1. Introduction
The Ailaoshan tectono-magmatic-metallogenic belt (abbreviated as the Ailaoshan Belt) of southwestern China, along with its northwestern extension in the Jinshajiang Belt, is a significant component of the expansive Tethyan–Himalayan domain (e.g. Allegre et al. Reference Allegre, Courtillot, Tapponnier, Hirn, Mattauer, Coulon, Jaeger, Achache, Scharer, Marcoux, Burg, Girardeau, Armijo, Gariepy, Gopel, Li, Xiao, Chang, Li, Lin, Teng, Wang, Chen, Han, Wang, Deng, Sheng, Cao, Zhou, Qiu, Bao, Wang, Wang, Zhou and Xu1984; Sengör, Reference Sengör1987; Zhong, Reference Zhong2000; Zi et al. Reference Zi, Cawood, Fan, Tohver, Wang and McCuaig2012 a; Deng et al. Reference Deng, Wang, Li and Santosh2014). This belt contains volcanic and plutonic rocks and ophiolite mélange zones that extend from northeastern Tibet to the eastern Indochina Block (Lai et al. Reference Lai, Meffre, Crawford, Zaw, Halpin, Xue and Salam2014 a, b; Fig. 1a). The ophiolite mélange represents a suture zone that has been suggested to be the boundary between the South China Block and the Simao Block that were present within the Palaeo-Tethys Ocean (Chung et al. Reference Chung, Lee, Lo, Wang, Chen, Yem, Hoa and Wu1997; Sone & Metcalfe, Reference Sone and Metcalfe2008; Jian et al. Reference Jian, Liu, Kröner, Zhang, Wang, Sun and Zhang2009 a, b; Wang et al. Reference Wang, Deng, Li, Li, Yu and Qiao2014). Based on palaeontological and geochronological investigations, Zhong (Reference Zhong2000), Pan et al. (Reference Pan, Xu, Hou, Wang, Du, Mo, Li, Wang, Li, Jiang and Hu2003) and Liu et al. (Reference Liu, Wang, Cao, Zou, Tang and Chen2008) suggested that the Simao Block now bounded to the east with the Ailaoshan Belt was once a segment of the South China Block. In contrast, recent comprehensive studies of detrital zircon ages and Hf isotope compositions suggest that the Simao Block was derived from the Indochina Block (Wang et al. Reference Wang, Deng, Li, Li, Yu and Qiao2014; Yang et al. Reference Yang, Wang, Wang and Li2018). As a branch of the Palaeo-Tethys, the Ailaoshan Ocean between the South China Block and the Simao Block was in existence during Late Devonian – Early Carboniferous time (Wang et al. Reference Wang, Metcalfe, Jian, He and Wang2000; Pan et al. Reference Pan, Xu, Hou, Wang, Du, Mo, Li, Wang, Li, Jiang and Hu2003) and became extinct by subduction during early–late Permian time (Jian et al. Reference Jian, Liu, Kröner, Zhang, Wang, Sun and Zhang2009 b; Zi et al. Reference Zi, Cawood, Fan, Wang and Tohver2012 b). However, it is difficult to determine the precise timing and geometry of the suture due to structural complications caused by the collision of India with Asia in Cenozoic time. In particular, provenances and sedimentary ages of widespread Phanerozoic strata in the Ailaoshan Belt are still not clearly confirmed, making reconstruction of the Palaeo-Tethys evolution history still obscure. For example, the Jinchangyan Formation, which is the main host of Au mineralization in the Jichang Au–Ni deposit, has been regarded as Silurian or Devonian with large uncertainties (Internal Exploration Report, 1982; Xiong, Reference Xiong2014). Although several studies focused on magmatic geochronology in this belt and its links with the evolution of the Ailaoshan Ocean (e.g. Zi et al. Reference Zi, Cawood, Fan, Tohver, Wang and McCuaig2012 a, c; Wu et al. Reference Wu, Liu, Chen and Zhang2017; Xu et al. Reference Xu, Xia, Cai, Lai, Liu, Yang, Zhou, Ma and Zhang2020 a), there are limited studies focusing on the sediments in the belt and their connections between source, transportation pathway and sink basin. A detailed provenance study of the sediments in the Ailaoshan Belt would therefore be helpful to identify the initial source and how these materials were transported. These factors are fundamental for reconstructing the evolution of the Ailaoshan Ocean, a branch of the Palaeo-Tethys Ocean. In addition, a geochronological study of the auriferous strata would help to better constrain mineralization processes.

Fig. 1. (a) Tectonic framework of SE Asia with the location of the Ailaoshan Belt (AB), which is generated by continental extrusion of Tibet caused by the collision between the Indian and Euroasian plates. (b) Geological map of the Ailaoshan tectono-magmatic-metallogenic belt showing the stratigraphic and igneous components (after Yang et al. Reference Yang, Wang, Wang and Li2018). Locations and ages of igneous rocks were taken from Jian et al. (Reference Jian, Liu, Kröner, Zhang, Wang, Sun and Zhang2009 b), Liu et al. (Reference Liu, Deng, Liu and Shi2011, Reference Liu, Wang, Cawood, Fan, Cai and Xing2015), Wang et al. (Reference Wang, Deng, Li, Li, Yu and Qiao2014), Yang et al. (Reference Yang, Wang, Wang and Li2018). The size of symbols for gold deposits denotes variable reserves with large symbols indicating larger gold deposits and small symbols indicting medium-small gold deposits.
In this study, we report U–Pb ages and trace-element contents for detrital zircons extracted from the Au-mineralized chert and unconformably overlying meta-sandstones of the Jinchang mining district in the Ailaoshan Belt. Due to widespread distribution of the chert and the meta-sandstone strata in the Ailaoshan Belt, a provenance study of these formations is ideal for revealing the evolution of the Ailaoshan Ocean. In combination with previously reported data, our results improve the understanding of sediment provenance for the studied units and give new insights into the geodynamic setting and evolution of the Palaeo-Tethys.
2. Geological background
The Ailaoshan Belt connects the Jinshajiang suture to the NW and the Song Ma suture to the SE with extension into northern Vietnam (Metcalfe, Reference Metcalfe2006; Fan et al. Reference Fan, Wang, Zhang, Zhang and Zhang2010). The belt is about 500 km long and 20–100 km wide, and is thought to be the suture that separates the South China Block to the NE from the Simao Block to the SW (Fig. 1a); it is characterized by ophiolitic relics of mafic and ultramafic rocks (Leloup et al. Reference Leloup, Lacassin, Tapponnier, Schärer, Zhong, Liu, Zhang, Ji and Trinh1995; Jian et al. Reference Jian, Liu, Kröner, Zhang, Wang, Sun and Zhang2009 a, b; Lai et al. Reference Lai, Meffre, Crawford, Zaw, Halpin, Xue and Salam2014 a, b; Wang et al. Reference Wang, Deng, Li, Li, Yu and Qiao2014). Deep-penetrating NW-trending faults with moderate to steep dips (60–80° NE; Red River, Ailaoshan, Jiujia–Anding Amojiang faults) cut the belt. The faults are tightly clustered in the NW and divergent toward the SE (Fig. 1b).
The Proterozoic Ailaoshan Group lies between the Red River and Ailaoshan faults. The group is composed of upper greenschist- to lower amphibolite-grade metamorphic rocks with a total thickness of more than 10 km. Lithologies include gneiss, amphibolite, marble and schist that have yielded whole-rock Rb–Sr ages of 1000–1700 Ma (Yunnan Bureau of Geology and Mineral Resources, 1982). Formation of strata between the Ailaoshan and Jiujia–Anding faults is related to the collision of India and Eurasia during Cenozoic time and the lateral extrusion of the Indochina Block. Offsets of Permian–Triassic flood basalt successions and late Palaeogene highly potassic mafic magmatic rocks imply c. 600 km of left-lateral movement along the belt (Chung et al. Reference Chung, Lee, Lo, Wang, Chen, Yem, Hoa and Wu1997). Gold mineralization occurs between the Ailaoshan Fault and the Amojiang Fault. The Ailaoshan Fault separates the Ailaoshan Group metamorphic rocks to the east and Palaeozoic low-grade metamorphic rocks (including Yiwanshui and Jinchangyan formations) and volcanic rocks to the west. The Ailaoshan Belt is bounded on the west by the Simao Block, which is considered part of the Indochina Block (Wang et al. Reference Wang, Deng, Li, Li, Yu and Qiao2014). The basement of the Simao Block is mainly Precambrian high-grade metamorphic rocks (Zhong, Reference Zhong2000). The basement rock is overlain by thick Palaeozoic–Mesozoic sequences of low metamorphic grade. The oldest sedimentary rocks are Lower Ordovician slates, quartzites, marbles and phyllites, which are unconformably overlain by Middle Devonian – Middle Triassic shallow-marine clastic rocks and carbonates (Pan et al. Reference Pan, Xu, Hou, Wang, Du, Mo, Li, Wang, Li, Jiang and Hu2003). The Ailaoshan Belt was bounded on the east by the South China Block by the Late Triassic Epoch (Zi et al. Reference Zi, Cawood, Fan, Tohver, Wang, McCuaig and Peng2013). The Permian Emeishan Large Igneous Province extends over the western South China Block to the Jinshajiang–Ailaoshan suture.
The Jinchang Au–Ni deposit is located c. 10 km NE of Mojiang County near the Jiujia–Anding fault in the middle portion of the Ailaoshan Belt. The deposit consists of five ore blocks (from NW to SE): Sishibaliangshan, Laojinniu, Lanshan, Pingpo and Mobiliangzi (Xu et al. Reference Xu, Kong, Qu, Li, Qiu, Olin and Danyushevsky2020 b). The eastern segment of the Jinchang deposit is contained in the Jinchang intrusion (387–374 Ma, Jian et al. Reference Jian, Liu, Kröner, Zhang, Wang, Sun and Zhang2009 b), which consists of peridotites, gabbros, diabase and plagiogranite (Mo et al. Reference Mo, Lu and Shen1993). Wang et al. (Reference Wang, Groves, Deng, Li, Yang and Dong2019) suggested that the Jinchang Au–Ni deposit is an orogenic gold deposit. Based on pyrite trace-element composition and in situ sulphur isotope, Xu et al. (Reference Xu, Kong, Qu, Li, Qiu, Olin and Danyushevsky2020 b) suggested that the ore-forming fluids and metals were dominantly derived from the Jinchang ultramafic intrusion. The western segment is contained in the Triassic Yiwanshui Formation and low-grade metasedimentary rocks of the Jinchangyan Formation. The stratigraphy in the region has been inverted due to folding, with the Triassic Yiwanshui Formation unconformably overlain by the older Jinchangyan Formation. Both units were intruded by the Jinchang ultramafic intrusion (Xu et al. Reference Xu, Kong, Qu, Li, Qiu, Olin and Danyushevsky2020 b).
The Yiwanshui Formation consists of two members with a total thickness of more than 400 m. The upper member consists of sandstone red beds and grey–black siltstone with mudstone interlayers, in which occurs Lamellibranchia and plant fragment fossils (Internal Exploration Report, 1982). The lower member varies in thickness from metres to tens of metres and dominantly occurs in the west segment of the Jinchang mining district. Lithologies of the lower member include meta-sandstone, mudstone and conglomerate-bearing mudstone. The meta-sandstone is grey in colour and has a massive texture. Conglomerate-bearing mudstone occurs at the bottom of the lower member. Conglomerates are dominantly slate, chert and mafic rocks with diameter generally less than 1–2 cm.
The Jinchangyan Formation occurs as a NW-trending succession in the district with a thickness of 100–400 m (Fig. 2). The depositional age of the succession is uncertain due to the lack of geochronological work and the strong deformation in the region, but has been suggested on the basis of palaeontology to be early–middle Silurian (Internal Exploration Report, 1982). However, based on regional stratigraphic correlations, Xiong (Reference Xiong2014) proposed that the Jinchangyan Formation is of Devonian age. The formation consists of carbonaceous slate, chert, meta-sandstone, pebble-bearing sandstone, with minor mudstone, greenschist and tuffaceous sandstone. Carbonaceous slate and chert are the main hosts of the Au mineralization.

Fig. 2. Geological map of the Jinchang Au–Ni deposit in Mojiang County, the northern segment of the Ailaoshan Belt (modified after 1:20 000 Geological Map of Mojiang sheet, F-47-VI, 1976).
Three members have been identified for the Jinchangyan Formation. The lower member consists predominantly of dark-grey chert and discontinuous lenticular meta-sandstone beds commonly interbedded with carbonaceous slate. Weak silicification resulted from low-grade regional metamorphism and from subsequent hydrothermal overprinting. Chert is one of the most important hosts for Au mineralization and commonly crops out as cliffs due to the fact that it is highly resistant to weathering. There are two interpretations for the formation of chert in the Jinchangyan Formation: (1) hydrothermal alteration of clastic rocks; or (2) chemical sedimentation following felsic seafloor volcanism. The latter is favoured by Fang et al. (Reference Fang, Hu, Xie, Su and Qi2001) and Xie et al. (Reference Xie, Hu, Fang and Qi2001). The middle member consists of carbonaceous slate and meta-sandstone, with locally interbedded slate. The middle member is bracketed by a lower discontinuous greenschist bed and an upper limestone bed. No gold mineralization is found in this member, but weak Ni mineralization related to ultramafic intrusions is present. The upper member consists mainly of carbonaceous slate with calcareous slate interbeds, and lenticular bodies of granite porphyry. Neither Au nor Ni mineralization has been identified in this unit. Contacts between the three members appear to be conformable, but the unit is inverted due to isoclinal folding.
3. Sample descriptions
The Yiwanshui and Jinchangyan formations are widespread in the Ailaoshan Belt. Sedimentary age and provenance studies therefore potentially reveal the evolutionary history of the Ailaoshan Belt, and ultimately the Palaeo-Tethys Ocean. Two samples of meta-sandstone (LS-1 and LS-3) were obtained from outcrops of the Yiwanshui Formation within the Lanshan ore Block (23° 30′ 25″ N, 101° 45′ 11″ E). The c. 20 kg samples were taken from the same stratigraphic level but from sites separated by c. 15 m laterally. The outcrops were strongly weathered and showed abundant Fe oxyhydroxides (Fig. 3a); removal of weathered surfaces revealed a rock that was massive (LS-1) to laminated (LS-3) and grey in colour (Fig. 3b). Both samples consisted mainly of quartz (70–85%) and sericite (15–30%). Quartz was present as a coarser fraction (50–200 μm grains) set in a matrix of finer quartz (10–20 μm grains) and sericite. Sericite also occurred in 100–300 μm diameter masses pseudomorphing original coarse muscovite crystals.
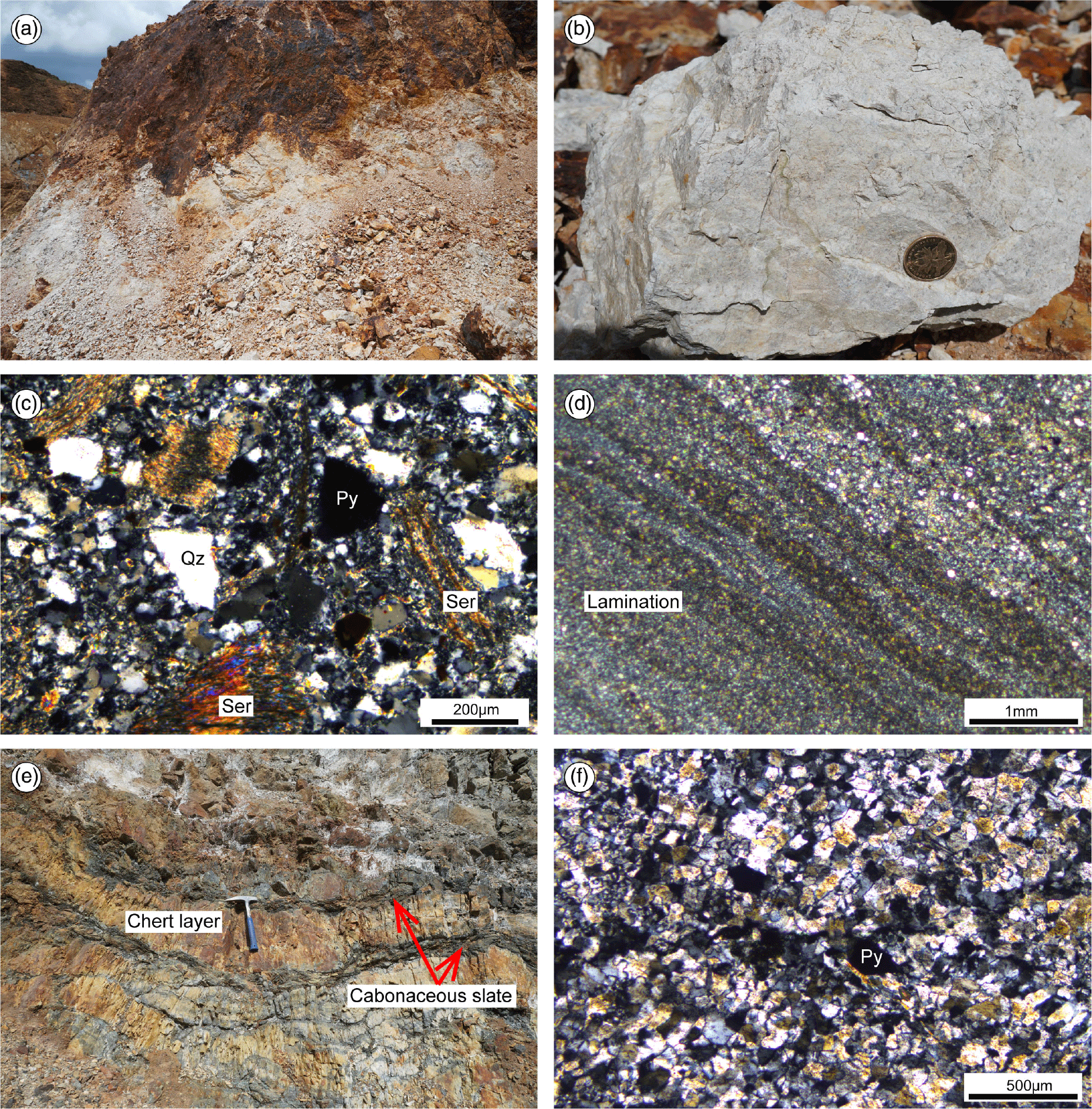
Fig. 3. Field and petrographic photographs of the meta-sandstone and chert samples from the Jinchang Au–Ni mine site examined for detrital zircon analysis. (a) Outcrop of meta-sandstone sampling site showing strong oxidative weathering (LS-1 and LS-3). (b) Hand specimen of sample LS-1, showing that the meta-sandstone sample is grey in colour and has a massive texture. (c) Thin-section petrology for sample LS-1. Coarse anhedral quartz occurs as phenocrysts surrounded by a fine-grained quartz and sericite matrix. The large sericites were altered from muscovite and keep the shape of the original minerals (cross-polarized light). (d) Meta-sandstone (LS-3) consists of fine-grained quartz and muscovite, and occurs as laminated relict texture (cross-polarized light). (e) Sample LS-52 is from a strata-bound Au-mineralized chert (10–30 cm thick), which is interlayered with thin layers of carbonaceous slate (5–20 cm thick). (f) Anhedral quartz is the dominant mineral in the chert, with minor anhedral pyrite intergrown (cross-polarized light). Qz – quartz; Ser – sericite; Py – pyrite.
A single sample of auriferous chert (LS-52) was collected from the lower member of the Jinchangyan Formation within the Lanshan ore block c. 50 m NE of the sample location of LS-1. The chert layers are typically strongly fractured, whereas the carbonaceous slate layers are unfractured, reflecting more ductile behaviour during deformation (Fig. 3e). Quartz makes up > 95% of the chert layer with minor amounts of sulphide minerals such as pyrite, pyrrhotite and gersdorffite (Fig. 3f). Quartz grains are typically uniform in size; the sample is characterized by a massive texture.
4. Analytical method and strategy
Samples were crushed to 60–80 mesh for zircon selection. To avoid potential contamination from interbedded slate, we collected the chert sample from a c. 50-cm-thick chert layer, and only chert from the middle of the layer was used (Fig. 3e). Because the chert is much harder than the slate, it is easy to wash away slate powders from chert. Zircon grains were separated by conventional heavy liquid and magnetic techniques followed by handpicking under a binocular microscope at the Research Center for Geoanalysis, Hebei Province. Approximately 150, 200 and 150 zircons were collected from LS-1, LS-3 and LS-52, respectively. Zircon grains without cracks and fluid inclusions were mounted onto double-sided adhesive tape and enclosed in an epoxy resin disc that was then polished to expose the crystals. The zircons were photographed under transmitted and reflected light, then internal structures were examined using cathodoluminescence (CL) images obtained with an EMPA-JXA-8100 scanning electron microscope at the Beijing GeoAnalysis Co. Ltd. U–Pb isotope data were obtained by laser ablation inductively coupled plasma mass spectrometry (LA-ICP-MS) at the Nanjing FocuMS Technology Co. Ltd. using an Agilent 7700X equipped with an ASI RESOlution S-155 193 nm excimer ArF laser ablation system. Zircons analysed were selected at random. The analytical procedures and instrument conditions are described in Xie et al. (Reference Xie, Zhang, Zhang, Sun and Wu2008) and summarized here. Each acquisition incorporated 20 s background (gas blank) and 40 s sample signals with laser ablation. The laser beam had a repetition rate of 10 Hz and a spot diameter of c. 30 μm. Helium was applied as carrier gas to efficiently transport aerosol out of the ablation cell, and was mixed with argon via a T-connector before entering an ICP torch. Dwell times were set to 20 ms for 207Pb, 15 ms for 206Pb and 208Pb, 10 ms for 232Th and 238U, and 8 ms for other elements. The fractionation correction was performed using the program ICPMSDataCal 9.7 (Liu et al. Reference Liu, Hu, Zhong, Gao, Gao, Xu and Chen2010). Harvard zircon 91500 was used as a calibration standard to correct instrumental mass discrimination. Trace-element concentrations were calculated using GLITTER 4.0 (Macquarie University) and calibrated using 29Si as internal standard, and NIST SRM 610 served as external reference material. After every eight unknown analyses, both standards were analysed to monitor accuracy and precision. 204Pb has a potential isobaric interference from 204Hg; this potential problem was monitored indirectly by monitoring 202Hg. 202Hg was usually < 10 cps in the gas blank, which indicates that the contribution of 204Hg to 204Pb was negligible. Common Pb was corrected according to the method of Andersen (Reference Andersen2002). The weighted mean U–Pb ages and Concordia plots were obtained using ISOPLOT 4.15 (Ludwig, Reference Ludwig2010). Errors in individual analyses were based on counting statistics and are reported as 1σ. The U–Pb ages were calculated using U decay constants recommended by Steiger and Jäger (Reference Steiger and Jäger1977), with which the individual analyses all fell within 1σ error at a 95% confidence level.
5. Results
5.a. Zircon U–Pb ages
The U–Pb data for zircons from meta-sandstone samples LS-1 and LS-3 are listed in Table 1. CL images with spot locations and 206Pb/238U ages are shown in Figure 4. The zircons targeted for U–Pb dating generally have clear oscillatory CL zoning and high Th/U ratios (> 0.1), indicating their initial magmatic origin (Allen et al. Reference Allen, Williams, Stephens and Fielding1998; Rubatto & Gebauer, Reference Rubatto, Gebauer, Pagel, Barbin, Blanc and Ohnenstetter2000; Hoskin & Schaltegger, Reference Hoskin and Schaltegger2003). They are typically transparent, light brown in colour, 100–200 μm in length and 50–100 μm in width (Fig. 4). A large proportion of zircons are excluded from analysis due to their fracture texture or dark colour, indicating strong Pb loss. As a result, only 24 analyses were performed for each sample. Among them, data with discordance rate lower than 95% are not used for concordant age calculation.
Table 1. Zircon LA-ICP-MS U–Pb age data for samples LS-1 and LS-3 from the Jinchang Au–Ni deposit in the northern segment of the Ailaoshan Belt

Note: PbT and PbC denote the total and common portions, respectively; *indicates that the data are not used for concordant age calculation.

Fig. 4. Cathodoluminescence (CL) images of zircons with 206Pb/238U ages used for U–Pb dating extracted from the meta-sandstone sample (a) LS-1 and (b) LS-3. Circles denote the location of laser ablation. Zircons with white circles are used for the concordant U–Pb age calculation.
Except for one discordant analysis (LS1-24), all zircons from LS-1 contained 128–652 ppm U and 73.5–339 ppm Th, with Th/U ratios varying from 0.33 to 1.59. A total of 16 analyses yielded 206Pb/238U ages of 243.8–259.2 Ma, interpreted as the youngest age population. When four disconcordant ages (LS-1-3, -7, -16 and -23) were disregarded, a concordant age of 252.8 ± 0.9 Ma (mean square weighted deviation (MSWD), 5.3; n = 12) (Fig. 5a) is interpreted. Seven analyses of older zircons (LS-1-2, -5, -8, -9, -11, -12 and -13) yielded 206Pb/238U ages older than 400 Ma. CL images of zircons with older ages are either brighter or darker and commonly anhedral in shape (Fig. 4). Although not exclusive, the CL images still offer alternative support to distinguish the older zircons from the younger group.

Fig. 5. Zircon U–Pb Concordia diagrams for the zircons with youngest populations from the meta-sandstone (a) LS-1, (b) LS-3 and (c) joint LS-1+3, as well as (d) all zircons analysed from LS-1+3.
The U and Th concentrations of zircons from sample LS-3 vary from 37.3 to 1036 ppm and from 41.7 to 643 ppm, respectively, with Th/U ratios of 0.09–1.19. A total of 14 analyses yielded 206Pb/238U ages of 244.9–255.9 Ma, judged to be the youngest population in the sample. Taking this population gives a concordant age of 249.2 ± 0.8 Ma (MSWD, 0.06; n = 14) (Fig. 5b). Ten analyses of this sample (LS-3-1, -4, -6, -8, -11, -12, -17, -18, -22 and -23) yielded much older ages. CL images of these zircons have a dark-grey colour, distinguishing zircons with younger ages that are consistently light-grey in colour (Fig. 4). Because samples LS-1 and LS-3 are stratigraphically equivalent, it is reasonable to combine the data to obtain a more precise age. The grouped data yield a concordant age of 250.8 ± 0.6 Ma (MSWD, 2.8; n = 26) (Fig. 5c), which can be considered the maximum depositional age for the Yiwanshui Formation.
The U–Pb data of zircons from the chert sample (LS-52) are listed in Table 2. CL images with spot locations and 206Pb/238U ages are shown in Figure 6. In contrast with the zircons from the meta-sandstone which are euhedral, zircons from the chert sample are mostly rounded, suggesting significant transport. In addition, zircon size is variable ranging from 60 to 200 μm in diameter. The analysed zircons are bright-grey to dark-grey in colour, and most lack zonation (Fig. 6). We omitted four analyses (LS-52-06, -11, -15 and -17) due to poor concordance. The remaining zircons contain 14.5–374 ppm U and 9.79–283 ppm Th, with Th/U ratios varying from 0.41 to 1.25. These results imply that the zircons are of magmatic origin. Another four analyses are disconcordant and yielded either much older or much younger ages (LS-52-20, -21, -22 and -24). CL images of these zircons are much darker than other zircons (Fig. 6). The remaining 16 analyses yielded 206Pb/238U ages of 343.7–354.1 Ma, with a concordant age of 347.0 ± 1.5 Ma (MSWD, 0.11) (Fig. 7), which can be considered a maximum age for sedimentation of the chert layer.
Table 2. Zircon LA-ICP-MS U–Pb age data for sample LS-52 from the Jinchang Au–Ni deposit in the northern segment of the Ailaoshan Belt

Note: PbT and PbC denote the total and common portions, respectively; *indicates that the data are not used for concordant age calculation.

Fig. 6. Cathodoluminescence (CL) images of zircons with 206Pb/238U ages used for U–Pb dating extracted from the chert sample LS-52. Circles denote the location of laser ablation. Zircons with white circles are used for the concordant U–Pb age calculation.

Fig. 7. Zircon U–Pb Concordia diagrams for the zircons with (a) the youngest populations and (b) all zircons from chert sample LS-52.
5.b. Trace-element compositions
The trace-element compositions of zircons used for concordant age calculation are listed in Table 3. Rare earth element (REE) patterns for the meta-sandstone zircons are similar to those for the chert layer zircons. Both exhibit enrichment in heavy REEs (HREEs), positive Ce anomalies and negative Eu anomalies (Fig. 8). The total REE concentrations of the meta-sandstone zircons vary from 1287 to 3551 ppm (mean, 2265 ppm), which is much lower than the concentrations in the chert zircons of 3759–14 315 ppm (mean, 8848 ppm).
Table 3. Trace-element composition of zircons used for the concordant age calculation from samples LS-1, LS-3 and LS-52


Fig. 8. Rare earth element (REE) concentrations for zircons from the meta-sandstone (black solid lines) and the chert (red solid lines). The REE values are normalized to C1 chondrite (McDonough & Sun, Reference McDonough and Sun1995). The distribution pattern is generally similar, with HREE-enriched, positive Ce anomalies and negative Eu anomalies. Total REE contents in zircons from the chert are higher than those from the meta-sandstone.
The uranium (U) concentrations of meta-sandstone zircons vary from 232 to 1036 ppm (mean, 462 ppm). This is one order of magnitude higher than the U concentrations of zircons from the chert sample, which vary from 14.5 to 47.5 ppm (mean, 31.6 ppm). Hafnium (Hf) compositions exhibit similar patterns. The Hf concentration of meta-sandstone zircons varies from 8595 to 10 916 ppm (mean, 9091 ppm), which is slightly higher in general than that of the chert of 7945–9887 ppm (mean, 8664 ppm). In contrast, the ytterbium (Yb) concentration of the meta-sandstone zircons ranges from 242 to 694 ppm (mean, 406 ppm), much lower than the Yb concentration of the chert zircons, which varies from 765 to 2626 ppm (mean, 1677 ppm). Similar to Yb, phosphorous (P) contents vary from 207 to 785 ppm (mean, 396 ppm) for the meta-sandstone zircons, while the chert sample shows higher contents of 658–2535 ppm (mean, 1520 ppm). The U/Yb ratios of the meta-sandstone zircons are two orders of magnitude higher than the U/Yb ratios of the chert zircons of 0.95–1.82 and 0.012–0.026, respectively. The Nb/Yb ratios of meta-sandstone zircons vary from 0.003 to 0.153, which is generally higher than the ratios of the chert zircons (0.002–0.004).
6. Discussion
6.a. Detrital zircon U-Pb ages
Detrital zircon geochronology has developed into an essential tool for studies of clastic strata during the past two decades. A basic tenet of detrital zircon geochronology is that a sedimentary unit can be no older than its youngest detrital zircon grains. Hence, the youngest U–Pb age in a population of detrital zircons constrains the maximum depositional age of the host stratigraphic unit (e.g. Stewart et al. Reference Stewart, Gehrels, Barth, Link, Christie-Blick and Wrucke2001; Surpless et al. Reference Surpless, Graham, Covault and Wooden2006; Dickinson & Gehrels, Reference Dickinson and Gehrels2009; Gehrels, Reference Gehrels2014). This approach is especially useful for dating metamorphosed strata that lack biostratigraphic age controls. However, there are potential complications that can result in measured dates that are younger than the true age of deposition, including Pb loss, poor precision of analysis and uncertainty of the decay constant for 238U (Gehrels, Reference Gehrels2014). This bias can be partly accounted for by using the youngest group of ages from a sample after evaluating the possibility of Pb loss using CL images (Dickinson & Gehrels, Reference Dickinson and Gehrels2009). In this study, we used concordant U–Pb ages for the youngest group of zircons to constrain the maximum depositional age of the auriferous chert of the Jinchangyan Formation and overlying meta-sandstone of the Yiwanshui Formation in the Jinchang Au deposit. The zircons from meta-sandstone samples LS-1 and LS-3 yielded youngest U–Pb ages of 243.8 ± 2.6 Ma (LS-1-03) and 244.9 ± 3.2 Ma (LS-3-09), which is only slightly younger than the concordant U–Pb ages of 252.8 ± 0.9 Ma and 249.2 ± 0.3 Ma, respectively. The close agreement between the single youngest zircon and the concordant age for each sample supports the conclusion that our concordant U–Pb ages give a robust maximum age of the sedimentary strata. With respect to sample LS-52, the youngest zircon yields a U–Pb age of 144.6 ± 1.4 Ma (LS-52-06) and shows a high degree of disconcordance compared with the much older concordant U–Pb age of 347.0 ± 1.5 Ma. We interpret the offset between the youngest and the concordant age to result from Pb loss in grain LS-52-06. Zircons LS-52-06, LS-52-21, LS-52-22 and LS-52-24, with a very dark colour in CL images, were omitted from the calculation of the concordant age to avoid inaccuracy due to Pb loss. The concordant age of the youngest zircons from the chert sample constrains the maximum depositional age of Au-bearing chert of 347 Ma. Our new age for the chert sample therefore suggests that the auriferous chert of the Jinchangyan Formation is Early Carboniferous or younger, and not Silurian or Devonian as suggested by previous workers (Internal Exploration Report, 1982; Fang et al. Reference Fang, Hu, Xie, Su and Qi2001; Xiong, Reference Xiong2014).
6.b. Provenance constraints
Most of the c. 250 Ma detrital zircons observed in this study have euhedral to subhedral morphology (Fig. 4), implying a short transport distance from their source region. Mesozoic intrusive and volcanic rocks are pervasive along the Ailaoshan Belt. Based on both radiometric and geochemical data, Zi et al. (Reference Zi, Cawood, Fan, Tohver, Wang, McCuaig and Peng2013) classified magmatic rocks in the Jinshajiang–Ailaoshan Belt into three groups: pre-collision granitoids, syn-collision volcanic rocks and post-collision granitoids. The pre-collision subduction-related magmatism consists mainly of granite, diorite and monzogranite. Zircon U–Pb dating indicates that the pre-collision magmatism occurred during 257–245 Ma, with peak ages at c. 250 Ma (Table 4). Examples include the granite dyke at Laowangzhai, c. 80 km NW of the Jinchang deposit, which has a concordant age of 251 ± 1.4 Ma, similar to the Xin’anzhai monzogranite of 251–252 Ma and Baimaxueshan granodiorite and diorite of 248–253 Ma (Zi et al. Reference Zi, Cawood, Fan, Wang, Tohver, McCuaig and Peng2012 c; Liu et al. Reference Liu, Wang, Cawood, Fan, Cai and Xing2015). Syn-collision period is dominated by volcanic rocks, with zircon U–Pb ages varying from 239 to 249 Ma (Table 4). Examples include the rhyolites of Lücun and Renzhixueshan, which have zircon ages of 247 ± 1.8 Ma (Liu et al. Reference Liu, Deng, Liu and Shi2011) and 247.4 ± 2.1 Ma to 249 ± 1.6 Ma (Wang et al. Reference Wang, Wang, Wang and Zhang2011), respectively, consistent with the zircon ages of granitoids exposed along the Ailaoshan Belt of 237–247 Ma (Wu et al. Reference Wu, Liu, Chen and Zhang2017). The youngest episode of magmatism in the Jinshajiang–Ailaoshan Belt is related to post-collisional extension. Zircon U–Pb ages of post-collision granitoids such as Jianren and Ludian vary from 214 to 234 Ma (Jian et al. Reference Jian, Liu and Sun2003; Zhu et al. Reference Zhu, Hu, Bi, Zhong and Chen2011; Zi et al. Reference Zi, Cawood, Fan, Tohver, Wang, McCuaig and Peng2013). Our concordant zircon age for samples LS-1 and LS-3 of 250.8 ± 0.6 Ma overlaps the period of pre-collision subduction-related magmatism (Fig. 9). We therefore suggest that zircons in the meta-sandstones associated with the Jinchang Au–Ni deposits were transported from nearby pre-collision magmatic rocks in the Ailaoshan Belt.
Table 4. A compilation of age data for the late Permian – Early Triassic magmatic rocks in the Jinshajiang–Ailaoshan Belt. Magmatism stage classification as described by Zi et al. (Reference Zi, Cawood, Fan, Tohver, Wang, McCuaig and Peng2013)


Fig. 9. Concordia age of meta-sandstone sample LS-1+3 compared with temporal distribution of magmatic activities during late Palaeozoic – Triassic time in the Jinshajiang–Ailaoshan Belt and relations with major tectonic events, compiled by Zi et al. (Reference Zi, Cawood, Fan, Tohver, Wang, McCuaig and Peng2013).
Previous studies indicate that the Palaeo-Tethys Ocean opened by seafloor spreading during Early Carboniferous time, leading to the formation of the Jinshajiang ophiolitic mélange (Jian et al. Reference Jian, Liu, Kröner, Zhang, Wang, Sun and Zhang2009 b; Zi et al. Reference Zi, Cawood, Fan, Wang and Tohver2012b ). However, the ophiolite in the Ailaoshan Belt and its northwestern extension in the Jinshajiang did not form simultaneously. Wang et al. (Reference Wang, Metcalfe, Jian, He and Wang2000) obtained a U–Pb age of 362 ± 41 Ma for plagiogranite in the Ailaoshan Belt. Zircon ages for diabase and plagiogranite from the Ailaoshan ophiolite are 374–387 Ma (Jian et al. Reference Jian, Liu, Kröner, Zhang, Wang, Sun and Zhang2009 b), significantly older than the cumulate gabbro of 343.5 ± 2.7 Ma in the Jinshajiang ophiolite located c. 300 km NW of the Ailaoshan ophiolite (Jian et al. Reference Jian, Liu, Kröner, Zhang, Wang, Sun and Zhang2009 b). Similarly, SHRIMP U–Pb analysis of zircons from the Dongzhulin trondhjemite within the Jinshajiang ophiolite yields a mean 206Pb/238U age of 347 ± 7 Ma (Zi et al. Reference Zi, Cawood, Fan, Wang and Tohver2012 b). The c. 347 Ma detrital zircons in chert from the Jinchang mining district match the age of the Jinshajiang ophiolite, but deviate slightly from the Ailaoshan ophiolite age, which is geographically closer to Jinchang district. The detrital zircons in the auriferous chert may therefore have been derived from the Jinshajiang ophiolite. The morphology of the detrital zircons is sub-rounded to rounded (Fig. 6), consistent with extensive ablation during long-distance transportation from their source regions.
Detrital zircon U–Pb geochronology is commonly applied to provenance analysis by comparing the age distribution pattern with the magmatic history of possible source regions (e.g. Thomas, Reference Thomas2011; Gehrels, Reference Gehrels2014; Craddock et al. Reference Craddock, Fitzgerald, Konstantinou, Nereson and Thomas2017). Based on a comprehensive comparison of detrital zircon ages from a northern segment of the Ailaoshan Belt with ages from the Indochina Block and western part of South China Block, Wang et al. (Reference Wang, Deng, Li, Li, Yu and Qiao2014) suggested that the Ailaoshan Belt belongs to the Simao–Indochina Block and was derived from Indian Gondwana. This interpretation differs from previous interpretations of the South China Block origin (Jian et al. Reference Jian, Liu, Kröner, Zhang, Wang, Sun and Zhang2009 b; Zi et al. Reference Zi, Cawood, Fan, Tohver, Wang, McCuaig and Peng2013; Wu et al. Reference Wu, Liu, Chen and Zhang2017). A comprehensive compilation of zircon U–Pb ages indicates that the western part of the South China Block was not characterized by a pervasive magmatic event during early Palaeozoic time, as indicated by lack of detrital zircons of this age in strata derived from the South China Block (Wang et al. Reference Wang, Deng, Li, Li, Yu and Qiao2014; Yang et al. Reference Yang, Wang, Wang and Li2018). The abundance of detrital zircons with a concordant age of 347 ± 1.5 Ma in the auriferous chert sample from the Jinchang mining district therefore supports the interpretation that the Ailaoshan Belt was once united with the Simao Block (Fig. 10).

Fig. 10. Comparison of age distribution pattern of detrital zircons from the meta-sandstone and chert with the Simao Block and the South China Block. The curves for the Simao Block and the South China Block are from Wang et al. (Reference Wang, Deng, Li, Li, Yu and Qiao2014).
6.c. Tectonic implications
Since the advent of LA-ICP-MS techniques, zircon trace-element geochemistry has been widely applied to questions of sedimentary provenance and tectono-magmatic processes (e.g. Grimes et al. Reference Grimes, John, Kelemen, Mazdab, Wooden, Cheadle, Hanghøj and Schwartz2007, Reference Grimes, Wooden, Cheadle and John2015; Buret et al. Reference Buret, Wotzlaw, Roozen, Guillong, von Quadt and Heinrich2017; Schmitt et al. Reference Schmitt, Klitzke, Gerdes and Schäfer2017; McKay et al. Reference McKay, Jackson and Hessler2018; Xu et al. Reference Xu, Li, Gui and Zhang2018; Yan et al. Reference Yan, He, Beier and Klemd2018). Because heavy REEs more closely match Zr4+ in ionic radius than light REEs do (0.985 Å for Yb3+ versus 1.16 Å for La3+, respectively, compared with 0.84 Å for Zr4+), igneous zircons typically display HREE-enriched and light REE (LREE) -depleted patterns (Grimes et al. Reference Grimes, John, Kelemen, Mazdab, Wooden, Cheadle, Hanghøj and Schwartz2007). Substitution of trivalent REEs for Zr requires charge compensating cations in the tetrahedral site; P5+ is an ideal candidate for such charge compensation. This coupled substitution may apply to zircons of the Jinchang mining district because YPO4 contents of the zircons correlate positively with total REE contents (R 2 = 0.93; Fig. 11). REE concentrations of zircons from continental and oceanic crust overlap, which complicates the distinction of source-rock composition using zircon geochemistry alone (Grimes et al. Reference Grimes, John, Kelemen, Mazdab, Wooden, Cheadle, Hanghøj and Schwartz2007). Nevertheless, the compilation of Grimes et al. (Reference Grimes, John, Kelemen, Mazdab, Wooden, Cheadle, Hanghøj and Schwartz2007) indicates that, despite the overlap, zircons from oceanic crust commonly have variable but more elevated REE contents than continental zircons. Zircons from auriferous chert sample LS-52 exhibit REE distribution patterns similar to those of zircons from the meta-sandstone samples, but with higher total REE concentrations (Fig. 8). This indicates that the zircons used for the concordant age calculation of the chert sample may originate from oceanic crust, whereas the zircons from meta-sandstone may derive from a continental source.

Fig. 11. A positive correlation (R 2 = 0.93) between total REE and P concentration in zircons, indicating that P5+ and trivalent REEs were incorporated into the Zr site.
Because zircon U, Yb, Y and Hf contents vary among different geologic settings, it is possible to use such data to determine the provenance of continental crust or oceanic crust (e.g. Belousova et al. Reference Belousova, Griffin, O’Reilly and Fisher2002; Grimes et al. Reference Grimes, John, Kelemen, Mazdab, Wooden, Cheadle, Hanghøj and Schwartz2007, Reference Grimes, Wooden, Cheadle and John2015; Carley et al. Reference Carley, Miller, Wooden, Padilla, Schmitt, Economos, Bindeman and Jordan2014). Hf4+ and Zr4+ are closely similar in ionic radius (0.83 and 0.84 Å, respectively), so Hf is highly compatible in zircon and Hf concentrations can reach weight percent levels. U4+ has a larger ionic radius (1.0 Å) than Zr4+, resulting in a lesser compatibility in zircon than Hf. U and Yb have similar compatibility in zircon, but they show different compatibility in magmatic systems; U is enriched in the continental crust relative to the oceanic crust of mid-ocean ridge basalts, whereas Yb is relatively enriched in the oceanic crust (Grimes et al. Reference Grimes, John, Kelemen, Mazdab, Wooden, Cheadle, Hanghøj and Schwartz2007). Yttrium and Hf behave similarly to Yb. As a result of differences in compatibility, these elements and their ratios can distinguish different zircon provenances. Based on a compilation of trace-element compositions of zircons from variable geological settings, Grimes et al. (Reference Grimes, John, Kelemen, Mazdab, Wooden, Cheadle, Hanghøj and Schwartz2007, Reference Grimes, Wooden, Cheadle and John2015) and Xu et al. (Reference Xu, Li, Gui and Zhang2018) suggested that U versus Yb and U/Yb versus Hf, Y and Nb/Yb plots can be used to classify zircons from continental or ocean crust. In our samples, the zircons used for the concordant age calculation from meta-sandstone (LS-1 and LS-3) all fall within the continental crust field (Fig. 12). In combination with the zircon morphology and concordant age, we infer that these zircons were transported from continental-arc magmatic rocks located near the Mojiang site. The widespread exposure of c. 250 Ma granitoids along the Ailaoshan Belt also supports intensive magmatism related to the Palaeo-Tethys collision (Reid et al. Reference Reid, Wilson, Shu, Pearson and Belousova2007; Liu et al. Reference Liu, Deng, Liu and Shi2011; Zi et al. Reference Zi, Cawood, Fan, Tohver, Wang and McCuaig2012 a, c, 2013; Wang et al. Reference Wang, Deng, Li, Li, Yu and Qiao2014; Yang et al. Reference Yang, Wang, Wang and Li2018). The zircons from chert sample LS-52 fall within the oceanic crust field (Fig. 12), and are probably from a mid-ocean ridge as suggested by U/Yb versus Nb/Yb plot (Fig. 12d). Mafic rocks of ocean-crust origin of c. 347 Ma have been reported along the Jinshanjiang–Ailaoshan Belt; examples include the cumulate gabbro of 343.5 ± 2.7 Ma and the trondhjemite of 347 ± 7 Ma from the Jinshajiang ophiolite (Jian et al. Reference Jian, Liu, Kröner, Zhang, Wang, Sun and Zhang2009 b; Zi et al. Reference Zi, Cawood, Fan, Wang and Tohver2012 b). We therefore suggest that the detrital zircons in the chert unit in the Jinchang Au–Ni mining district was likely transported from the Jinshajiang–Ailaoshan ophiolite related to the branch Palaeo-Tethys Ocean seafloor spreading. The zircons in the chert layer derived from the newly formed oceanic crust.

Fig. 12. Application of (a) U v. Yb, (b) U/Yb v. Hf, (c) U/Yb v. Y and (d) U/Yb v. Nb/Yb plots as geochemical discriminant proxies for zircons from variable provenances. The areas of continental zircon and ocean crust zircon are after Grimes et al. (Reference Grimes, John, Kelemen, Mazdab, Wooden, Cheadle, Hanghøj and Schwartz2007, Reference Grimes, Wooden, Cheadle and John2015).
Zircon U–Pb dating suggests that the Ailaoshan ophiolite formed at 387–374 Ma (Jian et al. Reference Jian, Liu, Kröner, Zhang, Wang, Sun and Zhang2009 b), slightly older than its northwestern extension of the Jinshajiang ophiolite of c. 347 Ma (Zi et al. Reference Zi, Cawood, Fan, Wang and Tohver2012 b). Considering that the chert in the Jinchang mining district in the Ailaoshan Belt and the Jinshajiang ophiolite share similar zircon ages, we suggest that the detrital zircons in the chert were not transported from the nearby Ailaoshan ophiolite, but from the Jinchang ophiolite, which is c. 300 km NW of the Ailaoshan ophiolite. The long-distance transportation is further supported by the sub-rounded to rounded shape of zircons in the chert unit. The SE-directed transportation of sedimentary materials indicates that collision after the closure of the Ailaoshan Ocean would be more intensive in the NW segment than in the SE segment. Strong collision and subsequent weathering and erosion created abundant zircons, which were transported SE-wards, probably due to altitude difference. The chert sample has no zircons from the nearby Ailaoshan ophiolite, which may indicate that the Ailaoshan Ocean was still not completely closed during the deposition of chert formation, in line with the theory that the closure of the Ailaoshan Ocean occurred from the NW to the SE. During Palaeozoic – Early Triassic time, subduction-related magmatism was most likely intensive in the entire Ailaoshan Belt because of widespread magmatic rocks with similar ages at both NW and SE segments (Zi et al. Reference Zi, Cawood, Fan, Tohver, Wang and McCuaig2012 a, 2013). Sedimentary materials of meta-sandstone were dominantly transported from the nearby magmatic rocks as indicated by similar zircon U–Pb ages and their euhedral shapes. The continental crust of the Simao Block served as the source of detrital zircons and other sedimentary materials, which were eroded, transported and finally deposited in nearby basins. The Ailaoshan Belt underwent collision after the closure of the Palaeo-Tethys Ocean.
7. Conclusions
The Ailaoshan Belt in southwestern China is a significant tectono-magmatic-metallogenic province. Our LA-ICP-MS U–Pb geochronological and trace-element study of zircons from the Jinchang Au–Ni deposit in the Ailaoshan Belt yields the following conclusions.
-
(1) The Yiwanshui Formation meta-sandstone, unconformably overlying the Jinchangyan Formation auriferous chert and slate layers, contain abundant detrital zircons. A group of young zircons yielded a concordant U–Pb age of 250.8 ± 0.6 Ma, constraining the maximum depositional age of the meta-sandstone to the Permian–Triassic boundary.
-
(2) Detrital zircons from the Jinchangyan Formation chert exhibit variable U–Pb ages; a group of young zircons yielded a concordant U–Pb age of 347.0 ± 1.5 Ma, indicating that the chert formed later than Early Carboniferous time, and not during Silurian or Devonian time as suggested by previous workers.
-
(3) Zircons in the Yiwanshui Formation meta-sandstone were derived from nearby magmatic rocks of continental crust origin, which were generated by pre-collision subduction-related magmatism related to closure of the Palaeo-Tethys Ocean. Zircons in the Jinchangyan Formation chert were transported long distances from the Jinshajiang ophiolite that were related to seafloor spreading in a branch of the Palaeo-Tethys Ocean.
Acknowledgements
This work was funded by the National Key Research and Development Project of China (2016YFC0600307). We appreciate Mr. Shi-Duo Shen, the general chief geologist of Mojiang Mining Co., Ltd., for permitting access to the mine site and field assistance. Critical comments from Dr. Guido Meinhold and one anonymous reviewer, as well as discussion with Prof. Qing-Fei Wang and Dr. Lin Yang at CUG-Beijing improved the manuscript greatly. We thank Dr. Yan Chen at CUG-Beijing for microscopic assistance. We also thank Dr. Anja Frank and Dr. Craig Johnson for language editing, and the Editor-in-Chief for insightful guidance and handling.
Conflict of interest
None.