1. Introduction
Skarn ore deposits, namely contact-metasomatic ore deposits, commonly occur at the contact zone between intermediate–acidic intrusion and carbonate rock, or at a certain distance (Pohl, Reference Pohl2011). They are mainly formed by a variety of metasomatic processes involving fluids of magmatic, metamorphic, meteoric and/or marine origin (Meinert, Reference Meinert1992; Meinert et al. Reference Meinert, Dipple and Nicolescu2005).
Garnet in most skarn deposits is commonly marked by calcic skarn ranging from grossular end-member to andradite end-member, and its composition and zonal patterns record important geological information (Meinert et al. Reference Meinert, Dipple and Nicolescu2005). Generally, the major composition of garnet shows the evolutionary trend of Al-rich garnet to Fe-rich garnet from diffusive metasomatism to infiltrative metasomatism (Gaspar et al. Reference Gaspar, Knaack, Meinert and Moretti2008; Park et al. Reference Park, Song, Kang, Shim, Chung and Park2017 b). Furthermore, backscattered electron (BSE) images of single garnet crystal, in combination with in situ laser ablation – inductively coupled plasma – mass spectrometry (LA-ICP-MS), provide a possible method of revealing skarn-forming processes and fluid evolution. The rare earth element (REE) signature of garnet is controlled by crystal chemistry (e.g. substitution mechanisms, surface sorption, lattice relaxation energy and growth rate) and fluid chemistry (e.g. fluid composition, temperature, oxygen fugacity, pH and REE complex speciation) (Smith et al. Reference Smith, Henderson, Jeffries, Long and Williams2004), which can effectively reflect the physical and chemical properties of the ore-forming fluids (Zhai et al. Reference Zhai, Liu, Zhang, Wang, Su, Yang and Wu2014; Zhang et al. Reference Zhang, Liu, Shao and Li2017 a, b; Fu et al. Reference Fu, Sun, Li and Lin2018; Tian et al. Reference Tian, Leng, Zhang, Zafar, Zhang, Hong and Lai2019), the origin and evolution of fluid (Park et al. Reference Park, Song, Kang, Shim, Chung and Park2017 b; Ding et al. Reference Ding, Ma, Lu and Zhang2018; Fan et al. Reference Fan, Wang, Lü, Wei and Chen2018; Sun et al. Reference Sun, Wang, Wang, Long, Hu, Wang, Li and Xie2020; Yang et al. Reference Yang, Ni, Wang, Wang and Zhang2020 b).
The late Palaeozoic Yong’an–Meizhou depression belt (YMDB) is a tectonic–magmatic–metallogenic belt in SE China (Fig. 1a) that has experienced the transformation from Tethyan tectonic domain to circum-Pacific tectonic domain (Zhang, Reference Zhang1999). This important Fe-polymetallic mineralized belt hosts several economic iron ore deposits (e.g. the Makeng, Luoyang, Yindingge, Yangshan, Pantian and Zhongjia deposits; Fig. 1b). The Luoyang deposit is located in the southeastern part of the YMDB (Fig. 1b) and is a large-scale Fe deposit second only to Makeng. It contains c. 5.20 Mt of resources at a grade of 45.7% Fe. Skarns, especially garnet, occur widely in the Luoyang deposit. However, early studies have focused on the origin and evolution of ore-forming fluids (Yang et al. Reference Yang, Ni, Pan, Chi, Ding and Wang2020 a; Wang et al. Reference Wang, Zhang, Yu, Wu, Di, Zhang and Yao2021), the source of iron materials (Wang et al. Reference Wang, Ji, Xing and Wang1981, Reference Wang, Zhang, Yu, Wu, Di, Zhang and Yao2021; Zheng & Zhang, Reference Zheng and Zhang1988; Liu, Reference Liu1989), petrogenic and metallogenic geochronology (Zhang et al. Reference Zhang, Wu, Di, Wang, Yao, Zhang, Lv, Yuan and Shi2012, Reference Zhang, Cheng, Yang and Hu2018; Yang et al. Reference Yang, Ni, Wu, Ding and Zhu2017) and ore genesis (Zhao et al. Reference Zhao, Tan, Xu, Yuan, Zheng and Lin1980; Huang, Reference Huang1982, Reference Huang2011; Liu, Reference Liu1989). Moreover, no studies have examined garnet detailed textures, or major- and trace-element characteristics, which can be used to reveal the genesis of garnet and their hydrothermal fluid evolution.

Fig. 1. (a) Simplified structural map of South China showing the location of the study area. (b) A schematic regional geological map of the Yong’an–Meizhou depression belt in eastern China showing principal deposit localities (after Zhang et al. Reference Zhang, Zuo and Cheng2015).
In this paper we present a first detailed study of the textures of garnet varieties and their in situ major- and trace-element signatures, in order to reveal hydrothermal fluid redox conditions and their implications for Fe–Zn mineralization, and to further elucidate the origin and evolution of ore-forming fluids.
2. Geological setting
The South China Block consists of the Yangtze Block and the Cathaysia Block, separated by the Jiangshan–Shaoxing suture zone (Fig. 1a). Within the Cathaysia Block, the YMDB is located west of a late Mesozoic magmatic zone and south of the Precambrian Wuyishan Terrane (Fig. 1b). The YMDB has undergone crystallized basement formation during the Jinning period (2600–1000 Ma), Caledonian (670–400 Ma) metamorphic folded basement formation, Hercynian–Indosinian (390–195 Ma) sedimentary cover formation and Yanshanian (195–100 Ma) active continental margin (Mao et al. Reference Mao, Tao, Xie, Xu and Chen2001). The poly-cycle tectonic evolution caused multistage magmatism and associated mineralization (Zhang, Reference Zhang2015): (1) volcanogenic massive sulphides Pb–Zn–Cu–Ag deposits associated with Jinning period submarine volcanism; (2) Au deposits associated with Caledonian ductile shear zone; (3) porphyry Cu–Mo deposits and pegmatite rare metal deposits associated with Hercynian–Indosinian magmatism; and (4) Fe–Cu–Pb–Zn skarn polymetallic deposits related to Yanshanian intermediate acidic intrusion. This period of magmatism extensively occurred in the YMDB, in particular, distributed along the NE–SW-trending Zhenghe–Dapu fault (Fig. 1b) (Lin, Reference Lin2011).
Ore genesis and the geodynamic setting of mineralization at Luoyang deposit have been investigated in detail by many workers (Zhao et al. Reference Zhao, Tan, Xu, Yuan, Zheng and Lin1980; Huang, Reference Huang1982, Reference Huang2011; Liu, Reference Liu1989; Zhang et al. Reference Zhang, Wu, Di, Wang, Yao, Zhang, Lv, Yuan and Shi2012, Reference Zhang, Cheng, Yang and Hu2018; Yang et al. Reference Yang, Ni, Pan, Chi, Ding and Wang2020 a; Wang et al. Reference Wang, Zhang, Yu, Wu, Di, Zhang and Yao2021). Based on geological observations of lower and middle Carboniferous volcanic rocks, the Luoyang deposit was originally considered to be a submarine volcanogenic massive sulphide iron deposit (Huang, Reference Huang1982; Liu, Reference Liu1989). Another genetic interpretation (stratabound skarn type) was proposed by Yang (1980) to explain ore genesis on the basis of analysis of geological characteristics of iron orebody. Subsequently, increasing evidence from ore deposit geochemistry (Huang, Reference Huang2011; Yang et al. Reference Yang, Ni, Pan, Chi, Ding and Wang2020 a; Wang et al. Reference Wang, Zhang, Yu, Wu, Di, Zhang and Yao2021), petrologic geochemistry of granites (Yang et al. Reference Yang, Ni, Wu, Ding and Zhu2017; Zhang et al. Reference Zhang, Cheng, Yang and Hu2018), and petrogenic and metallogenetic ages (Zhang et al. Reference Zhang, Wu, Di, Wang, Yao, Zhang, Lv, Yuan and Shi2012; Wang et al. Reference Wang, Zhang, Yu, Wu, Di, Zhang and Yao2021) indicated that the Luoyang deposit belongs to a typical skarn-type iron deposit. Based on these studies, we propose the following metallogenic model for Luoyang deposit. As a key criterion in the identification of ore types, Cretaceous granite provides the main metallogenic material and iron mineralization fluid that were generated in an extensional environment responding to rollback of the subducting slab during early Cretaceous time. The contact metasomatism between lower Cretaceous granite and lower–middle Permian carbonate strata caused the formation of stratiform-like iron orebody and skarn.
3. Deposit geology
The Luoyang Fe skarn deposit is located in the southern part of YMDB with proven metal reserves of 14.4 Mt, accompanied by Mo and Zn metal elements. The exposed strata in the Luoyang mining area are the Carboniferous Lindi Formation detrital rocks and Chuanshan Formation carbonates, as well as the Permian Qixia Formation carbonates, the clastic rocks (e.g. sandstone, argillite and siltstone) of Permian Wenbishan, Tongziyan and Cuipingshan formations, and the Triassic Xikou and Wenbinshan formations mudstone and shale (Fig. 2). This area widely develops faults with mostly NE- and NW-directed strikes (Fig. 2). The NE-trending faults may control the emplacement of the granitoids, whereas the NW-trending faults cross-cut through NE-trending faults and all exposed strata. These carbonate and clastic rocks are intruded by rhyolite porphyry ranging in composition from diorite to granite (Fig. 2). Three main skarn Fe orebodies (no. 131, 23, and 2) occur as stratiform to lenticular in shape and are hosted in limestone of the upper Carboniferous Chuanshan and lower Permian Qixia Formations (Fig. 3). They dip to the NE, strike c. NW–SE (290–340°), and have lengths of 266–400 m, widths of 48–349 m and thicknesses of < 48 m. Alteration resulted in the formation of mainly calcic skarns that are zoned from granite porphyry to marble in the following sequence: granite porphyry → actinolite–pyrite±garnet–diopside skarn → garnet–diopside–magnetite±actinolite–pyrite skarn → marble (Fig. 3). Most iron mineralization occurs in the garnet–diopside skarn. In this zoned skarn sequence, garnet in the granite porphyry contact is abundant and occurs as coarse-grained crystals of green-light brown colour, which comprises a massive garnet skarn (Grt1 sampling site). Away from the granite porphyry, garnet is absent from the main body of the skarn and diopside–magnetite association is abundant, which constitutes the main iron orebody; locally, fibrous actinolite and coarse-grained pyrite assemblage replaced or cross-cut the diopside–magnetite skarn. Noticeably, yellow garnet veinlets (Grt2 sampling site) are widely developed in the diopside–magnetite skarn. In the marble front, the green–light-brown garnet becomes abundant away from the iron orebody and occurs as massive (Grt1 sampling site) in relict marble patches.
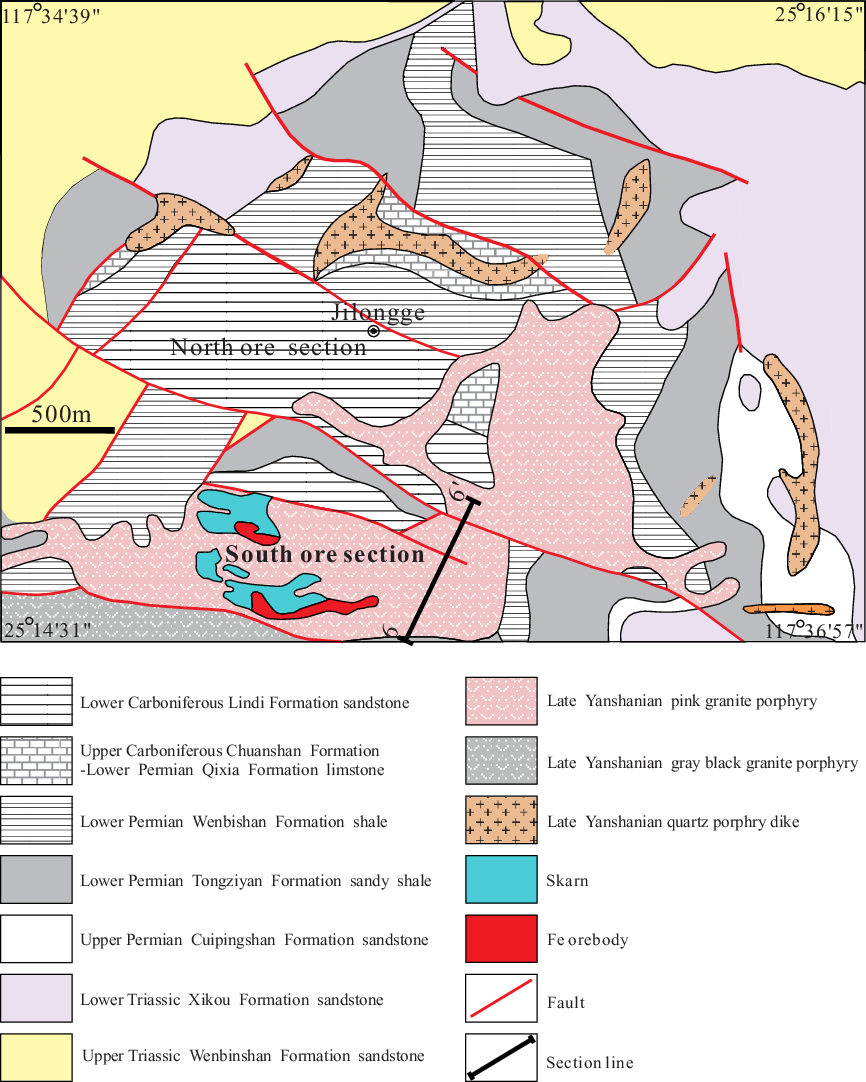
Fig. 2. Detailed geological map of the Luoyang iron deposit, showing the distribution of host rocks, Fe orebody, skarn and granitoids. The location of exploration line 6 is shown in this figure.

Fig. 3. Geological section along exploration line 6 in the Luoyang deposit and the location of this investigation area is also marked on the cross-section.
4. Sampling and methodology
4.a. Sample description
Garnet samples (samples LY40, LY45, LY50 and LY52) were collected from the Luoyang main iron orebody, sampled from depths of 40, 70 and 100 m. The garnet in sample LY40 from massive garnet skarn is millimetre to centimetre in size, dark green in colour, and is characterized by coarse-grained crystals (Grt1), along with disseminated fine-grained pyrite and calcite as interstitial particles within garnet (Fig. 4a). Sample LY45 is dominated by magnetite replacing garnet as relict (Grt1), and is locally filled with coarse-grained pyrite patches or veinlets (Fig. 4b). In thin-sections, Grt1 with rough surface is euhedral or subhedral textures and shows oscillatory growth zoning, which is locally replaced by calcite and minor magnetite (Fig. 5a, b). A large number of fine-grained magnetite grains occur between diopside grains and replaced the Grt1 (Fig. 5c–f). In sample LY50, garnet occurs as fine-grained particles filling between magnetite grains or as veinlets along fractures (Grt2) (Fig. 4c); some other minerals, such as coarse-grained pyrite and fibrous actinolite, also fill in the massive ore (Fig. 4c). Sample LY52 is massive iron ore with several garnet-bearing calcite veins (Grt2) and sulphide veinlets cross-cutting massive ore (Fig. 4d). In thin-sections, it is difficult to distinguish textural relationship between Grt2 and sphalerite, with the replacement of sphalerite by Grt2 (Fig. 6a, b) or sphalerite filling between Grt2 grains (Fig. 6c–d). However, these textural characteristics are observed in different parts of the same vein (Fig. 4d), implying that both Grt2 and sphalerite belong to the same generation.

Fig. 4. Hand specimens characteristic of different garnet generations collected from adit in the Luyang deposit. (a) Massive Grt1 filled with calcite or cross-cut by pyrite veinlets; (b) massive diopside–magnetite replaced by pyrite and actinolite, and Grt1 relict locally occurs in the ore; (c) pyrite, fine-grained Grt2 and actinolite replace massive magnetite; (d) sphalerite, Grt2 and calcite veins cross-cut massive. Grt1 – garnets of the first generation; Grt2 – garnets of the second generation; Di – diopside; Py – pyrite; Mt – magnetite; Cal – calcite; Act – actinolite; Sph – sphalerite.
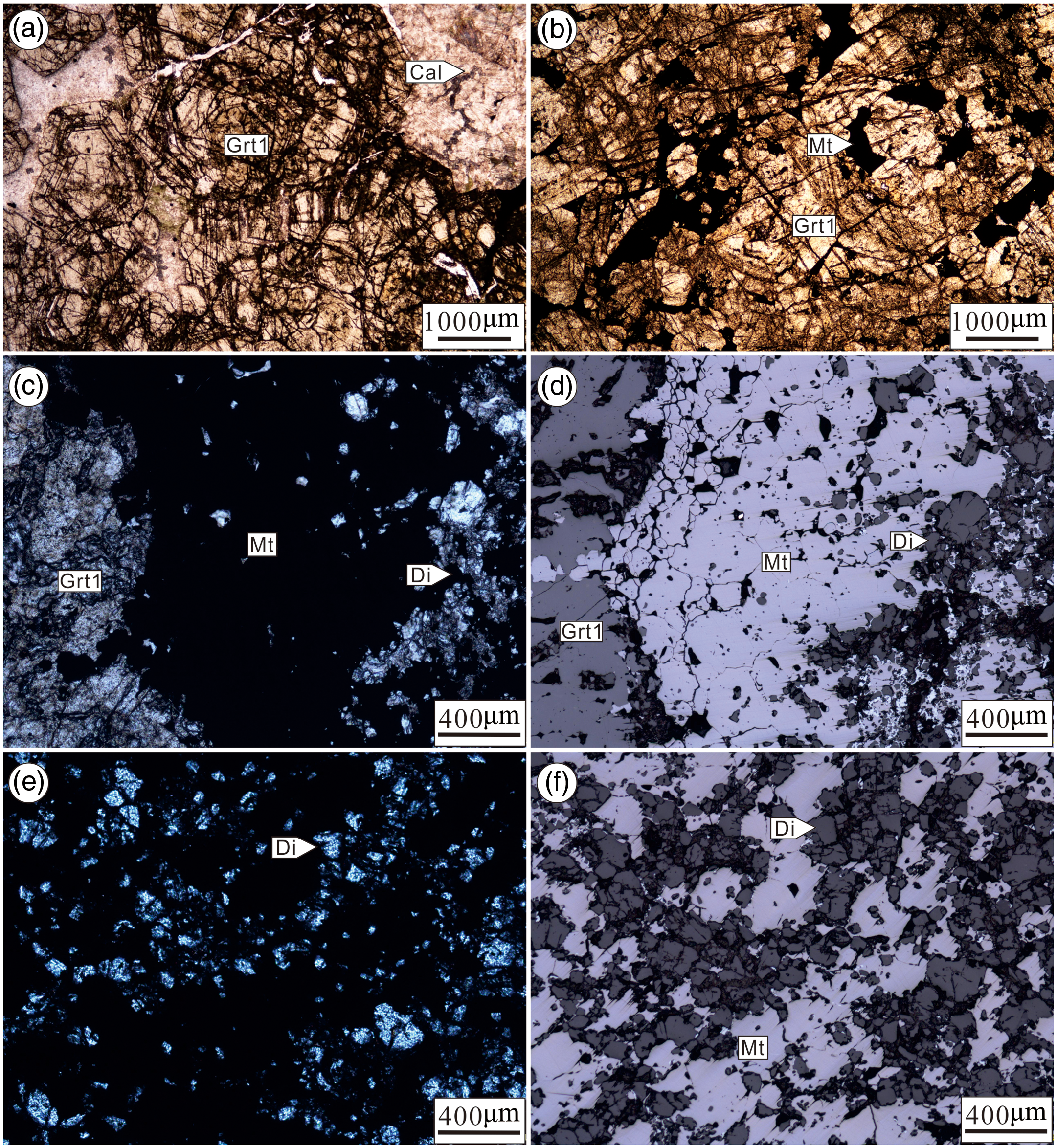
Fig. 5. Petrographic photomicrographs of Grt1 from the Luoyang Fe skarn assemblages. (a, b) Grt1 shows oscillatory zonings and is replaced by magnetite and calcite along their fractures. (c, d) Interstitial magnetite between diopside crystals which replaces Grt1. (e, f) Magnetite grains fill the area between diopside crystals, showing coexisting texture.

Fig. 6. Petrographic light microscope images and backscattered electron images of different garnet generations from the Luoyang deposit. (a, b) The fine-grained garnet (Grt2) together with sphalerite fills spaces between coarse-grained magnetite, overprinted by minor pyrite and molybdenite. (c, d) Yellow anhedral sphalerite and fine-grained calcite fill spaces between subhedral garnet (Grt3). (e) BSE image of Grt1 shows two distinct garnet generations: early garnet (Grt1-A) with oscillatory zoning and the later magnetite-grain-bearing irregular garnet (Grt1-B) replaces Grt1-A along its fissures. (f) BSE image of subhedral-shaped garnet (Grt2) without oscillatory zones. Cal – calcite; Mt – magnetite; Sph – sphalerite; Py – pyrite; Mo – molybdenite. Yellow dots and red numbers represent the LA-ICP-MS locations and spot numbers, respectively.
In BSE images, Grt1 can be further classified into two subtypes based on its textural characteristics: type A and type B garnets of the first generation (Grt1-A and Grt1-B, respectively). BSE images of Grt1-A shows conspicuous oscillatory zoning (Fig. 6e). Grt1-B images are characterized by irregular bands; Grt1-A is dissolved in the older dark parts (Fig. 6e). Noticeably, Grt1-B coexisted with some small magnetite grains (Fig. 6e). The grains of Grt2 lack oscillatory zonings in BSE images (Fig. 6f). The interstitial magnetite is observed as replacements in the fissures of Grt2 (Fig. 6f).
4.b. Analytical methods: scanning electron microscopy and in situ LA-ICP-MS analysis
All samples were prepared as polished thin-sections in which we identified the mineral assemblages, textures, alterations and metasomatic relationships. Our studies were conducted with a Nikon DS-Ri1 optical microscope at the State Key Laboratory for Mineral Deposits Research in Nanjing University. After a textural and petrographic study, two polished sections of representative garnet types from two selected samples (samples LY45 and LY50) were subjected to more detailed petrographic observations and systematic in situ major- and trace-element analyses.
Garnet zoning patterns were investigated by BSE studies prior to in situ LA-ICP-MS analysis. The BSE investigations were carried out using an analytical scanning electron microscope (JSMIT100) connected to a GATAN MINICL system at Wuhan Sample Solution Analytical Technology, Wuhan, China. The imaging conditions were 10.0–13.0 kV voltage and 80–85 μA current. Major- and trace-element compositions can be analysed precisely in situ by LA-ICP-MS without applying internal standardization by applying an ablation yield correction factor on the basis of the normalization of the sum of all major element oxides to 100 wt%, and using NIST 610 and USGS reference glasses BHVO-2G, BIR-1G and BCR-2G as multiple reference materials for calibration (Liu et al. Reference Liu, Hu, Gao, Günther, Xu, Gao and Chen2008). In situ analyses of the major and trace elements in garnet were performed with a Coherent GeoLas2005 laser ablation system coupled with an Agilent 7700e ICP-MS at Wuhan Sample Solution Analytical Technology. Helium was used as a carrier gas that was mixed with argon in a T-shaped switcher before entering the ICP-MS. The working parameters of the laser instrument were: a laser beam of 32 μm diameter, a repetition rate of 5 Hz and an energy of 80 mJ. It took 70 s to acquire the data for each analysis (20 s on background, 50 s on signal). The NIST 610 glass and the three USGS reference glasses were analysed at the beginning of each session, and the analysis of every set of six samples was followed by one analysis of NIST 610 as an external standard for quality control. The preferred values of element concentrations in the USGS reference glasses were taken from the GeoReM database (http://georem.mpchmainz.gwdg.de/). Details of the analytical and data processing methodology are described by Liu et al. (Reference Liu, Hu, Gao, Günther, Xu, Gao and Chen2008). The raw ICP-MS data were treated offline using ICPMS DataCal software 10.8 and using a 100% normalization strategy without applying internal standards (Liu et al. Reference Liu, Hu, Gao, Günther, Xu, Gao and Chen2008). The detection limit for most elements was below 0.1 ppm.
5. Results
5.a. Garnet major-element geochemistry
The LA-ICP-MS analytical results for the three generations of garnet are given in online Supplementary Table S1 (available at http://journals.cambridge.org/geo) and its major and trace elements are summarized in Table 1. The results generally show that garnet types from the Luoyang deposit belong to the grossular-andradite solid solution with compositions ranging from Adr79.5Grs15.9 to almost pure andradite (Adr99.4) (Fig. 7). The spessartine, pyrope and almandine contents are collectively less than 9% (online Supplementary Table S1). We have compiled data for the major-element compositions of garnets from other skarn deposits, and the data demonstrate that garnet is dominated by grossular–andradite (Fig. 7). Compared with garnets from typical Fe skarn deposits worldwide (Meinert et al. Reference Meinert, Dipple and Nicolescu2005), from the Makeng Fe skarn deposit in the same belt (Yang et al. Reference Yang, Ni, Wang, Wang and Zhang2020 b) and the Tieshan Fe skarn deposit in the middle–lower Yangtze river metallogenic belt (Wang et al. Reference Wang, Shang, Zhang, Wei and Wang2019) (Fig. 7), garnet composition in the Luoyang deposit has relatively narrow variations and is featured by distinctive Fe-enriched andradite (Fig. 7). Grt1-A is relatively homogeneous in composition with relatively low andraditic contents [Adr79.5-88.4]. Grt1-B has generally more andraditic (Fe-rich) [Adr91.5-95.6]. Grt2 is nearly pure andradite [Adr95.7-99.4] with similar andraditic composition to Grt1-B.
Table 1. Summary of features of two generations of garnets from the Luoyang deposit.


Fig. 7. Diagram showing major-element compositions and dominance of end-member garnets from the Luoyang deposit, with comparison to garnet from Fe skarn deposits worldwide (Meinert et al. Reference Meinert, Dipple and Nicolescu2005), and from the Makeng Fe deposit (Yang et al. Reference Yang, Ni, Wang, Wang and Zhang2020 b) and the Tieshan Fe–Cu deposit (Wang et al. Reference Wang, Shang, Zhang, Wei and Wang2019). Pyr – pyrope; Sps – spessartine; Alm – almandine; Grs – grossular; Adr – andradite.
5.b. Garnet trace-element geochemistry
The LA-ICP-MS trace-element data of the garnets are presented in online Supplementary Table S1, and major and trace elements of all garnets are summarized in Table 1. Grt1-A has highest total REE contents ranging over 381–1339 ppm, and high LREE/HREE ratios (1.01–10.4). The garnets mainly show negative Eu anomalies (δEu = 0.46–1.24), have high U (31.8–128 ppm) and Y (89.3–633 ppm) content, and high-field-strength element (HFSE = 10.6–94.7 ppm) content. Grt1-B has distinctively low total REE contents (ΣREE = 138–197 ppm), high LREE/HREE ratios (1.79–12.8) and show mainly negative Eu anomalies (δEu = 0.39–2.50). They have lower U (12.6–22.3 ppm), Y (26.6–146 ppm) and HFSE (2.18–15.3 ppm) contents than those of Grt1-A. In contrast, Grt2 has lowest total REE contents (ΣREE = 3.40–88.6 ppm), U (0.04–0.78 ppm), Y (4.60–84.4 ppm) and HFSE (0.26–1.27 ppm), with significantly negative Eu anomalies (δEu = 0.10–0.54).
The chondrite-normalized REE patterns for garnet in each generation also correspond to variations in their major elements and textures (Fig. 8). Grt1-A has a flat to moderately downwards-sloping (LREE relatively enriched and HREE depleted) trend with weak negative Eu anomalies, whereas Grt1-B shows slightly right-sloping profiles with slight LREE enrichments and weak negative Eu anomalies (Fig. 8a). The REE patterns of Grt2 show a flat profile with slight LREE enrichment and distinctively negative Eu anomaly overall (Fig. 8b).

Fig. 8. Chondrite–normalized REE patterns for the different generations of garnets. The chondrite-normalized values were calculated using the normalizing factors of Sun & McDonough (Reference Sun, McDonough, Saunders and Norry1989). Included for reference are REE distribution patterns of granitoids and marble in the Luoyang area. Data from Yang et al. (Reference Yang, Ni, Wu, Ding and Zhu2017).
6. Discussion
6.a. REE substitution mechanism
The general chemical formula of garnet can be written as X3Y2Z3O12, where X2+ is Ca2+, Mg2+, Mn2+ or Fe2+ in eightfold coordination, Y3+ is Al3+, Fe3+ or Cr3+ in octahedral coordination and Z4+ is mainly Si4+ in a tetrahedral coordination (Deer et al. Reference Deer, Howie, Wise and Zussman1997; Gaspar et al. Reference Gaspar, Knaack, Meinert and Moretti2008). As a calc-silicate mineral, garnet can incorporate a certain amount of REEs3+ as substitutes for Ca2+ (Pan & Fleet, Reference Pan and Fleet1996), which generates a hump-shaped REE pattern with Pr peak (Fig. 9a). However, the substitution of REE3+ into garnet is heterovalent, which requires coupled substitutions to maintain the charge balance (Gaspar et al. Reference Gaspar, Knaack, Meinert and Moretti2008; Park et al. Reference Park, Song, Kang, Shim, Chung and Park2017 b). The four main charge balanced substitutions have been suggested by previous studies (Jaffe, Reference Jaffe1951; McIntire, Reference McIntire1963; Enami et al. Reference Enami, Bolin, Yoshida and Kawabe1995; Smith et al. Reference Smith, Henderson, Jeffries, Long and Williams2004; Gaspar et al. Reference Gaspar, Knaack, Meinert and Moretti2008; Grew et al. Reference Grew, Marsh, Yates, Lazic, Armbruster, Locock, Bell, Dyar, Bernhardt and Medenbach2010; Park et al. Reference Park, Song, Kang, Shim, Chung and Park2017 b):





Fig. 9. (a) Plot of ionic radii of trivalent REEs and bivalent Eu in eightfold co-ordination against the absolute difference in radii with eightfold coordinated Ca2+; (b) total REEs versus percentage of andradite end-member component in garnet; (c) total REEs versus total Al (AlIV + AlVI) in atoms per formula unit (apfu), and (d–f) plots of ionic radii of trivalent REEs and bivalent Eu in eightfold co-ordination against the absolute difference in radii with eightfold coordinated and tetrahedral coordinated (d) 2Ca2+(VIII)–Na+(VIII), (e) Ca2+(VIII)+Si4+(IV)–Al3+(IV) and (f) Ca2+(VIII)+Al3+(VI) –Fe2+(VI). Data from Shannon (Reference Shannon1976). The solid circle and triangle in the grey fields are after Park et al. (Reference Park, Song, Kang, Shim, Chung and Park2017 b) and Gaspar et al. (Reference Gaspar, Knaack, Meinert and Moretti2008), respectively.
where X2+ is dominantly Ca2+ in eightfold coordination, X+ represents Na+, Y2+ is Mg2+ or Fe2+, Y3+ is mainly Al3+, Z3+ is Al3+ or Fe3+, [] is a Ca site vacancy, and VIII, IV, and VI represent 8-, 4- and 6-fold coordination, respectively. Substitution mechanism (1) mainly involves high Na contents in fluid (McIntire, Reference McIntire1963; Enami et al. Reference Enami, Bolin, Yoshida and Kawabe1995; Smith et al. Reference Smith, Henderson, Jeffries, Long and Williams2004). However, the very low Na concentrations (online Supplementary Table S1) make it difficult to detect possible correlations and evaluate the role of this mechanism. Substitution mechanism (3) involves charge compensation by substituting trivalent cations into the octahedral site (Grew et al. Reference Grew, Marsh, Yates, Lazic, Armbruster, Locock, Bell, Dyar, Bernhardt and Medenbach2010). However, octahedral Mg and Fe2+ (both calculated by stoichiometry) have very low contents and neither the calculated Mg or Fe2+ contents correlate with the REE3+ contents (online Supplementary Table S1), suggesting that substitution (3) is not the main substitution mechanism. Substitution mechanism (4), involving the creation of structural vacancies, is harder to evaluate but cannot be completely ruled out. Coupled substitution (2) is a possible substitution, which depends on Fe3+ or Al3+ content in fluid (Jaffe, Reference Jaffe1951; Gaspar et al. Reference Gaspar, Knaack, Meinert and Moretti2008; Park et al. Reference Park, Song, Kang, Shim, Chung and Park2017 b). The negative total REE3+ versus andradite composition (Fig. 9b) and positive total REE3+ versus total Al (AlIV + AlVI) correlations (Fig. 9c) indicate that the incorporation of REEs followed mechanism (2). Although andradite composition and total Al have a narrow range of variations (Fig. 9b, c), the variation of total REE3+ is obvious. Our results show that Grt1 with relatively Al-rich andradite has higher total REE contents (138–1339 ppm) than Grt2 (3.40–88.6 ppm) with nearly pure andradite and Grt1-A with Al-rich sections enriched in total REE (381–1339 ppm) in relation to Grt1-B with Fe-rich sections (Table 1), which also demonstrates the negative correlation between andradite components and total REE contents and positive correlation between total Al and total REE contents. Park et al. (Reference Park, Song, Kang, Shim, Chung and Park2017 b) reported that REE contents increase with increasing andradite composition, which indicates that garnet in a Fe3+-enriched environment would have higher REE concentrations, assuming that the physicochemical factors (P, T, pH and the host rock) are similar during garnet growth. However, our results showed that the REE contents were positively correlated to total Al (Fig. 9c), which was also reported by Gaspar et al. (Reference Gaspar, Knaack, Meinert and Moretti2008). This result potentially indicates that the incorporation of REEs should preferentially concentrate in fluids in an Al-enriched environment. It is pointed out that this substitution mechanism is the only way for the incorporation of REEs into garnet, although some workers (e.g. Ding et al. Reference Ding, Ma, Lu and Zhang2018) explained REE patterns of garnet using the mechanisms above on the basis of calculated ionic radius (Fig. 9d–f), and cannot determine REE patterns. Assuming that the growth of the studied garnets is not influenced by external factors (e.g. fluid), they show HREE-enriched and LREE-depleted patterns as indicated by Figure 9e on the basis of coupled substitution (2). However, two generations of garnets do not show HREE-enriched and LREE-depleted patterns and instead show LREE-enriched to flat patterns (Fig. 8), which most likely suggests that there are other external factors (e.g. fluid composition and physicochemical conditions) controlling the growth of garnets. REE patterns are controlled by REE partition coefficient between garnet and fluid, which is affected by fluid composition and physicochemical factors (e.g. T, pH, fO2) (Smith et al. Reference Smith, Henderson, Jeffries, Long and Williams2004).
6.b. Fluid redox conditions and implications for Fe–Zn mineralization
6.b.1. Fluid redox conditions
The redox state of hydrothermal fluids can be indicated by variable valence elements (e.g. Eu and U). Eu and U occur as Eu2+ and Eu3+, and U4+ and U6+, respectively, which, like other REEs, replaced Ca2+ in the dodecahedral site (Smith et al. Reference Smith, Henderson, Jeffries, Long and Williams2004). The relationships between total REE and Eu anomalies and U concentrations in garnet can therefore be used to discriminate redox conditions of hydrothermal fluid (Zhai et al. Reference Zhai, Liu, Zhang, Wang, Su, Yang and Wu2014; Zhang et al. Reference Zhang, Liu, Shao and Li2017 a, b; Tian et al. Reference Tian, Leng, Zhang, Zafar, Zhang, Hong and Lai2019).
The analytical results of our study show a generally positive correlation between δEu and the total REE (Fig. 10a). As a result of similar geochemical properties of Eu2+ and Ca2+, it is easy for Eu2+ to enter the Ca-site relative to Eu3+ (Van Westrenen et al. Reference Van Westrenen, Allan, Blundy, Purto and Wood2000). Eu anomalies of garnets are therefore determined by the Eu2+/Eu3+ ratios of mineralizing fluids. The differences in total and Eu anomalies of three-generation garnets reflect fluid redox fluctuation. From Grt1 to Grt2, the increase in oxygen fugacity of fluids reduced Eu2+/Eu3+ ratios, which produces a significant Eu depletion and low total REE in Grt2 relative to Grt1 (Fig. 10a). This possibility is further indicated by the relationship between total REE and U contents. Total REE and U concentrations in different generations of garnet were also plotted in distinct fields (Fig. 10b, c), suggesting that U and total REE concentrations in garnets gradually decrease from Grt1 to Grt2. Meanwhile, U4+ relative to U6+ is preferentially incorporated into Ca2+ in garnet based on ionic radius (Smith et al. Reference Smith, Henderson, Jeffries, Long and Williams2004). The U variations in different generations of garnet are therefore indicative of an increasing trend in fO2 of fluid during garnet formation. Following Grt1-A formation, the skarn fluid system shifted into oxidizing conditions with a relatively higher fO2 value. The increasing fO2 value of the fluid could increase U solubility and, in turn, decrease U incorporation into Grt1-B and Grt2.

Fig. 10. Scatter variation diagrams of (a) δEu versus ΣREE; (b) U versus ΣREE; (c) δEu versus Fe3+(apfu); (d) Mg2+(apfu) versus SnO2; (e) Fe2+(apfu) versus SnO2; and (f) SnO2 versus Fe3+(apfu) of all garnet types. The circle and triangle in the grey fields are after Zhou et al. (Reference Zhou, Feng and Li2017) and Ding et al. (Reference Ding, Ma, Lu and Zhang2018), respectively.
6.b.2. Implications for Fe–Zn mineralization
Fluid redox conditions are important to reveal the mineralization of metal elements (e.g. W, Sn, Fe and Zn) in ore-forming fluids. In this study, three types of metallogenic elements include W–Sn, Fe-related metal elements (e.g. Sc, V, Co and Ti) and Zn, which are used to discuss their variation characteristics. The incorporation of W–Sn into the structure of garnet is generally closely related to oxidizing fluids, which is based on the positive relationship between W, Sn and andradite contents (Park et al. Reference Park, Choi, Kim, Park, Kang, Lee and Song2017 a; Zhou et al. Reference Zhou, Feng and Li2017; Ding et al. Reference Ding, Ma, Lu and Zhang2018). In other words, the incorporation of W and Sn is related to Fe3+ content in fluid, which follows the substitution of Sn4+ + Mg2+ or Fe2+ = 2Fe3+ (Novak & Gibbs, Reference Novak and Gibbs1971; Feneyrol, Reference Feneyrol2012).
The analytical data of garnets from the Luoyang deposit indicate the positive Sn versus Mg (Fig. 10d) and negative Sn versus Fe2+ correlations (Fig. 10e), suggesting the Sn substitution mechanism of Sn4+ + Mg2+ = 2Fe3+. The existing data (e.g. Park et al. Reference Park, Choi, Kim, Park, Kang, Lee and Song2017 a; Zhou et al. Reference Zhou, Feng and Li2017; Ding et al. Reference Ding, Ma, Lu and Zhang2018) suggest that the high oxygen fugacity favours the incorporation of Sn into garnet and Sn-rich garnets are nearly pure andradite. Overall, the studied garnets belong to andradite-rich garnet, which is in favour of incorporation of Sn. However, nearly pure andradite Grt2, which forms under relatively high oxidizing conditions, shows abnormal low SnO2 contents (Fig. 10f). It is possible that, from Grt1 to Grt2, the sudden drop of Sn in hydrothermal fluid caused precipitation of Grt2 with low Sn concentrations. As for W, and as for the correlation of W and andradite contents in most W–Sn skarn deposits (Fig. 11a), the three generations of garnets yield a positive correlation, suggesting that the enrichment of W in garnet was closely linked to a Fe3+-rich fluid with high oxygen fugacity. We therefore inferred that Sn enrichment is related to Grt1 formation during relatively low fO2 conditions, whereas W enrichment is related to Grt2 formation during relatively high fO2 condition. It is difficult to evaluate Fe element changes based on major elements (e.g. Fe), but can be reflected by Fe-related trace elements (e.g. Sc, V, Co and Ti). The reduced Sc, V, Co and Ti contents from Grt1 to Grt2 (Fig. 11b–e) indicate that the iron in the fluid decreases gradually, which probably causes Fe precipitation. The ore-forming fluid shifted to a relatively high fO2 condition during the formation of Grt1-B. This condition favours the precipitation of iron in the ore-forming fluid, which is consistent with the fact that Grt1-B coexists with magnetite (Fig. 6e). Another ore-forming element Zn also showed prominent variation characteristics, with the highest Zn contents in Grt1-B and the lowest in Grt2, which may promote the precipitation of zinc. This is documented by the coexistence of sphalerite and Grt2 (Fig. 6a–d).
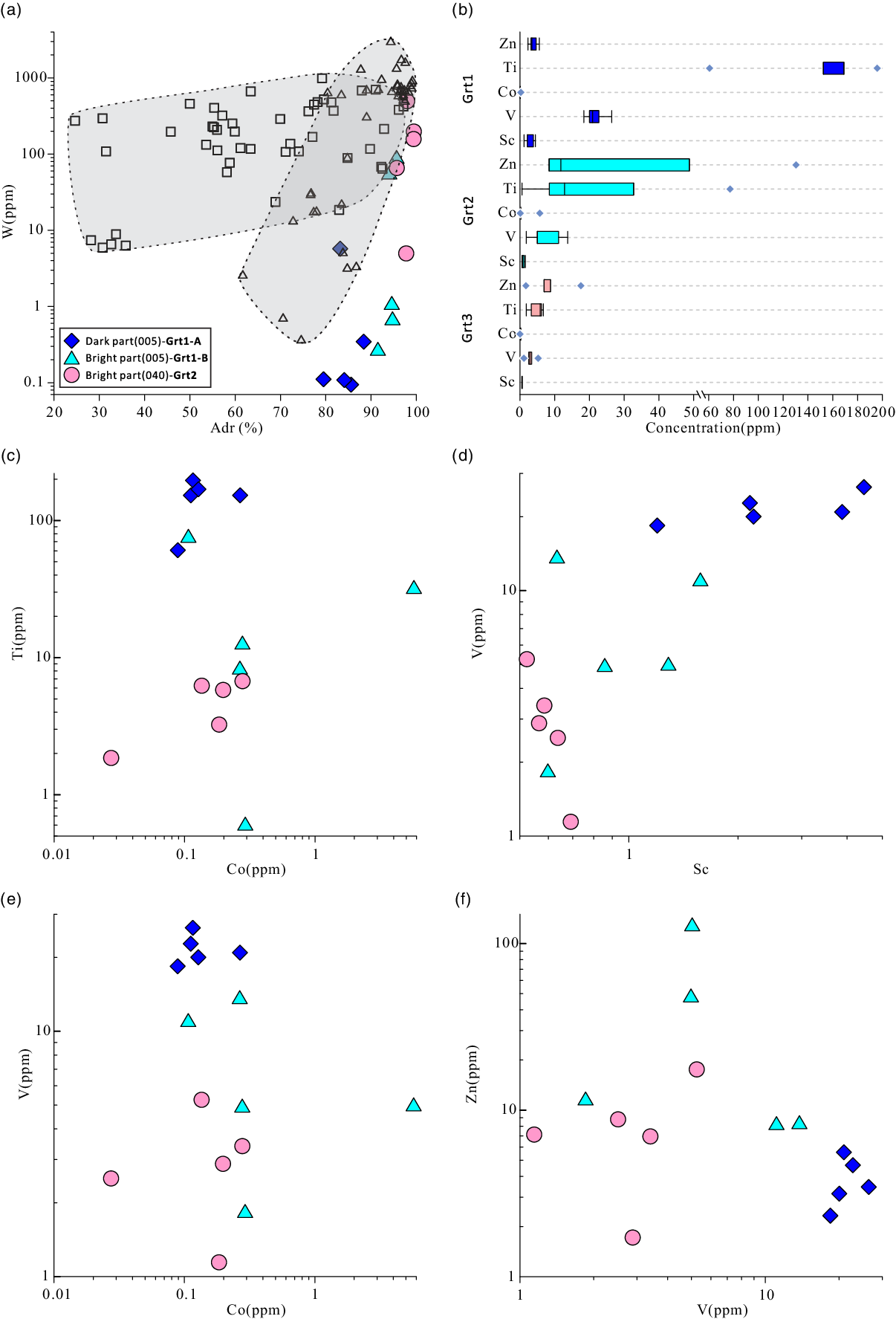
Fig. 11. (a) W versus Adr diagram for all garnets from the Luoyang deposit; and (b–f) scatter variation diagrams of siderophile element compositions in three generations of garnets from the Luoyang deposit. The square and triangle in the grey fields are after Park et al. (Reference Park, Song, Kang, Shim, Chung and Park2017b) and Ding et al. (Reference Ding, Ma, Lu and Zhang2018), respectively.
6.c. Origin and evolution of hydrothermal fluid
The interrelation of HFSEs, total REE, Y and Ho in hydrothermal fluid may reflect the original and the developed fluid or the influence of an external fluid (Jamtveit et al. Reference Jamtveit, Wogelius and Fraser1993). The decreasing of HFSEs, total REE and Y content in fluid indicates the increasing water/rock ratios or greater amounts of external fluid incursion (Green et al. Reference Green, Blundy and Adam2000; Gaspar et al. Reference Gaspar, Knaack, Meinert and Moretti2008). Moreover, the possibility of different sources of fluids is addressed using the diagrams of Y versus Ho and total REE. Generally, magmatic fluids have Y/Ho ratios close to the chondrite value (Bau, Reference Bau1996), and their Y and total REE contents exhibit a linear relationship (Park et al. Reference Park, Song, Kang, Shim, Chung and Park2017 b). Instead, if their Y/Ho ratios deviate from the chondritic line and there is no linear relationship between Y and total REE, it is possible that another type of fluid (e.g. meteoric water) has entered the skarn system.
Studies have shown that zonation patterns of skarn garnets record the transformation from more diffusive metasomatism responsible for Al-rich garnet formation with low water/rock ratios to more infiltrative metasomatism corresponding to Fe-rich garnet formation with high water/rock ratios (Jamtveit et al. Reference Jamtveit, Wogelius and Fraser1993; Meinert et al. Reference Meinert, Dipple and Nicolescu2005; Gaspar et al. Reference Gaspar, Knaack, Meinert and Moretti2008; Park et al. Reference Park, Song, Kang, Shim, Chung and Park2017 b). The andradite contents of garnets observed in the Luoyang deposit increase from Grt1 to Grt2 (Fig. 7), whereas this variation range is small relative to other Fe skarn deposits (Fig. 7) and mainly Fe-enriched andradite without observation of Al-rich garnet. Moreover, the occurrence of infiltration-controlled growth textures, such as epitaxial overgrowth, trapezohedral {211} crystal faces, oscillatory zoning and dissolved zonation, imply that Luoyang garnets formed from rapid growth with high water/rock ratios and were produced by infiltrative metasomatism of externally derived fluids (Gaspar et al. Reference Gaspar, Knaack, Meinert and Moretti2008). Furthermore, the similar positive relations among the various elements (Fig. 12), such as ΣREE, HFSE, Y and Ho, and the consistent chondritic Y/Ho ratio with granite (Fig. 12a) in the Luoyang deposit, suggest that the externally derived fluids for all garnet formations were derived from magmatic–hydrothermal fluid without undergoing differentiation due to the precipitation of garnet. The obviously low HFSE content of garnet relative to granite (Fig. 12b, c) is probably caused by phase separation from magmatic to hydrothermal process (Green et al. Reference Green, Blundy and Adam2000). The variation diagram of ΣREE versus Y (Fig. 12d) indicates that all garnets crystallized in a relatively closed condition compared with those in an extremely opened-system condition, shifting from linear correlation (Park et al. Reference Park, Song, Kang, Shim, Chung and Park2017 b).

Fig. 12. Diagrams of (a) Ho versus Y; (b) HFSE versus ΣREE; (c) HFSE versus Y; and (d) Y versus ΣREE in all garnet types. Data for the Luoyang granite porphyry are from Yang et al. (Reference Yang, Ni, Wu, Ding and Zhu2017); chondrite line is from Anders & Grevesse (Reference Anders and Grevesse1989), and skarn W deposit included for comparison is from Park et al. (Reference Park, Song, Kang, Shim, Chung and Park2017b).
7. Conclusions
-
(1) Two generations of garnets were identified in the Luoyang deposit based on field and optical characteristics. Grt1 occurs as a coarse-grained crystal replaced by massive diopside–magnetite assemblage, whereas Grt2 is characterized by fine-grained crystal that replaced massive magnetite together with sphalerite and exhibits unzoned patterns. Grt1 can be further classified into two types, Grt1-A and Grt1-B, based on BSE images. Grt1-A shows clear oscillatory zonings and Grt1-B occurs as irregular zones coexisting with abundant small magnetite grains. Luoyang garnets are dominated by Fe-enriched andradite and belong to the grossular–andradite solid solution series.
-
(2) Grt1-A exhibits LREE-enriched to weak HREE-enriched patterns with convex curves and weak negative to positive Eu anomalies. Grt1-B has a moderately downwards-sloping (LREE-enriched and HREE-depleted) trend with weak negative to positive Eu anomalies. Grt2, as for Grt1-B, shows a flat REE pattern with pronounced negative Eu anomalies. The incorporation of REEs into the three generations of garnets was likely controlled by the substitution mechanism of [X2+]VIII –1[REE3+]VIII +1[Si4+]IV –1[Z3+]IV +1 in an Al-enriched environment.
-
(3) Grt1-A has high U, ΣREE and δEu, indicative of fluid with a low fO2, and Grt1-B and Grt2 are depleted in U, ΣREE and low δEu, suggesting fluid with a high fO2. Grt1-A is enriched in Sn and depleted in W, whereas Grt2 is significantly enriched in W and depleted in Sn. The Sn and W enrichments can be attributed to a fluid with a low and a high fO2, respectively. The decreasing contents of siderophile elements in Grt1-A are likely to have been provided to the late magnetite mineralization. The increased fO2 in fluid during the formation of Grt1-B causes the deposition of magnetite, and the sphalerite deposition is likely related to reduced contents of Zn from Grt1-B to Grt2.
-
(4) The ore-forming fluids, which formed all garnet types, were derived from magmatic fluid. All garnets crystallized in an opened-system condition controlled by infiltrative metasomatism.
Acknowledgements
We are greatly indebted to Senior Engineer Qi-Rui Li (Fujian Luoyang Mine) for assistance during fieldwork. This research was supported by the opening funding of State Key Laboratory for Mineral Deposits Research, Nanjing University (grant no. 2019-LAMD-K08), the research initiation funds of Chengdu University of Technology (grant nos 10912-2018KYQD-06881 and 10912-2019KYQD-06881) and Hunan Key Laboratory of Land Resources Evaluation and Utilization (Grant No. SYS-ZX-201901).
Supplementary material
To view supplementary material for this article, please visit https://doi.org/10.1017/S0016756821000431