reduced in hibernating animals. The decreased rate of protein synthesis is due to inhibition of both initiation and elongation (Frerichs et al. Reference Frerichs, Smith, Brenner, DeGracia, Krause, Marrone, Dever and Hallenbeck1998).
The mechanism that contributes to the regulated suppression of metabolism and thermogenesis during hibernation is reversible phosphorylation of enzymes and proteins that limits rates of flux through metabolic pathways (Storey et al. Reference Storey, Baust and Beuscher1981). The acute regulation of mRNA translation depends on changes in the activity of initiation factors or elongation factors, which is also commonly mediated by alteration in their states of phosphorylation (Hovland et al. Reference Hovland, Eikhom and Proud1999). A substantial decrease in the rate of protein synthesis initiation in hibernators is contributed by phosphorylation of eIF2α (Frerichs et al. Reference Frerichs, Smith, Brenner, DeGracia, Krause, Marrone, Dever and Hallenbeck1998); however, the mechanism(s) involved in the regulation of elongation in hibernators is not known (Chen et al. Reference Chen, Matsushita, Nairn, Damuni, Cai, Frerichs and Hallenbeck2001). mRNA translocation on the ribosome involves elongation factor-2 (eEF-2). eEF-2 is phosphorylated and inhibited by a specific kinase (eEF-2 kinase), with phosphorylation reducing the ability of the factor to promote translocation, possibly by decreasing its affinity for pretranslocation ribosomes (Nairn & Palfrey Reference Nairn and Palfrey1987; Ryazanov & Shestakova Reference Ryazanov and Shestakova1988; Redpath & Proud Reference Redpath and Proud1989; Carlberg et al. Reference Carlberg, Nilsson and Nygaard1990). Increased levels of phosphorylation of eEF-2 occur in response to cellular stimulation by mitogens, growth factors and neurotransmitters that influence intracellular Ca2+ levels (Redpath et al. Reference Redpath, Foulstone and Proud1996; Ryazanov & Shestakova Reference Ryazanov and Shestakova1988). Results provided by Chen et al. (Reference Chen, Matsushita, Nairn, Damuni, Cai, Frerichs and Hallenbeck2001) suggest that the level of eEF-2 phosphorylation is increased in hibernators through both an increase in eEF-2 kinase activity and a decrease in PP2A activity. However, the precise physiological role of eEF-2 phosphorylation has not yet been elucidated.
Changes in gene expression
Hibernation's unique characteristics as a phenotype probably constrain the types of molecular changes that are employed. Seasonal changes prepare the animal for hibernation; one example is the storage of enormous reserves of energy in the form of white adipose tissue (WAT) in late summer and early autumn. These seasonal changes may be regulated less by changing the amounts of gene product via altered transcription, translation or stability, and more by employing rapidly reversible molecular switches such as phosphorylation to control protein activity (Storey et al. Reference Storey, Baust and Beuscher1981). Because the hibernating mammal arouses periodically from torpor throughout the hibernation season, the ability to function at euthermic temperatures must be maintained continuously. At the molecular level, this indicates that the steady-state levels of mRNA and protein expressed from the majority of genes will remain relatively constant between the active and hibernating seasons. A relatively small number of genes that perform crucial functions for hibernation will be up-regulated during winter, whereas other gene products that are not essential during hibernation may be down-regulated. Several mRNAs are differentially expressed during hibernation. An example of a seasonally up-regulated gene at the mRNA level in hibernators is -2-macroglobulin, which encodes a broad-spectrum protease inhibitor that is produced in the liver and then secreted into the plasma (O'Hara et al. Reference O'Hara, Watson, Srere, Kumar, Wiler, Welch, Bitting, Heller and Kilduff1999). Both its mRNA and protein were shown to increase during winter in ground squirrels. This protein plays a pivotal role in controlling blood clotting. It is well known that clotting times of blood taken from hibernators are significantly increased (O'Hara et al. Reference O'Hara, Watson, Srere, Kumar, Wiler, Welch, Bitting, Heller and Kilduff1999). Because improving microcirculation in hypothermic rats (Lee et al. Reference Lee, Jordan, Wang, Geiser, Hurlbert and Nicol1996) and preventing or reversing blood clotting in cardiac arrest patients (Bottiger & Martin Reference Bottiger and Martin2001) enhances survival, reduced clotting is likely to be a significant adaptation for hibernation. In the liver, the genes encoding thyroxine-binding globulin, apolipoprotein A1 and cathepsin H are also up-regulated at the mRNA level during the hibernation season (Epperson & Martin Reference Epperson and Martin2002). The mRNAs for the immediate-early genes, c-fos, cjun and junB are differentially expressed in the brain of ground squirrels across the hibernation cycle (O'Hara et al. Reference O'Hara, Watson, Srere, Kumar, Wiler, Welch, Bitting, Heller and Kilduff1999). This probably reflects the established link between neuronal activation and immediate-early gene expression (Christman et al. Reference Christman, Morgan, Jacobson and Ames1985). The switch from carbohydrate to fatty acid metabolism is regulated by differential gene expression at multiple levels, including altering the steady-state levels of key proteins and the mRNAs encoding them. The general pattern is to suppress carbohydrate metabolism and increase fatty acid metabolism. Elevated levels of pyruvate dehydrogenase kinase isoenzyme 4 (PDK4) mRNA and protein in hibernating ground squirrels suppress glycolytic activity (Christman et al. Reference Christman, Morgan, Jacobson and Ames1985; Buck et al. Reference Buck, Squire and Andrews2002). Pancreatic triacylglycerol lipase (PTL), which hydrolyses ester linkages in dietary triacylglycerols and is normally expressed exclusively in the pancreas, is also expressed at high levels in the heart of hibernating ground squirrels, at both the mRNA and protein levels (Andrews et al. Reference Andrews, Squire, Bowen and Rollins1998). Glyceraldehyde-3-phosphate dehydrogenase is down-regulated at the mRNA, protein and activity levels in skeletal muscle of hibernating jerboas (Soukri et al. Reference Soukri, Valverde, Hafid, Elkebbaj and Serrano1996), as is acetyl CoA carboxylase in the hearts of hibernating ground squirrels (Belke et al. Reference Belke, Wang and Lopaschuk1998). Other reports of up-regulated mRNAs in hibernating ground squirrels include: uncoupling protein 2 (UCP2) in WAT and UCP3 in skeletal muscle (Boyer et al. Reference Boyer, Barnes, Lowell and Grujic1998); heart-type fatty acid binding protein (FABP) in brown adipose tissue (BAT), skeletal muscle and heart (Hittel & Storey Reference Hittel and Storey2001); adipose type FABP in BAT and heart; cytochrome-c oxidase subunit 1 and ATP synthase 6/8 in kidney, BAT and heart; NADH-ubiquinone oxidoreductase subunit 2 in heart, skeletal muscle and liver; and ventricular myosin light chain 1 in heart and skeletal muscle (Fahlman et al. Reference Fahlman, Storey and Storey2000). Down-regulated mRNAs during hibernation have also been reported, including: those for prostaglandin D2 synthase in the brain of ground squirrels (O'Hara et al. Reference O'Hara, Watson, Srere, Kumar, Wiler, Welch, Bitting, Heller and Kilduff1999); glyceraldehyde-3-phosphate dehydrogenase in the muscle of jerboas (Soukri et al. Reference Soukri, Valverde, Hafid, Elkebbaj and Serrano1996); and liver-expressed HP-22, -25, -27 and -55, which form a protein complex in chipmunk plasma and which are also decreased in hibernation (Takamatsu et al. Reference Takamatsu, Ohba, Kondo, Kondo and Shiba1993, Reference Takamatsu1997). Recent work on the plasma proteins HP-25 (Kojima et al. Reference Kojima, Takamatsu, Ishii, Kondo and Shiba2000, Reference Kojima, Shiba, Kondo and Takamatsu2001), HP-20 (Ono et al. Reference Ono, Hosoe, Azuma, Shoji, Nara, Kondo, Shiba and Takamatsu2001) and HP-27 (Ono et al. Reference Ono, Kojima-Kawagoe, Kondo, Shiba and Takamatsu2003) has focused on the liver-specific regulation of their expression at the transcriptional level.
At the protein level, myoglobin appears to increase in skeletal muscle as animals prepare for and undergo hibernation. This increase may facilitate shivering thermogenesis during torpor and arousal (Postnikova et al. Reference Postnikova, Tselikova, Kolaeva and Solomonov1999). Immunoreactivity for the gap junction protein connexin43 is elevated in cardiac myocytes of hibernating hamsters and reverses to euthermic control values within 2 hours of arousal to euthermy (Saitongdee et al. Reference Saitongdee, Milner, Becker, Knigh and Burnstock2000). It was suggested that an increase in gap junctions might prevent ventricular fibrillation at the low body temperature values of torpor. However, because connexin43 immunoreactivity is elevated in cold-acclimated control hamsters that did not hibernate (Saitongdee et al. Reference Saitongdee, Milner, Becker, Knigh and Burnstock2000), this change is not strictly associated with the hibernating phenotype.
Histamine (HA) is a multifunctional messenger that acts as a neurotransmitter or neuromodulator in the brain (Schwartz et al. Reference Schwartz, Arrang, Garbarg, Pollard and Ruat1991; Wada et al. Reference Wada, Inagaki, Yamatodani and Watanabe1991). In mammals, histaminergic perikarya are located in the tuberomammillary nucleus of the posterior hypothalamus, projecting fibres to almost all parts of the brain (Airaksinen & Panula Reference Airaksinen and Panula1988; Inagaki et al. Reference Inagaki, Yamatodani, Ando-Yamamoto, Tohyama, Watanabe and Wada1988; Panula et al. Reference Panula, Pirvola, Auvinen and Airaksinen1989). HA has been implicated in the regulation of the sleep–wake cycle, body temperature, energy metabolism, reproductive behaviour and neuroendocrine secretion (Schwartz et al. Reference Schwartz, Arrang, Garbarg, Pollard and Ruat1991). Hibernation is a physiological state and is under strict neuronal control involving the hypothalamus, septum, hippocampus and brainstem reticular formation (Beckman & Stanton Reference Beckman, Stanton, Beckman and Stanton1982; O'Hara et al. Reference O'Hara, Watson, Srere, Kumar, Wiler, Welch, Bitting, Heller and Kilduff1999).
The fact that at least one species of mammalian hibernator, the arctic ground squirrel, maintains subzero body temperature values during deep torpor raises the possibility that specialized antifreeze proteins similar to those found in Antarctic fishes might be expressed during hibernation that would prevent freezing of body fluids at body temperatures below 0°C (Fig. 7). To date, however there is no evidence for antifreeze activity such as thermal hysteresis in blood from supercooled arctic ground squirrels (Barnes Reference Barnes1989). Other mammals that are specially adapted to very cold temperatures are the Arctic fox, which can sleep on open snow at temperatures as low as −80°C for up to 1 hour without decreasing its body temperature (Wharton Reference Wharton2002), and the Antarctic springtail (Gomphiocephalus hodgsoni), which can live at temperatures below −39°C in the Antarctic environment (Sinclair & Sjursen Reference Sinclair and Sjursen2001).

Fig. 7. Arctic Ground Squirrel (Spermophilus parryii), an ideal object to study hibernation in mammals. During hibernation ground squirrels typically spend 80–90% of the hibernation season at body temperatures below 5°C (Wang Reference Wang, Wang and Hudson1978), although due to periodical interruption body temperatures reach above 35°C. The metabolic rate of hibernating mammals is less than 6% of corresponding normal thermic values, which saves energy considerably (Geiser & Ruf Reference Geiser and Ruf1995; Song et al. Reference Song, Kortner and Geiser1997; Kortner & Geiser Reference Kortner and Geiser2000) (image from http://www.nhptv.org/natureworks/arcticgroundsquirrel.htm with permission of Nature Works, New Hampshire Public Television).
Diapause
Diapause is a common feature of the life cycle of many animal species (Lees Reference Lees1955; Yamashita & Hasegawa Reference Yamashita, Hasegawa, Kerkut and Gilbert1985; Marcus Reference Marcus1996). The significance of diapause includes the acquisition of mechanisms that led to resistance to environmental stress or as a timing device to synchronize favourable environmental states with an appropriate stage of development or both (Sandell Reference Sandell1990; Mcnamara Reference Mcnamara1994). Diapause is a dynamic state of low metabolic activity that is neurohormonally mediated. Reduced morphogenesis, increased resistance to environmental extremes and altered or reduced behavioural activity is associated with diapause. It occurs during a genetically determined stage of metamorphosis, and its full expression develops in a species-specific manner, usually in response to a number of environmental stimuli that precede unfavourable conditions. Upon beginning of diapause, metabolic activity is suppressed even if conditions favourable for development prevail.
Discussion
Most scientists associate micro-organisms with the word extremophile, particularly Archaea. However, there are many multicellular and macroscopic organisms that are well adapted to extreme environmental conditions. Here we have reviewed some of the processes used by animals and plants to adapt to extreme environmental stresses such as temperature extremes, lack of oxygen and water, and high salt conditions. We barely understand the responses to these processes, the associated biochemical changes, genetic switches and what leads to the induction of the cryptobiotic state or hibernation. Perhaps the largest strides have been made in the research about hibernation. Most of the hibernation research has been geared towards mammals, which shows our own bias as humans towards our own heritage and also the economic potential for biomedicine for the freezing and preservation of organs and organ tissue. Even here, however, we only have an incomplete picture about what induces hibernation and the associated biochemical changes and genetic switches to maintain it during torpor.
This research has great significance for astrobiology as it does put in question the common assumption that increased complexity has necessarily to result in an increased sensitivity to environmental changes and extremes. As some of the multicellular extremophiles discussed here clearly underline, this does not necessarily have to be the case. Although microbes are the ultimate masters of adaptation owing to techniques such as gene swapping, transposition, transformation, etc, increases in complexity may in certain cases actually provide increased opportunity for adaptation. These opportunities have been utilized in a broad spectrum of organisms ranging from plants to animals and from rather simplistic organisms such as worms to highly complex organisms such as mammals. While the exploration of life is probably limited to microbial forms on our neighbouring planets and moons, understanding the adaptation mechanisms would enhance our ability to make planets such as Mars suitable for the habitation of terrestrial life forms (if we ultimately decide to pursue terra-forming concepts). The multicellular extremophiles on Earth also represent the best analogue for the study of possible forms and adaptation mechanisms employed by multicellular extraterrestrial organisms. This may even be relevant for intelligent life as many of the larger mammals (e.g. the omnivorous northern bears such as the brown bear, black bear and Grizzly bear) have the capability to hibernate during the cold winter periods and show incredible adaptation capabilities to their environment. In essence, even the human species Homo sapiens has adapted quite well to an extreme range of environments, which includes the temporary habitability of space. Homo sapiens achieved that feat by using a strategy not employed by any other species on our planet: manual dexterity and intelligence culminating in technology.
Conclusions
Adaptation to extreme environmental stresses not only occurs in microbes, but it also occurs in many multicellular and macroscopic organisms. Increases in complexity within an organism do not necessarily imply an increased sensitivity to environmental stresses, but, in fact, can lead under certain circumstances to new opportunities and adaptation strategies. Examples are organisms such as certain species of fish that can survive without oxygen for months without giving up brain activity and resurrection plants that can survive near-complete desiccation. These organisms are clearly relevant as an analogue for possible complex extraterrestrial life as well as for terraforming planets or moons in our solar neighbourhood.
Acknowledgement
We appreciate the constructive comments by Jim Clegg of the University of California-Davis, which significantly improved the quality of this paper.