1. Introduction
The basement of Iran evolved during the late Neoproterozoic – early Cambrian period (610–520 Ma; e.g. Ramezani & Tucker, Reference Ramezani and Tucker2003; Hassanzadeh et al. Reference Hassanzadeh, Stockli, Horton, Axen, Stockli, Grove, Schmitt and Walker2008; Rossetti et al. Reference Rossetti, Nozaem, Lucci, Vignaroli, Gerdes, Nasrabadi and Theye2015; Shabanian et al. Reference Shabanian, Davoudian, Dong and Liu2018; Daneshvar et al. Reference Daneshvar, Maanijou, Azizi and Asahara2019). This basement resulted from magmatism reflecting subduction of Proto-Tethys oceanic lithosphere beneath northern Gondwana (Ramezani & Tucker, Reference Ramezani and Tucker2003; Hassanzadeh et al. Reference Hassanzadeh, Stockli, Horton, Axen, Stockli, Grove, Schmitt and Walker2008). It was also fragmented and drifted from Gondwana as the Palaeo-Tethys and Neo-Tethys oceans opened and later reclosed during the Cambrian–Tertiary period (e.g. Berberian & Berberian, Reference Berberian, Berberian, Gupta and Delany1981; Şengör, Reference Şengör, Coward, Dewey and Hancock1987). The closure of the Palaeo-Tethys was completed by the Late Triassic Epoch (e.g. Horton et al. Reference Horton, Hassanzadeh, Stockli, Axen, Gillis, Guest, Amini, Fakllari, Zamanzadeh and Grove2008), and the opening of the Neo-Tethys was underway by early Permian time (e.g. Alirezaei & Hassanzadeh, Reference Alirezaei and Hassanzadeh2012).
The Zagros orogen, which resulted from the opening and closure of the Neo-Tethys Ocean, is composed of three NW–SE elongated parallel tectonic zones (Alavi, Reference Alavi1994) that from the Arabian Plate to Central Iran are: (1) the Zagros Simply Folded Belt; (2) the Sanandaj–Sirjan Zone; and (3) the Urumieh–Dokhtar magmatic arc (UDMA) (Fig. 1a). It is attributed to subduction of the western portion of Neo-Tethys oceanic crust and subsequent collision of the Arabian Plate with the eastern Iranian microplate (e.g. Alavi, Reference Alavi1994). The estimates for the time of the Arabia–Eurasia plate collision have been highly controversial, ranging from the Late Cretaceous to the Pliocene epochs. The irregular shape of the Arabian indenter suggests that collision was probably diachronous along the belt (e.g. Agard et al. Reference Agard, Omrani, Jolivet and Mouthereau2005; Ballato et al. Reference Ballato, Uba, Landgraf, Strecker, Sudo, Stockli, Friedrich and Tabatabaei2011), which accounts for some of the inconsistency in estimates for collision. Chiu et al. (Reference Chiu, Chung, Zarrinkoub, Mohammadi, Khatib and Iizuka2013) suggested that the oblique collision between Arabia and Eurasia started no later than c. 30 Ma (early Oligocene). The collision then propagated SE-wards in the southeastern part by c. 10 Ma (late Miocene), when the UDMA magmatism started changing geochemical composition from calc-alkaline to adakitic. Some research (e.g. Ao et al. Reference Ao, Xiao, Khalatbari Jafari, Talebian, Chen, Wan, Ji and Zhang2016) suggested that final closure of the Neo-Tethys Ocean occurred during the late Miocene Age, whereas others (e.g. Alavi, Reference Alavi1994; Mohajjel et al. Reference Mohajjel, Fergusson and Sahandi2003) suggested that the Urumieh Dokhtar magmatic system is still active and is associated with ongoing subduction of Indian oceanic crust (e.g. Agard et al. Reference Agard, Omrani, Jolivet, Whitechurch, Vrielynck, Spakman, Monié, Meyer and Wortel2011).

Fig. 1. (a) Simplified geological map of Iran and (b, c) distribution of igneous rocks of Urumieh Dokhtar magmatic belt (UDMA) within it. Location of intrusive rocks and details of age are (1) Haji Abad (40 Ma, Kazemi et al. Reference Kazemi, Kananian, Xiao and Sarjoughian2019); (2) Gheshlagh–Aftabrow (40 Ma, Kazemi et al. Reference Kazemi, Kananian, Yilin and Sarjoughian2020), (3) Saveh (40–37 Ma, Nouri et al. Reference Nouri, Azizi, Stern, Asahara, Khodaparast, Madanipour and Yamamoto2018); (4) Khalkhab–Neshveh (38 Ma, Rezaei-Kahkhaei et al. Reference Rezaei-Kahkhaei, Galindo, Pankhurst and Esmaeily2011); (5) Soheyle–Pakuh (39.6 Ma, Sarjoughian et al. Reference Sarjoughian, Javadi, Azizi, Ling, Asahara and Lentz2020); (6) Golshekanan (37.6 Ma, this study); (7) Zafarghand (24.6 Ma, Sarjoughian & Kananian Reference Sarjoughian and Kananian2017; Sarjoughian et al. Reference Sarjoughian, Lentz, Kananian, Ao and Xiao2018); (8) Marshenan (20.5 Ma, Sarjoughian & Kananian Reference Sarjoughian and Kananian2017; Sarjoughian et al. Reference Sarjoughian, Azizi, Lentz and Ling2019); (9) Sarduiyeh (27.95 Ma, Nazarinia et al. Reference Nazarinia, Mortazavia, Arvinb, Hu, Zhao and Poosti2020); (10) Rabor–Lalehzar (23 Ma, Chekani Moghadam et al. Reference Chekani Moghadam, Tahmasbi, Ahmadi-Khalaji and Santos2018); and (11) Kuh–Panj (16.9–12.2 Ma, Asadi, Reference Asadi2018).
The UDMA is located along the northeastern margin of the Zagros orogenic belt, between the Sanandaj–Sirjan zone and Central Iran zone, representing a magmatic belt, which extends along the Sanandaj–Sirjan zone and Central Iranian microcontinent (Alavi, Reference Alavi1994). The UDMA is composed of voluminous mafic to felsic volcanic successions with minor intrusive rocks. The volcanic successions largely comprise calc-alkaline rocks occurring as basaltic and andesitic lava flows, pyroclastic tuffs and ignimbrites (e.g. Stöcklin, Reference Stöcklin1968; Berberian & Berberian, Reference Berberian, Berberian, Gupta and Delany1981). The arc volcanism across the UDMA has been argued to be dominated by an Eocene pulse, although Chiu et al. (Reference Chiu, Chung, Zarrinkoub, Mohammadi, Khatib and Iizuka2013, Reference Chiu, Chung, Zarrinkoub, Melkonyan, Pang, Lee, Wang, Mohammadi and Khatib2017) reported abundant ages that indicate long-lasting magmatic activity, from the Eocene to the Oligocene epochs. The plutonic rocks include gabbroic, dioritic, granodioritic and granitic intrusions of different sizes, ranging in age from Eocene to Miocene (e.g. Chiu et al. Reference Chiu, Chung, Zarrinkoub, Mohammadi, Khatib and Iizuka2013; Sarjoughian & Kananian, Reference Sarjoughian and Kananian2017). Geochemical studies indicate that the UDMA is composed of subduction-related calc-alkaline and, to a limited extent, tholeiitic rocks (e.g. Ahmad & Posht Kuhi, Reference Ahmad and Posht Kuhi1993), but also with slight alkaline and shoshonitic rocks in the terminal stages (e.g. Ahmadzadeh et al. Reference Ahmadzadeh, Jahangiri, Lentz and Mojtahedi2010; Sarjoughian et al. Reference Sarjoughian, Kananian, Haschke and Ahmadian2012).
The Golshekanan pluton is located in the central part of the UDMA, and was studied in order to build a better understanding of the magmatic and associated geodynamic evolution of the UDMA. Granitoids provide key clues to understanding Cenozoic magmatism in the UDMA and the growth of continental crust. The UDMA has been the focus of exploration as a result of the high potential of locating porphyry Cu resources. Considering the dual importance of UDMA from geodynamic and metallogenic perspectives, comprehensive petrogenetic studies are fundamental. Although the subduction tectonic context of the UDMA is well constrained, the processes of magma production and source compositions remain controversial. Some researchers (e.g. Asadi, Reference Asadi2018; Deng et al. Reference Deng, Wan, Dong, Talebian, Windley, Dadashzadeh, Mohammadi and Barati2018; Wan et al. Reference Wan, Deng, Najafi, Hezareh, Talebian, Dong, Chen and Xiao2018) suggested that the intrusive rocks in the central UDMA were generated from partial melting of juvenile mafic lower crust. However, Honarmand et al. (Reference Honarmand, Rashidnejad Omran, Neubauer, Hashem Emami, Nabatian, Liu, Dong, Quadt and Chen2014), Babazadeh et al. (Reference Babazadeh, Ghorbani, Bröcker, D’Antonio, Cottle, Gebbing, Mazzeo and Ahmadi2017, Reference Babazadeh, Ghorbani, Cottle and Bröcker2019) and Nouri et al. (Reference Nouri, Azizi, Stern, Asahara, Khodaparast, Madanipour and Yamamoto2018) concluded that some magmas were formed from partial melting of asthenospheric mantle and (or) subcontinental lithospheric mantle with or without lower crustal contributions. Detailed studies of individual igneous rocks could therefore be a more appropriate approach to evaluate the origin of the magma.
The Golshekanan pluton belongs to the calc-alkaline series that is similar to an active continental margin setting, and suggests a crustal origin for these rocks (H. Aliashrafzadeh, unpub. MSc thesis, University of Tehran, 2013). Based on the U–Pb method, Sarjoughian & Kananian (Reference Sarjoughian and Kananian2017) show an age of 36.8 ± 0.5 Ma for the Mehrabad intrusion. Although this pluton is not well known, it is studied here in detail for the first time. This research reports new petrographic and geochemical data and U–Pb zircon ages together with Rb–Sr and Sm–Nd data for the Golshekanan granitoid rocks of northern Nain. These data provide insights into: (1) the geochemical characteristics of the source involved in the petrogenesis of UDMA igneous rocks; (2) a petrogenetic model for its generation and emplacement in the framework of the development of the UDMA; and (3) the geodynamic evolution and nature of Cenozoic magmatism across the region.
2. Geological overview
The Golshekanan area is exposed in the central UDMA, in the western part of the Central Iran zone (Fig. 1a) and in the NE of the Esfahan Province, at latitudes 33° 12′–33° 13′ N and longitudes 52° 54′–52° 56′ E (Figs 1b, 2). The basement rocks in this area consist of an ophiolitic mélange complex that is composed of serpentinite, peridotite and minor dunite and pyroxenite, along with radiolarite, limestones and chert. Tertiary magmatic activity can be divided into two main phases: Eocene volcanism with andesite, dacite and rhyolite compositions, including pyroclastic rocks (mainly tuff); followed by emplacement of intermediate to felsic intrusive bodies (Davoudzadeh, Reference Davoudzadeh1972; Mansouri Esfahani et al. Reference Mansouri Esfahani, Khalili and Bakhshi2017).

Fig. 2. Regional geological map of the Golshekanan granitoid based on the geological map of Shahrab 1:100 000 (modified after Bahroudi, Reference Bahroudi1999), with slight modifications.
The Golshekanan granitoid is exposed as small intrusive bodies in the Mehrabad area, near the western part of the village of Golshekanan. Field observations reveal the studied rocks are mostly medium-grained granite and granodiorite with a minor proportion of monzonite and diorite (Fig. 3a). Porphyritic granodiorite and granite with large feldspar and amphibole phenocrysts also occur. Because of the poor outcrop exposure, the intrusive field relationships cannot be observed. It seems that contacts between granite and granodiorite rocks and porphyritic granodiorite and granite are transitional, and the intrusive bodies may have been rapidly cooled (thermal quenching) at their margin; however, on previous maps a large part of the intrusive rocks was mapped as plutonic rocks (Bahroudi, Reference Bahroudi1999).

Fig. 3. Field photographs of (a) the Golshekanan granitoid and (b) xenolith enclaves in the host granitoids, and petrographic photographs of (c) porphyritic granodiorite and (d) granite rocks. Mineral abbreviations are from Kretz (Reference Kretz1983).
This body is characterized by a smooth rounded morphology and is typically light grey to pink in colour. The pluton hosts a few small xenolith enclaves of the host rocks (Fig. 3b). Additionally, a few mafic doleritic dykes with basalt, andesite and trachyandesite composition cross-cut the Goshekanan granitoid and its peripheral rocks. These dykes are variable in thickness, from a few centimetres to a metre in width.
3. Petrographic features
The studied rocks are mostly granite and granodiorite plus lesser monzonite and diorite varieties. They are composed mainly of quartz, K-feldspar, plagioclase and amphibole, and accessory biotite, zircon, apatite and titanite. The granodioritic rocks contain more plagioclase than the granitic rocks, the monzonite contains more amphibole and less quartz, and diorite has more amphibole and plagioclase, with less quartz and K-feldspar compared with the granites and granodiorites.
These rocks are generally medium-grained and shown granular texture and subordinate granophyre. Plagioclase forms euhedral to subhedral tabular crystals and commonly displays zoning and polysynthetic twinning. Some K-feldspars exhibit Carlsbad twinning and perthitic texture (Fig. 3c). Quartz is anhedral and in part fills interstices between other minerals. Granophyric intergrowths of quartz and alkali feldspar are common, suggesting emplacement at a shallow crustal level. The dominant ferromagnesian phase in these rocks is amphibole and tends to form euhedral to subhedral crystals. Some rocks contain biotite as an accessory phase occurring along the boundaries of K-feldspar and plagioclase, indicating that biotite crystallized later than plagioclase and K-feldspar. Sericite, actinolite, chlorite, calcite, titanite and epidote are also locally present as secondary phases.
In the porphyric granodiorite and granite, K-feldspar, plagioclase, amphibole and quartz are the main constituents and commonly associated with minor biotite, zircon, titanite, apatite and Fe–Ti oxides. They contain abundant amphibole and plagioclase phenocrysts (Fig. 3d). Plagioclase has complex zoning with resorption surfaces and polysynthetic twinning. Quartz and K-feldspar are anhedral and interstitial to the other minerals. Amphiboles occur as euhedral to subhedral tabular-shaped prismatic crystals and show greenish-brown pleochroism. The minor secondary minerals include sericite, chlorite, actinolite, calcite, epidote, titanite and iron oxide.
Dolerite dykes with basalt, andesite and trachyandesite compositions are generally porphyritic and microlitic in texture. Plagioclase and pyroxene are major phenocrysts in these units. Plagioclase, as a phenocryst or a lath-shaped microlite in the groundmass, is ubiquitous. Sieve-textured plagioclase is a common feature. Plagioclase crystals show various amounts of saussuritization. Pyroxene is present as euhedral and subhedral crystals in the basaltic dykes, but is locally altered to epidote, calcite and chlorite. Rarely, anhedral quartz and alkali feldspar occur as aggregates of smaller grains.
4. Analytical methods
A total of 33 samples were collected from representative and least-altered intrusive rocks including dykes. Following petrographic examination, 10 fresh and most representative samples were selected for geochemical analyses.
Granodiorite sample MA1 was selected for zircon dating. The sample (c. 3 kg) was crushed and sieved before undergoing magnetic and heavy liquid separation. The analysed zircon grains were hand-picked under a binocular microscope, obtaining highly pure zircon grains (> 99%). About 80 zircon grains were mounted in epoxy together with the standard, and then polished. Zircon grains were studied by cathodoluminescence imaging and analysed for U–Pb isotopes by laser ablation inductively coupled plasma mass spectrometry (LA-ICP-MS) at the State Key Laboratory of Geological Processes and Mineral Resources (GPMR), China University of Geosciences, Wuhan (see Simonetti et al. Reference Simonetti, Heaman, Chacko and Banerjee2006). Detailed operating conditions for the LA system and the ICP-MS instrument and data reduction were described by Liu et al. (Reference Liu, Hu, Gao, Günther, Xu, Gao and Chen2008). The weighted mean U–Pb ages were calculated and Concordia plots were constructed using Isoplot 4.1 (Ludwig, Reference Ludwig2008).
The samples were analysed for major and trace elements including rare earth elements (REEs) at the ALS Minerals Laboratory in Ireland using fusion ICP-MS and ICP-AES, and also at Nagoya University in Japan using X-ray flourescence (XRF) (Rigaku ZSX PrimusII) and ICP-MS (Agilent 7700×). At ALS Minerals laboratory, samples (0.200 g) for ICP-AES analysis are added to lithium metaborate and/or lithium tetraborate flux (0.90 g), mixed well and fused in a furnace at 1000°C. The resulting melt is then cooled and dissolved in 100 mL of 4% nitric acid and 2% hydrochloric acid. This solution is then analysed by ICP-AES and the results are corrected for spectral inter-element interferences. Oxide concentration is calculated from the determined elemental concentration. In ICP-MS analysis at ALS, samples are added to lithium metaborate flux (0.90 g), mixed well and fused in a furnace at 1000°C. The resulting melt is then dissolved in 100 mL of 4% HNO3 and 2% HCl solution.
At Nagoya University, the major elements were analysed by XRF spectrometry. The glass beads for the XRF analysis were prepared as follows: 0.50 g of sample powder was mixed with 5.0 g of lithium tetraborate, and the mixture was melted at 1200°C for 12–17 min in a high-frequency bead sampler (Rigaku Co. Japan). As for quantitative analysis of trace elements and Sr–Nd isotopic analysis, 50–100 mg of the powder was decomposed in a covered Teflon beaker using 3 mL of HF (38%) and 0.5–1 mL of HClO4 (70%) at 120–140°C on a hot plate in a clean room until the powder was dissolved completely. The dissolved samples were then dried at 140°C on the hot plate using infrared lamps. After drying, the samples were dissolved in 10 mL of 2 M HCl and the residue was completely decomposed in a steel-jacketed bomb at 180°C for 2–5 days.
The Nd and Sr isotopic ratios were analysed using thermal ionization mass spectrometry (TIMS) at GPMR (China) and at Nagoya University (Japan). At GPMR, the sample powders were digested in Teflon bombs using a mixture of double-distilled HNO3 and HF acids at 190°C for 48 hours. The Sr and Nd elements were separated and purified in a clean laboratory using ion exchange columns of Dowex AG50WX12 cation resin and Eichrom Ln-Spec resin successively. At GPMR the Sr and Nd isotope ratios were measured using a Finnigan Triton TIMS. Isotopic ratios of 87Sr/86Sr and 143Nd/144Nd were normalized to 88Sr/86Sr = 8.375209 and 146Nd/144Nd = 0.7219, respectively. Measurements of standard NIST-SRM987 and La Jolla gave average values of 0.710254 ± 8 (n = 22) and 0.511847 ± 3 (n = 25) for ratios of 87Sr/86Sr and 143Nd/144Nd, respectively. The initial ratios of 87Rb/86Sr and 147Sm/144Nd were calculated using Rb, Sr, Nd and Sm concentrations determined by ICP-MS.
At Nagoya University, the isotope ratios of Sr and Nd were measured using a TIMS, VG Sector 54-30 and a GVI IsoProbe-T. The mass fractionations during the Sr and Nd isotope measurements were corrected according to the ratios of 86Sr/88Sr = 0.1194 and 146Nd/144Nd = 0.7219, respectively. In this work, NIST-SRM987 and JNdi-1 (Tanaka et al. Reference Tanaka, Togashi, Kamioka, Amakawa, Kagami, Hamamoto, Yuhara, Orihashi, Yoneda, Shimizu, Kunimaru, Takahashi, Yanagi, Nakano, Fujimaki, Shinjo, Asahara, Tanimizu and Dragusanu2000) were adopted as the natural Sr and Nd isotope ratio standards, respectively. During this study, repeated analyses of NIST-SRM987 and JNdi-1 standards gave average values of 87Sr/86Sr = 0.710256 ± 0.000006 (n = 5) and 143Nd/144Nd = 0.512110 ± 0.000002 (n = 2), respectively. Detailed descriptions of the quantitative and isotopic analyses in Nagoya University are provided by Azizi & Asahara (Reference Azizi and Asahara2013).
5. Results
5.a. Zircon U–Pb ages
Zircon grains from granodiorite sample (MA1) (Table 1) are mostly pale brown, euhedral to subhedral stubby prisms without any inherited core or new growth rim, and length to width ratios of c. 2:1 to 3:1. They have a Th/U ratio > 0.50 (average, 0.66), consistent with a magmatic origin (cf. Belousova et al. Reference Belousova, Griffin, O’Reilly and Fisher2002; Kirkland et al. Reference Kirkland, Smithies, Taylor, Evans and McDonald2015).
Table 1. Zircon U–Pb dating data for the Golshekanan granitoid

The results of LA-ICP-MS U–Pb isotopic analyses are presented graphically in Figure 4. Nineteen spots yield constant 238U–206Pb ages ranging from 36.1 to 38.9 Ma. The analyses give a weighted mean 238U–206Pb age of 37.6 ± 0.2 Ma (mean squared weighted deviation (MSWD), 0.41), which is identical to the concordia age (37.6 ± 0.3 Ma; MSWD, 0.022). The late Eocene (Priabonian) age is consistent with previously published data from other parts of the Mehrabad intrusive rocks (Sarjoughian & Kananian, Reference Sarjoughian and Kananian2017).

Fig. 4. Concordia diagram for zircon grains (MA1) from the Golshekanan granitoid.
5.b. Whole-rock chemistry
Whole-rock analyses of major and trace elements for all samples are reported in Table 2. The studied samples yielded low loss on ignition (LOI) values (mostly < 3 wt%), indicating low levels of hydrothermal alteration.
Table 2. Data for major (wt%) and trace elements (ppm) of Golshekanan granitoids. gr – granite; gd – granodiorite; di –diorite; dk – dolerite dyke

aALS Minerals Laboratory (Ireland); bNagoya University (Japan).
Analyses of this study and the previous data (H Aliashrafzadeh, unpub. MSc thesis, University of Tehran, 2013) indicate that the Golshekanan granitoid can be classified into two groups. In the classification diagram of Middlemost (Reference Middlemost1994), the intermediate samples plot in the fields of diorite and monzonite, whereas the felsic samples plot in the fields of granodiorite and granite and one dyke plots in the monzogabbro field (Fig. 5).

Fig. 5. Chemical classification diagram of Middlemost (Reference Middlemost1994). The studied samples plot in the granite, granodiorite, diorite and monzonite fields. Square – felsic rocks; diamond – mafic-intermediate. Open symbols are from H. Aliashrafzadeh (unpub. MSc thesis, University of Tehran, 2013).
The Golshekanan granitoid has high contents of SiO2 (61.1–71.5; average, 66.7 wt%), Al2O3 (13.2–16.8; average, 15.4 wt%), and CaO (1.3–7.1; average, 4.5 wt%) and low contents of Fe2O3 T (0.3–3.3; average, 1.3 wt%) and MgO (0.8–3.3; average, 1.8 wt%). Most of the samples from the studied granitoid mostly belong to the calc-alkaline series (see Ross & Bedard, 2009; Fig. 6a) and display metaluminous to mildly peraluminous characteristics, with ASI (molar Al2O3/(CaO+K2O+Na2O)) ranging from 0.73 to 1.10, consistent with the lack of typical peraluminous minerals or alkaline mafic minerals. The granitoid samples show I-type affinity according to the classification of Chappell & White (Reference Chappell and White1974) on the A/CNK (molar Al2O3/(CaO+Na2O+K2O)) versus SiO2 diagram (Fig. 6b).

Fig. 6. (a) Zr versus Y (Ross & Bédard, Reference Ross and Bédard2009) and (b) A/CNK versus SiO2 (Chappell & White, Reference Chappell and White1974) diagrams, indicating that most of the samples are calc-alkaline and metaluminous.
In the primitive mantle-normalized trace-element spider diagram (Sun & McDonough, Reference Sun, McDonough, Saunders and Norry1989) (Fig. 7a), most of the samples display trace-element patterns, marked by enrichment by large-ion lithophile elements (LILE), such as Cs, Ba and K, and the depletion of high-field-strength elements (HFSE), such as Nb, Ta, Ti and P. These features are recognized as a fingerprint of subduction-related magmas (e.g. Wilson, Reference Wilson1989; Pearce, Reference Pearce1996). In chondrite-normalized rare earth element (REE) plot (Sun & McDonough, Reference Sun, McDonough, Saunders and Norry1989; Fig. 7b) the samples have a relatively homogeneous pattern, with an enrichment of light REEs (LREE) and heavy REEs (HREE) by a factor of approximately 20–100 and 10–20, respectively. They also show LREE enrichment relative to HREE (LaN/YbN = 1.66–8.14) and flat HREE (GdN/YbN = 0.90–1.31) with negative Eu anomalies (average Eu/Eu* = 0.75), except one sample with Eu/Eu* = 1.02.

Fig. 7. (a) Primitive mantle-normalized spider diagrams and (b) chondrite-normalized REE patterns for representative samples of the Golshekanan granitoid. Normalization values from Sun & McDonough (Reference Sun, McDonough, Saunders and Norry1989).
5.c. Whole-rock Sr–Nd isotope ratios
Eight whole-rock samples were analysed for Rb–Sr and Sm–Nd isotopic compositions (Table 3). As illustrated in Figure 8, the samples mostly plot along the bulk earth vertical line and in the right quadrants of a conventional Sr–Nd isotope diagram. Initial 87Sr/86Sr ratios and ϵNd(t) values were calculated using the age of 37.6 Ma. The initial 87Sr/86Sr ratios range from 0.70440 to 0.70504, 143Nd/144Nd(t) ratios range from 0.51279 to 0.51286, and the ϵNd(t) values are positive in all cases, ranging from +4.0 to +5.2.
Table 3. Sr and Nd isotopic ratios from the Golshekanan granitoids

aGPMR (China); bNagoya University (Japan).

Fig. 8. 87Sr/86Sr(i) versus ϵNd(t) diagram indicates the source of the magma from the Golshekanan granitoid and is compared with (a) other igneous provinces in the UDMA and (b) other cogenetic suites over the world.
6. Discussion
6.a. Petrogenesis
The Golshekanan granitoid body has I-type calc-alkaline arc signatures. The following three models, or some combination of them, could account for the formation of these rocks: (1) fractional crystallization of peridotite mantle-derived melts with and (or) without contamination (e.g. Soesoo, Reference Soesoo2000); (2) partial melting of old mafic to intermediate meta-igneous crust (e.g. Roberts & Clemens, Reference Roberts and Clemens1993); and/or (3) partial melting of juvenile crust (e.g. Xiao et al. Reference Xiao, Zhang, Clemens, Wang, Kan, Wang, Ni and Liu2007; Peng et al. Reference Peng, Zhao, Fan, Peng and Mao2014; Zhao et al. Reference Zhao, Tan, Wei, Tian, Zhang, Liang and Chen2015).
The first model is not favoured for the Golshekanan granitoid, because felsic magmas are voluminous (> 95%) relative to intermediate magma. It has high SiO2 contents, with low MgO, Cr and V contents compared with magmaic rocks that would be derived by partial melting of the mantle (e.g. van Middelaar & Keith, Reference van Middelaar, Keith, Stein and Hannah1990).
A very low-degree hydrous partial melting of mantle could produce andesitic melts, but to produce high volumes of felsic magma is physically difficult and practically unlikely (e.g. Mo et al. Reference Mo, Hou, Niu, Dong, Qu, Zhao and Yang2007). Moreover, there are no contemporary mafic volcano-plutonic complexes in the studied area. The variations in incompatible element ratios potentially indicate that they can be generated by variable degrees of partial melting or by fractional crystallization. In the diagrams of La versus La/Sm (e.g. Zhao et al. Reference Zhao, Tan, Wei, Tian, Zhang, Liang and Chen2015) and versus La/Yb (e.g. Jiang et al. Reference Jiang, Wang, Wyman, Li, Yang, Shi, Ma, Tang, Gou, Jia and Guo2014) (Fig. 9), the data display a clear ascending trend, indicating that the magma was dominantly controlled by partial melting and not by fractional crystallization.
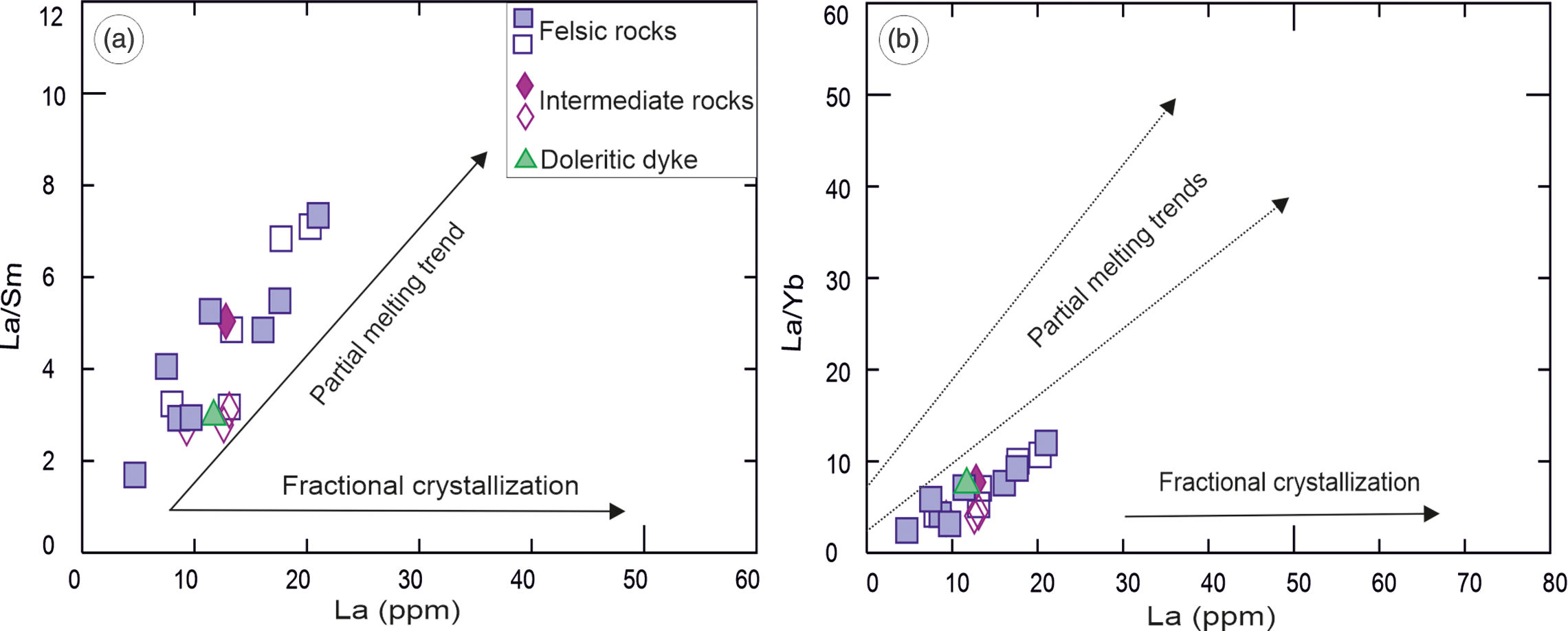
Fig. 9. La against (a) La/Sm and (b) La/Yb diagrams, illustrating trends of partial melting against fractional crystallization processes.
The uniform zircon and lack of inherited zircons in the studied samples probably indicate that crustal contamination is relatively insignificant during ascent and emplacement of the Golshekanan granitoid (Li et al. Reference Li, Qin, Li, Cao, Xiao, Chen, Zhao, Evans and McInnes2012).
Most of the Golshekanan granitoid rocks have MgO < 2 wt%, CaO > 2 wt%, FeOT > 2 wt% and TiO2 < 1.0 wt%. These features are emulated in high temperature melting experiments (1000°C) using amphibolite as the starting material (e.g. Beard & Lofgren, Reference Beard and Lofgren1991; Rapp & Watson, Reference Rapp and Watson1995). It is postulated that entrainment of peritectic phases may produce magmas more mafic than granite (Clemens et al. Reference Clemens, Stevens and Farina2011; Clemens & Stevens, Reference Clemens and Stevens2012), similar to some of the studied samples. The low K2O/Na2O value rules out an affinity to meta-pelitic, meta-greywacke and meta-andesitic sources (Fig. 10a; Patiño Douce, Reference Patiño Douce, Castro, Fernandez and Vigneresse1999). These features are analogous to those of the Cordilleran calc-alkaline granites resulting from partial melting of a meta-basaltic source (Patiño Douce, Reference Patiño Douce, Castro, Fernandez and Vigneresse1999). Variation in SiO2 and Al2O3 also point to magma generated from H2O undersaturated basaltic amphibolite at low pressures (Fig. 10b; Gürsu, Reference Gürsu2016). Large proportions of meta-basaltic continental lower crust have a composition similar to that of amphibolite facies metamorphic terrains (Hacker et al. Reference Hacker, Kelemen and Behn2015).

Fig. 10. Molar (a) K2O/Na2O versus CaO/(MgO+FeOT) (Patiño Douce, Reference Patiño Douce, Castro, Fernandez and Vigneresse1999); (b) Al2O3 versus SiO2 (Gürsu, Reference Gürsu2016); and (c) Al2O3/(FeOT+MgO+TiO2) versus Al2O3+MgO+TiO2+FeOT (Douce, Reference Douce1999) diagrams for samples from the Golshekanan granitoid. MB – metabasalt; MA – meta-andesite; MGW – metagreywacke; MP – metapelite. Data from Patiño Douce (Reference Patiño Douce, Castro, Fernandez and Vigneresse1999).
Wyllie & Wolf (Reference Wyllie, Wolf, Prichard, Alabaster, Harris and Neary1993) and López & Castro (Reference López and Castro2001) have experimentally shown that amphibolites start to melt at relatively high temperatures (800–900°C) at pressures < 1 GPa, while dehydration melting commences at temperatures as low as 750°C at < 1 GPa. The experimental data recommend that the partial melting of the mafic lower crust could produce melts of metaluminous composition irrespective of the degree of the partial melting, and less silicic melts were derived from high degrees of partial melting in the lower crust (e.g. Rushmer, Reference Rushmer1991; Rapp & Watson, Reference Rapp and Watson1995). It is concluded that the Golshekanan granitoid magma was initially generated from low-pressure partial melting in the plagioclase stability field, which is also confirmed by Al2O3/(FeOT+MgO+TiO2) versus Al2O3+MgO+TiO2+FeOT discrimination diagram (Fig. 10c; Douce, Reference Douce1999).
The (La/Yb)N values in the Golshekanan samples are lower than those in magma derived from a source with garnet as the major phase ((La/Yb)N < 20), although low Yb (average, 2.1 ppm) and Y (average, 18.9 ppm) of the studied rocks could show the presence of residual hornblende in the source (e.g. Martin, Reference Martin1987; Tang et al. Reference Tang, Wang, Wyman, Chung, Chen and Zhao2017). In conclusion, the weak HREE fractionation and also negative Eu anomalies suggest a residual plagioclase + hornblende + pyroxene assemblage without garnet in the melting region (e.g. Guo et al. Reference Guo, Fan, Li, Gao and Miao2009).
The above discussion proposes that the Golshekanan granitoid magma was generated in lower crust, but there are two different types of lower crust in orogenic belts, that is, ancient basement and more juvenile crusts. According to the tracer isotopic results, these granitoid melts are partial melts of juvenile crust; perhaps this juvenile source is the mixing product between juvenile and ancient basement crust during the Neo-Tethyan subduction.
In comparison with the igneous rocks equivalents from the UDMA, the Sr–Nd isotopic composition of the studied rocks is relatively similar to those from Khalkhab–Neshveh (Rezaei-Kahkhaei et al. Reference Rezaei-Kahkhaei, Galindo, Pankhurst and Esmaeily2011), Haji Abad (Kazemi et al. Reference Kazemi, Kananian, Xiao and Sarjoughian2019), Gheshlagh–Aftabrow (Kazemi et al. Reference Kazemi, Kananian, Yilin and Sarjoughian2020), Saveh (Nouri et al. Reference Nouri, Azizi, Stern, Asahara, Khodaparast, Madanipour and Yamamoto2018), Zafarghand (Sarjoughian et al. Reference Sarjoughian, Lentz, Kananian, Ao and Xiao2018), Marshenan (Sarjoughian et al. Reference Sarjoughian, Azizi, Lentz and Ling2019), Soheyle–Pakuh (Sarjoughian et al. Reference Sarjoughian, Javadi, Azizi, Ling, Asahara and Lentz2020), Rabor–Lalehzar adakitic (Chekani Moghadama et al. 2018), Sarduiyeh (Nazarinia et al. Reference Nazarinia, Mortazavia, Arvinb, Hu, Zhao and Poosti2020) and Kuh–Panj (Asadi, Reference Asadi2018). Most of the data lie well above the right quadrant of the Sr–Nd diagram (Fig. 8a). Geological characteristics of intrusive rocks elsewhere in the UDMA are noteworthy (see Table 4).
Table 4. Geological characteristics and ages of intrusions elsewhere in the UDMA

Data for some subduction-related, cogenetic rocks from around the world also compare to the two Golshekanan granitoid phases (Fig. 8b). The Golshekanan isotopic signatures are similar to juvenile crust-derived granitoids rocks, as found in some parts of China and Russia (e.g. Jahn et al. Reference Jahn, Wu and Chen2000 a, b; Kröner et al. Reference Kröner, Kovach, Belousova, Hegner, Armstrong, Dolgopolova, Seltmann, Alexeiev, Hoffmann, Wong and Sun2014).
The positive ϵNd(t) can be produced by partial melting of juvenile lower crust, such as arcs, ophiolites and accretionary complexes (e.g. Gromet & Silver, Reference Gromet and Silver1987; Wu et al. Reference Wu, Jahn, Wilde and Sun2000). The juvenile crustal components in arc systems can be sourced either from crustal underplating during extension or from partial melting of a subducted oceanic crust (Zhang et al. Reference Zhang, Chen, Han, Zhao, Huang, Yang and Yan2016). However, significant oceanic slab partial melting is precluded by the low Sr/Y (average, 27.3) and La/Yb (average, 6.4) values.
To evaluate, a simple mixing model was applied (Fig. 11). We assume that the Golshekanan granitoid formed through magma mixing processes involving the juvenile magmas incorporating either old lower continental crustal (LCC) or upper continental crustal (UCC) material. We are use typical LCC compositions from Taylor & McLennan (Reference Taylor and McLennan1985) and UCC compositions from Rudnick & Fountain (Reference Rudnick and Fountain1995). It would be better to use the juvenile end-members that have been reported for Cadomian rocks from Iran, with an end-member from Sabzevar ophiolite (Shafaii Moghadam et al. Reference Shafaii Moghadam, Corfu, Chiaradia, Stern and Ghorbani2014).

Fig. 11. ϵNd(t) versus 87Sr/86Sr(i) isotopic modelling results for the Golshekanan granitoid and adjacent fields in the UDMA. Parameters for Sr–Nd isotopic modelling are from Sabzevar ophiolite (Shafaii Moghadam et al. Reference Shafaii Moghadam, Corfu, Chiaradia, Stern and Ghorbani2014), lower crust (Taylor & McLennan, Reference Taylor and McLennan1985) and upper crust (Rudnick & Fountain, Reference Rudnick and Fountain1995). NW UDMA including: Haji Abad (Kazemi et al. Reference Kazemi, Kananian, Xiao and Sarjoughian2019), Khalkhab–Neshveh (Rezaei-Kahkhaei et al. Reference Rezaei-Kahkhaei, Galindo, Pankhurst and Esmaeily2011), Saveh (Nouri et al. Reference Nouri, Azizi, Stern, Asahara, Khodaparast, Madanipour and Yamamoto2018) and Gheshlagh–Aftabrow (Kazemi et al. Reference Kazemi, Kananian, Yilin and Sarjoughian2020). Central UDMA including: Marshenan (Sarjoughian et al. Reference Sarjoughian, Azizi, Lentz and Ling2019), Zafarghand (Sarjoughian et al. Reference Sarjoughian, Lentz, Kananian, Ao and Xiao2018) and Soheyle–Pakuh (Sarjoughian et al. Reference Sarjoughian, Javadi, Azizi, Ling, Asahara and Lentz2020). SE UDMA including: Rabor–Lalehzar (Chekani Moghadam et al. Reference Chekani Moghadam, Tahmasbi, Ahmadi-Khalaji and Santos2018), Sarduiyeh (Nazarinia et al. Reference Nazarinia, Mortazavia, Arvinb, Hu, Zhao and Poosti2020) and Kuh-Panj (Asadi, Reference Asadi2018).
The modelling calculation results suggest that a considerable proportion of juvenile crust (c. 90–95%) and slight ancient lower crustal components (c. 5–10%) were involved in the magma formation. We also used Sr–Nd isotope data on other intrusive rocks in the UDMA. As shown in Figure 11, the isotope modelling results are similar to Golshekanan granitoid with 60–95% mantle-derived juvenile crustal composition with crustal contamination. It is of course highly possible that other parameters, such as the mantle source (asthenospheric versus lithospheric, mantle wedge, etc.) could have affected our results.
6.b. Paleocene magmatism in the UDMA
The Palaeogene–Neogene magmatic flare-up was one of the most significant magmatic events in the UDMA, which occurred during 53.9–5.3 Ma (e.g. Chiu et al. Reference Chiu, Chung, Zarrinkoub, Melkonyan, Pang, Lee, Wang, Mohammadi and Khatib2017; Sarjoughian & Kananian, Reference Sarjoughian and Kananian2017; Babazadeh et al. Reference Babazadeh, Ghorbani, Cottle and Bröcker2019) within an Andean-type belt. The isotopic signature of the Golshekanan granitoid rocks is compared with that of well-known igneous rocks from UDMA (Fig. 1b, c). The Golshekanan granitoid and adjacent igneous rocks in the UDMA have almost similar major- and trace-element contents. Isotopically, almost all data lie well above quadrants near and along the bulk earth vertical line of a conventional Sr–Nd isotope diagram (Fig. 8a). The Golshekanan granitoid has significantly higher ϵNd(t) values and lower 87Sr/86Sr(i) ratios than the Kuh–Panj (Asadi, Reference Asadi2018), Zafarghand (Sarjoughian et al. Reference Sarjoughian, Lentz, Kananian, Ao and Xiao2018) and Marshenan (Sarjoughian et al. Reference Sarjoughian, Azizi, Lentz and Ling2019) intrusions. It also has slightly higher ϵNd(t) values and lower 87Sr/86Sr(i) ratios than the Khalkhab–Neshveh (Rezaei-Kahkhaei et al. Reference Rezaei-Kahkhaei, Galindo, Pankhurst and Esmaeily2011), Haji Abad (Kazemi et al. Reference Kazemi, Kananian, Xiao and Sarjoughian2019), Gheshlagh–Aftabrow (Kazemi et al. Reference Kazemi, Kananian, Yilin and Sarjoughian2020), Saveh (Nouri et al. Reference Nouri, Azizi, Stern, Asahara, Khodaparast, Madanipour and Yamamoto2018), Sarduiyeh (Nazarinia et al. Reference Nazarinia, Mortazavia, Arvinb, Hu, Zhao and Poosti2020) and Rabor–Lalehzar (Chekani Moghadam et al. Reference Chekani Moghadam, Tahmasbi, Ahmadi-Khalaji and Santos2018), and is similar to Soheyle–Pakuh (Sarjoughian et al. Reference Sarjoughian, Javadi, Azizi, Ling, Asahara and Lentz2020) (Fig. 8a). The similar isotopic signatures reveal the interaction of mantle-dominant juvenile melts with a greater contribution of old lower continental crust, where undoubtedly mantle material was injected into the magma. Deng et al. (Reference Deng, Wan, Dong, Talebian, Windley, Dadashzadeh, Mohammadi and Barati2018) postulated that most of the reported igneous rocks from the UDMA have intermediate to felsic compositions and only small volumes of mafic rocks, with low MgO, Cr and Ni values, inconsistent with the compositional characteristics of mantle source rocks. The partial melting of a mafic lower crust is therefore a more plausible mechanism for the generation of the UDMA. The Sr–Nd isotope compositions of samples from the UDMA are close to the CHUR (chondritic uniform reservoir) composition, which can also be regarded as the isotopic characteristics of the juvenile source, which could record Cambrian–Neoproterozoic crustal growth in the area.
The thermal anomaly associated with widespread upwelling of mantle caused selective and localized partial melting of juvenile crust to produce the melts in the UDMA during the Eocene–Miocene epochs.
6.c. Geodynamic implications and tectonic setting
Subduction of the Proto-Tethys underneath the Gondwana margin caused mantle-derived basaltic magmas to intrude the lower crust during Ediacaran–early Cambrian time, making the mafic juvenile lower crust of the UDMA.
During the late Eocene – Miocene period, the mantle upwelling in the UDMA responsible for partial melting of the juvenile lower crust in the extensional environment, as a result of oblique subduction (local transpression), might be a valid mechanism to produce the Golshekanan granitoid and nearby intrusive rocks under locally extensional tectonics. The Cenozoic extension was related to the steepening dip of the Neotethyan slab and trench rollback (e.g. Sepidbar et al. Reference Sepidbar, Ao, Palin, Li and Zhang2019). Slab rollback is a mechanism suggested to have caused Palaeogene magmatism throughout the UDMA during the transition from a compressional to an extensional convergent plate margin. The rollback followed a period of subduction, analogous to the Laramide and post-Laramide evolution of the western USA.
In Iran, during the closure of the Neo-Tethys basin, middle Eocene – early Oligocene extension and lithospheric thinning might have been accompanied by decompression melting of upwelling hydrous mantle (Verdel et al. Reference Verdel, Wernicke, Hassanzadeh and Guest2011). The extension may also have been accompanied by lithospheric delamination (e.g. Haschke et al. Reference Haschke, Ahmadian, Murata and McDonald2010; Shomali et al. Reference Shomali, Keshvari, Hassanzadeh and Mirzaei2011; Ahmadian et al. Reference Ahmadian, Sarjoughian, Lentz, Esna-Ashari, Murata and Ozawa2016), further initiating extension and rapid exhumation of the central Iranian core complexes (e.g. Sepidbar et al. Reference Sepidbar, Mirnejad, Ma and Moghadam2018).
Consequently, we postulate that the subduction of the Neo-Tethys in the UDMA may have been initiated during early Cenozoic time and the shallow dip of the slab penetrated the mantle beneath the central Iran microcontinent. With significantly enhanced convergence rates during Cenozoic time, the subducted slab descended more rapidly with a steeper dip, resulting in decompression melting and an influx of mantle magma with slab fluid to shallower depths, which supplied the primitive melts of UDMA plutonic rocks. The increase in the gradient and the decompression triggered melting of the juvenile lower crust (Fig. 12). Consequently, it is reasonable to suggest that the Golshekanan granitoid formed in an extensional setting and (or) transpression related to NE-wards subduction of the Neo-Tethys slab between Arabia and Eurasia.

Fig. 12. Schematic geodynamic model for the tectonic evolution of subduction of Neo-Tethys oceanic lithosphere under the Iranian continental plate and development of igneous rocks in the UDMA. The metasomatized mantle upwelling and lithospheric extension activities caused partial melting of mantle-derived juvenile lower crust rocks to generate the late Eocene Golshekanan in the UDMA (see text for the details).
7. Conclusions
The Golshekanan intrusive rocks are mostly granodiorite and granite, I-type affinity that crystallized at 37 Ma, based on the zircon U–Pb age. This body has low initial 87Sr/86Sr ratios and positive ϵNd(t) values, and geochemical features indicative of derivation from juvenile crust rocks during late Eocene time, when the entire UDMA was probably under lithospheric extension, with thinning of continental lithosphere as a result of the Neo-Tethys oceanic rollback. Injection of hot mafic magma increased the geothermal gradient in the crustal root zone that was then responsible for partial melting of juvenile mafic bodies in the UDMA.
Acknowledgments
This paper is part of the MSc thesis of BZ supervised by FS. We are grateful to Professor K. Zong at the State Key Laboratory, China University of Geosciences, Wuhan, who prepared the U–Pb–Th isotopic data. Some of the chemical data and analyses were supported by Nagoya University in Japan and JSPS KAKENHI grant no. 17H01671, Japan. DRL was supported by a NSERC Discovery grant.
Declaration of interest
None.