1. Introduction
Albeit rare on Earth, marine meteorite impacts can affect diversity and ecological configuration of the living biota on a local to global scale. Such events, at least in the case of large-scale ones, generally are associated with extinctions in the Phanerozoic record, and their effects on the contemporary ecosystems are thus believed to have been negative to devastating (e.g. Alvarez et al. Reference Alvarez, Alvarez, Asaro and Michel1980). Therefore a great deal of earlier work has dealt with and discussed the patterns, rates and range of causes of mass extinctions, whereas considerably less attention has been paid to the post-extinction faunal rebound (Erwin, Reference Erwin2001), and detailed information on, for example, the patterns of faunal recovery in and around marine craters is scarce. However, impacts are also known to create more varied environments with new ecological niches for various organisms to fill and blooming of disaster taxa (Cockell & Bland, Reference Cockell and Bland2005; Smelror & Dypvik, Reference Smelror, Dypvik, Cockell, Koeberl and Gilmour2006). Within recently formed impact craters, a variety of habitats are accessible for immigration, generating a distinct post-impact succession of primary colonizers and variable phases of succeeding colonizers (Cockell, Osinski & Lee, Reference Cockell, Osinski and Lee2003). Along those lines a thought-provoking hypothesis was recently put forward by Schmitz et al. (Reference Schmitz, Harper, Peucker-Ehrenbrink, Stouge, Alwmark, Cronholm, Bergström, Tassinari and Xiaofeng2008), namely that increased bombardment of extraterrestrial matter on Earth, during the Middle Ordovician, actually triggered evolution and accelerated the biodiversification process usually referred to as the Great Ordovician Biodiversification Event.
During the Ordovician Period the Baltic basin, a sediment-starved temperate-water carbonate basin, was bombarded by bolides, one of which resulted in the 2 km wide Tvären crater in southeastern Sweden. Because of the discovery of the crater, and subsequent drillings resulting in the Tvären-2 drill core, investigations of the post-impact sedimentary infillings and faunal dynamics commenced (Lindström et al. Reference Lindström, Flodén, Grahn and Kathol1994). This impact in a shallow marine area resulted in resurging sea water, followed by settling of ejecta and resurge materials prior to the continued deposition of carbonates forming the Upper Ordovician (Caradocian, lower Sandbian) Dalby Limestone (Lindström et al. Reference Lindström, Flodén, Puura and Suuroja1992, Reference Lindström, Flodén, Grahn and Kathol1994; Ormö, Reference Ormö1994; Wallin & Hagenfeldt, Reference Wallin and Hagenfeldt1996; Ormö, Sturkell & Lindström, Reference Ormö, Sturkell and Lindström2007; Frisk & Ormö, Reference Frisk and Ormö2007). The confined marine ecospace formed within the crater represents various environments, ranging from the deep central depression towards the shallower environments at the crater wall and rim (Lindström et al. Reference Lindström, Flodén, Grahn and Kathol1994; Frisk & Ormö, Reference Frisk and Ormö2007). As the crater progressively filled with sediments, the crater depression was reduced in depth and probably transformed successively into less restricted environmental conditions for the residing biota. The sediment-trapping crater structure has resulted in the preservation of a more refined and complete post-impact sedimentary succession in the Tvären Bay, where sediments four times the thickness of the average coeval basinal succession accumulated.
As investigations on the biota of the Tvären-2 drill core advanced, it was discovered that scolecodonts, or polychaete jaws, are one of the most abundantly occurring fossil groups throughout the core, which inspired this study. In modern oceans, polychaetes comprise one of the most abundant and diverse invertebrate clades, inhabiting all types of environments from harbours through coral reefs and estuaries to the bathyal zone. Although their fossil record is patchy and not known in great detail, the most common fossil remains of these animals, the scolecodonts, bear witness to their success in exploiting different niches of the marine realm already in the early Palaeozoic. From at least the Darriwilian (Middle Ordovician) and onward, jaw-bearing polychaetes diversified significantly (Hints & Eriksson, Reference Hints and Eriksson2007a).
This paper aims at investigating the polychaete faunas succeeding an extraterrestrial impact that occurred in the midst of their radiation. In the Tvären crater we are focusing on the post-impact Dalby Limestone, from the drill core and erratic boulders and its previously unknown faunas of scolecodont-bearing polychaetes. In order to understand the faunal dynamics in this variable environment, we have analysed the taxonomic interactions, primarily at the genus level, during immigration of the crater and the subsequent temporal development of the fauna. Moreover, their implications as to the ecological complexity of the impact crater are considered.
2. Geological setting and stratigraphy
As a result of a marine bolide impact forming a crater in the Tvären Bay (Fig. 1), the area has a well-preserved sequence of Upper Ordovician sediments that otherwise would have been eroded away. Flodén, Tunander & Wickman (Reference Flodén, Tunander and Wickman1986) conducted geophysical investigations in the bay that led Wickman (Reference Wickman, Bodén and Eriksson1988) to interpret the structure as an impact crater. It is located in a region now dominated by Precambrian crystalline rocks and comprises an approximately 2 km wide, partially sediment-filled circular depression (Fig. 2). It has for long periods of time been exposed to subaerial, and lately also glacial, erosion but is at present submerged on the Swedish east coast. The target sequence consists of Ordovician carbonates resting on non-lithified sands of early to earliest middle Cambrian age covering a crystalline basement.

Figure 1. Map showing the location of the Tvären crater in southeastern Sweden and the position of the two drill cores made in 1991 through the structure. The fan of glacial erratics (also sampled for this study) of the Dalby Limestone is marked by triangles. Map modified from Lindström et al. (Reference Lindström, Flodén, Grahn and Kathol1994).

Figure 2. Cross-section of the Tvären crater based on seismic profiling and drillings (modified from Lindström et al. Reference Lindström, Flodén, Grahn and Kathol1994) and proposed rim heights (Ormö, Sturkell & Lindström, Reference Ormö, Sturkell and Lindström2007). The main depositional environments are located on the crater rim and in the central depression. Infilling of the crater was probably completed but due to subsequent erosion the crater rim and upper central depression deposits are not preserved.
Drilling in the structure in 1991 resulted in two cores: the Tvären-1 drilling instantly struck the Precambrian crystalline basement, whereas the Tvären-2 drill core yielded an approximately 140 m thick sedimentary sequence through the crater (Figs 2, 3; Lindström et al. Reference Lindström, Flodén, Puura and Suuroja1992, Reference Lindström, Flodén, Grahn and Kathol1994). Post-impact infill strata are also accessible in glacial erratic boulders from the region (Fig. 1).

Figure 3. Lithological succession of the Tvären-2 drill core with distribution of structures and fossil elements (ranges based on first through last occurrence) in the post-impact sediments. Based on Lindström et al. (Reference Lindström, Flodén, Grahn and Kathol1994), Wallin & Hagenfeldt (Reference Wallin and Hagenfeldt1996), Grahn, Nõlvak & Paris (Reference Grahn, Nõlvak and Paris1996), and data herein. The range of conodonts within the Dalby Limestone is based on unpublished data, courtesy of ÅMF.
Shocked quartz has been found in the sandy deposits of the graded resurge deposits, confirming that the structure is the result of a bolide impact (Lindström et al. Reference Lindström, Flodén, Grahn and Kathol1994). The Dalby Limestone deposition occurred both before and after the impact event, however, the pre-impact sediments are merely preserved in the resurge deposits. Post-impact Dalby sediments overlie the resurge deposits with a gradual transition. The marine impact occurred at a depth estimated to 100–150 m (Ormö, Sturkell & Lindström, Reference Ormö, Sturkell and Lindström2007; Frisk & Ormö, Reference Frisk and Ormö2007).
2.a. The Tvären-2 drill-core
The Tvären-2 drilling reached a depth of 224.4 m. The entire, 140 m thick, sedimentary sequence consists of a crystalline basement breccia (224.4–219.6 m), overlain by roughly 60 m of sandy to silty, fining-upwards resurge deposits of limestone breccia (219.6–199.05 m), followed by sand and siltstones (199.05–161.4 m). No internal lithological boundaries have been determined in the sequence between 219.6 and 161.4 m, most likely indicating a single turbidite-like fining-upwards sequence (Lindström et al. Reference Lindström, Flodén, Grahn and Kathol1994). Subsequently, these sediments were succeeded by approximately 80 m (the 161.4–82.15 m interval) of secular sediments of mixed mud and carbonate rocks primarily representing the Dalby Limestone (Fig. 3; Lindström et al. Reference Lindström, Flodén, Grahn and Kathol1994; Ormö, Sturkell & Lindström, Reference Ormö, Sturkell and Lindström2007). The first fossiliferous deposits of medium grey mudstone with coarse sand (161.4–149.0 m) represent a combination of resurge sediments and the initial Dalby Limestone. It is poorly sorted and contains some chitinozoans and a few graptolites as the only fossils. Thus, because sedimentation was still affected by resurge, the fossil content of this unit is partly reworked and, as a result, less applicable for biostratigraphy. Based on lithological and palaeontological data, a boundary between the resurge deposits and the ‘normal’ Dalby Limestone is visible at about 149 m depth (Lindström et al. Reference Lindström, Flodén, Grahn and Kathol1994). Recent geochemical results (Frisk & Ormö, Reference Frisk and Ormö2007; Ormö et al. Reference Ormö, Hill, Self-Trail and Frisk2009) confirm that estimated boundary level.
The initiating muddy and clayey post-impact Dalby sedimentation slowly and gradually changed to become more calcareous (Lindström et al. Reference Lindström, Flodén, Grahn and Kathol1994; Frisk & Ormö, Reference Frisk and Ormö2007), and higher up in the stratigraphy the calcareous mudstones are intercalated more often with distinct fossiliferous calcarenites. This increase in biocalcarenite beds is shown by the evidence of peaks in inorganic carbon (IC) content, coupled with distinct drops in organic carbon (Corg) content (Frisk & Ormö, Reference Frisk and Ormö2007). The high IC values and low Corg values of all the calcarenitic turbidites from the Tvären-2 core correspond very well with samples of the Ringsön limestone erratics, supporting a similar origin in relation to the crater (Lindström et al. Reference Lindström, Flodén, Grahn and Kathol1994; Frisk & Ormö, Reference Frisk and Ormö2007). In addition to the geochemical support, the turbidites show a noticeable difference in fauna and lithology as compared to the enclosing strata, revealing their origin as debris flows from a facies developed on the crater rim. Rhynchonelliformean brachiopods, bryozoans, echinoderms and trilobites characterize the general fossil assemblage of the post-impact secular strata of the rim facies. The turbidites in the core are characterized by an identical biota (Lindström et al. Reference Lindström, Flodén, Grahn and Kathol1994; Frisk & Ormö, Reference Frisk and Ormö2007; but see also below), further linking the introduced material to the same source as the erratics. Calcarenitic turbidites are found early in the succession above the lowermost part of the Dalby Limestone boundary at 149 m, suggesting that the development of the shallow water environment on the crystalline crater rim was initiated shortly after the resurged sediments settled (Lindström et al. Reference Lindström, Flodén, Grahn and Kathol1994; Frisk & Ormö, Reference Frisk and Ormö2007).
The studies on conodonts by Ormö (Reference Ormö1994) and chitinozoans by Grahn, Nõlvak & Paris (Reference Grahn, Nõlvak and Paris1996), from the Tvären-2 core sequence, have dated the impact to the Late Kukruse Age; the latter also indicate that infilling of the structure was completed prior to the end of the Kukruse (Fig. 4). The post-impact sequence of the Dalby Limestone biostratigraphically belongs to the Laufeldochitina stentor chitinozoan Zone (Lindström et al. Reference Lindström, Flodén, Grahn and Kathol1994; Grahn, Nõlvak & Paris, Reference Grahn, Nõlvak and Paris1996) and at least its lower part represents the Baltoniodus variabilis conodont Subzone of the Amorphognathus tvaerensis conodont Zone (Lindström et al. Reference Lindström, Flodén, Grahn and Kathol1994; Ormö, Reference Ormö1994). Conodonts of the upper part of the drill core remain unstudied. The chitinozoan Conochitina tigrina was recorded in the uppermost beds of the core, which correspond to the topmost Kukruse Stage (Grahn, Nõlvak & Paris, Reference Grahn, Nõlvak and Paris1996).
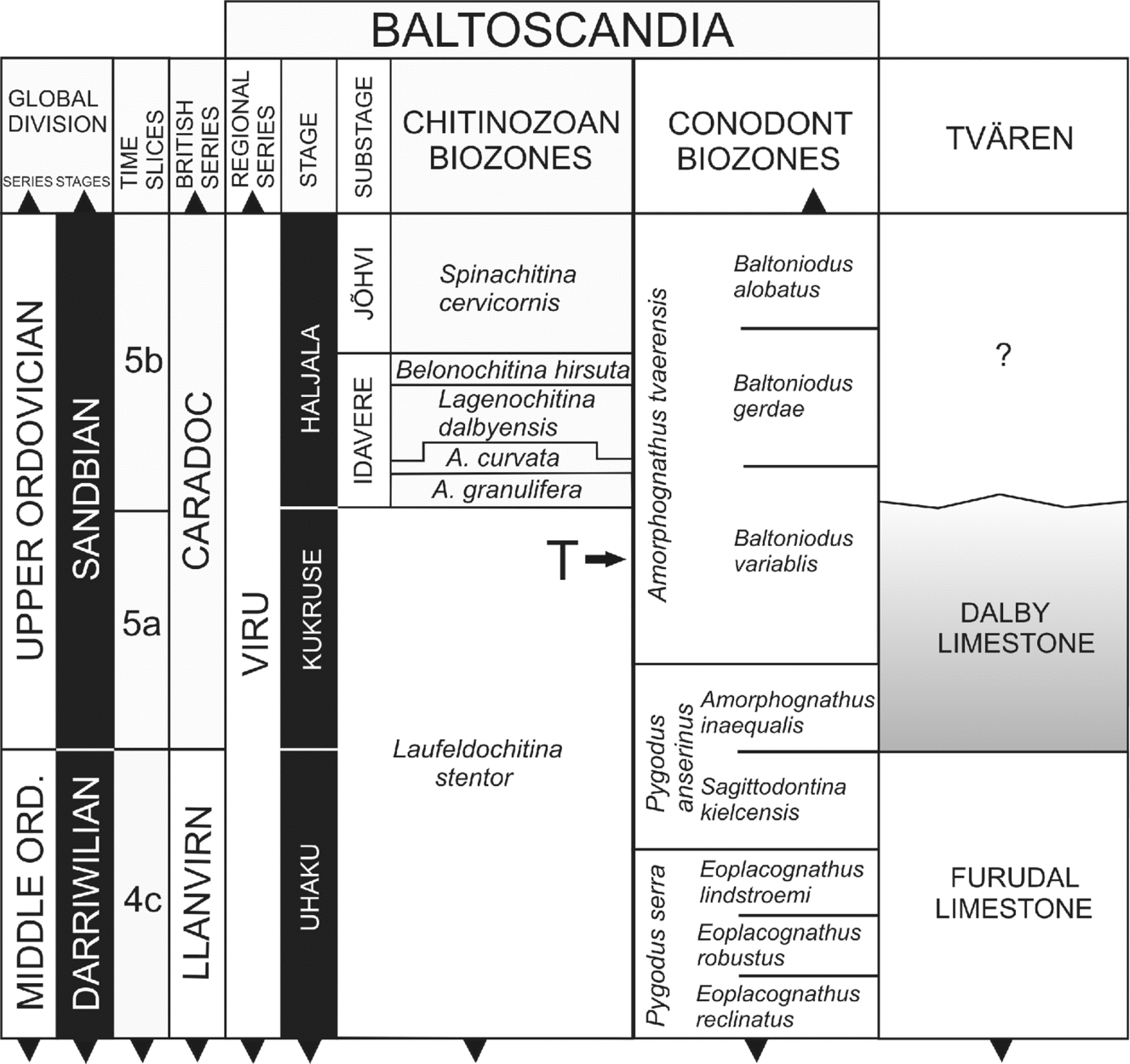
Figure 4. Stratigraphic chart showing parts of the Upper Ordovician and the chronostratigraphical and biostratigraphical age of the Dalby Limestone of the Tvären district (based on the Tvären-2 drill core and the erratic boulders of Ringsön island). T indicates the time of impact at Tvären. Figure modified from Frisk & Ormö (Reference Frisk and Ebbestad2007) and Ebbestad & Högström (Reference Ebbestad, Högström, Ebbestad, Wickström and Högström2007) and references therein.
2.b. The erratic limestone boulders
In a limited area south of the Tvären Bay, fossiliferous erratics of Ordovician age are locally fairly abundant (Fig. 1). As mentioned by Thorslund (Reference Thorslund1940), the first boulders were discovered and sampled by B. Asklund and A. H. Westergård during geological investigations on the island of Ringsön in 1927. Based on detailed studies it was concluded that the erratics were derived from solid beds in the bay and were transported southeastwards by ice from the mainland (Thorslund, Reference Thorslund1940; Bergström, Reference Bergström1962; Lindström et al. Reference Lindström, Flodén, Grahn and Kathol1994). Three different kinds of lithologies of these erratic boulders have been described from the Tvären Bay area: limestone, calcareous sandstone and breccia (Thorslund, Reference Thorslund1940; Bergström, Reference Bergström1962). These previously known lithologies can be correlated to the Tvären crater succession of the Tvären-2 drill core as these three lithologies are present in the drill core strata. Lithologically, the studied limestone boulders are brownish-grey, coarse bioclastic grainstone and occasionally moderately silicified (Bergström, Reference Bergström1962; ÅMF, pers. obs.). Coarse and angular crystalline clasts have been found in the boulders; in all probability they originate from the breccia containing the underlying pre-impact bedrock substrate. From the Lockne crater, with a comparable rim facies, Frisk & Ormö (Reference Frisk and Ebbestad2007) reported the presence of crystalline fragments that were transported from the adjacent unconsolidated sediments of the breccia due to irregularities in the topography.
The limestone boulders examined are abundant in fossils, and this content has been examined by several authors, particularly with regards to trilobites (Thorslund, Reference Thorslund1940; Jaanusson, Reference Jaanusson1957a), ostracods (Thorslund, Reference Thorslund1940; Jaanusson, Reference Jaanusson1957b), graptolites (Strachan, Reference Strachan1959), conodonts (Bergström, Reference Bergström1962; Ormö, Reference Ormö1994), gastropods (Frisk & Ebbestad, Reference Frisk and Ebbestad2007) and brachiopods (material in preparation by ÅMF). Graptolites of the Nemagraptus gracilis and Mesograptus foliaceus zones and conodonts suggest that the erratics belong to the Upper Ordovician Dalby Limestone (Strachan, Reference Strachan1959; Bergström, Reference Bergström1962).
3. Materials and Methods
The Tvären-2 drill core has been repeatedly sampled for petrography, geochemistry and palaeontology (e.g. Grahn & Nõlvak, Reference Grahn and Nõlvak1993; Lindström et al. Reference Lindström, Flodén, Grahn and Kathol1994; Ormö, Reference Ormö1994; Grahn, Nõlvak & Paris, Reference Grahn, Nõlvak and Paris1996; Wallin & Hagenfeldt, Reference Wallin and Hagenfeldt1996; Frisk & Ormö, Reference Frisk and Ormö2007; Ormö, Sturkell & Lindström, Reference Ormö, Sturkell and Lindström2007). For this study, 15 samples (with prefix TT followed by digits) were collected from the interval 149.0–82.15 m (Figs 5, 6) and processed for scolecodonts and conodonts; the latter fossils will be described elsewhere. High-resolution sampling was carried out in the lower part of the core in order to investigate any short-term effects related to the impact. Each sample level consists of 8–14 cm of core, depending on lithology. The core has a diameter of 4 cm, and only half of the core was used, so it still can be accessible for supplementary analyses. The total weight of the samples varied between 63.23 and 208.08 g (Fig. 6). In addition to these core samples, a number of glacial erratics from Ringsön island were processed (sample TTERR; Fig. 6). Those erratics were collected by B. Asklund and P. Thorslund in the 1930s and have since been stored at the Museum of Evolution in Uppsala (UPM). All samples were digested in 7 % acetic acid, washed and electrostatically hand-picked in dry conditions down to 63 μm. The sample residues tended to yield small aggregates and many of the scolecodonts recovered have adhering matrix.

Figure 5. Distribution of recorded polychaete taxa in the post-impact Dalby Limestone succession of the Tvären-2 drill core and the Ringsön erratics (TTERR). For lithological key, see Figure 3. An open circle indicates uncertain identification. Note that Lunoprionella may include more than one species. Pie charts show relative frequency (%) of the most common genera in selected samples.

Figure 6. Sample data showing sample level (depth in m for the Tvären-2 drill core), weight of unprocessed microfossil sample (when available), lithology, number of first maxillae (MI) recorded, fossils identified on bedding plane surfaces and/or from the acid-resistant residues from the corresponding sample level of the drill core and in the erratics, and the relative conodont abundance calculated from the digested samples (high > 100; medium ~ 50; low < 20 specimens counted in a sample). Note that precise sample weight for the ÅW samples is unknown (approximately 50 g each used in Wallin & Hagenfeldt, Reference Wallin and Hagenfeldt1996). Fossil abbreviations: a – asaphid; b – bryozoan; c – cephalopod; ch – chitinozoan; con – conodont; cr – craniform; e – echinoderm; g – graptolite; l – linguliform; o – ostracod; r – remopleuridid; rb – rhynconelliformean brachiopod; t – trilobite.
An additional set of sample residues was handed to us by Åsa Wallin, Stockholm University (see Figs 5, 6). These were core samples, digested using hydrofluoric (HF) acid, from Wallin & Hagenfeldt's (Reference Wallin and Hagenfeldt1996) work on acritarchs and included in this study (samples with prefix ÅW followed by digits indicating the sample level). The scolecodonts occurring in these residues generally are very well cleaned from adhering matrix, reddish-brown in colour and semi-transparent, as opposed to those recovered through acetic acid digestion, most of which are jet-black. The HF-treated samples, moreover, contained a larger number of small specimens that in many cases do not have very well-developed characters, which makes them difficult to identify unambiguously. These differences to some degree complicate direct comparisons between the different sets of samples.
Altogether, more than 2000 scolecodonts and partially articulated jaw apparatuses were recovered. Taxonomic identification was complicated because a large number of specimens are strongly deformed and/or fragmented, obscuring original morphology and species characteristics. For this reason, several specimens could only be determined to genus level with certainty and many had to be left under open nomenclature.
Counting of specimens, for relative frequency (%) and multivariate analyses, was based on the first maxillae (MI), unless stated otherwise. It should be noted that the counting of ‘Xanioprion’ was difficult since its jaws disarticulate easily along the fragile and prolonged denticle boundaries. Therefore only relatively large and complete-looking specimens were counted. Moreover, because the jaw apparatus architecture of this taxon is not known, the counts might include other elements in addition to the MI.
The PAST software package of Hammer, Harper & Ryan (Reference Hammer, Harper and Ryan2001) was used to perform a Paired Group, Brey Curtis (suitable for smaller samples) cluster analysis. A rarefaction curve was created which made us omit samples with less than 20 specimens recorded. Because of the differences noted above between the different sets of samples, only the TT samples were included in this analysis.
All figured specimens are housed at the Department of Geology, Lund University (numbers with prefix LO, for Lund Original). The Tvären-2 drill core is stored at the Department of Geology and Geochemistry, Stockholm University.
4. Post-impact biota of the crater environments
The post-impact event Dalby Limestone succession of the Tvären-2 core has yielded diverse fossil assemblages, including planktonic and nektonic organisms as well as sessile and mobile benthos (Figs 3, 6). The fossils identified include graptolites, conodonts, chitinozoans, scolecodonts, acritarchs, trilobites (asaphids and remopleuridids), ostracods, echinoderms, bryozoans and brachiopods (strophomenoids and various linguliform brachiopods) (Lindström et al. Reference Lindström, Flodén, Grahn and Kathol1994; Grahn, Nõlvak & Paris, Reference Grahn, Nõlvak and Paris1996; Wallin & Hagenfeldt, Reference Wallin and Hagenfeldt1996; data herein). Lindström et al. (Reference Lindström, Flodén, Grahn and Kathol1994) suggested that as the crater successively filled, the post-impact region had a depth-controlled palaeoecology for a considerable period of time in which successions of various metazoan groups followed. They moreover subdivided the faunal content of the post-impact sediments (above 149.0 m depth) as representative of three different environments: (1) the deepest part of the crater, (2) the shallowest part (including particularly the rim and crater wall fauna) and (3) the regional water column.
Asaphid trilobites, commonly Neoasaphus ludibundus, are present in the lower portion of the Dalby Limestone, with the first specimens appearing a couple of metres above the base and then occurring continuously throughout the core (Fig. 3). Abundant, but irregularly distributed and flattened, specimens of Echinosphaerites are established early on in the deeper settings, and also later in less deep environments as the crater becomes shallower. Scolecodonts belong to the first non-planktonic groups to enter the crater floor. Small brachiopods, such as acrotretoids and lingulids, are common. Remopleuridids, ostracods and the rhynchonelliformean brachiopod Sericoidea all appear on the crater floor, but higher up in the sedimentary sequence than asaphid trilobites and scolecodonts, indicating that the environment was not habitable enough or that they did not only prefer deeper water as they have been attributed to, but also shallower water environments (Lindström et al. Reference Lindström, Flodén, Grahn and Kathol1994).
The shallower water assemblages of the rim facies primarily comprise rhynchonelliformean brachiopods, bryozoans, crinoid stems and ostracods. Trilobites, graptolites, hyoliths, craniids and gastropods are present but less common. In terms of microfossils, conodonts, scolecodonts, chitinozoans, acrotretoids, lingulids and spicules of sponges are particularly common.
Chitinozoans and graptolites are the first faunal elements of the open shelf sea to enter the regional water column in the crater interior, appearing already below the lower boundary of the Dalby Limestone at 149 m.
The conodont abundance was estimated from the TT samples (Fig. 6). In the upper third of the succession, conodonts are considerably more abundant than scolecodonts, whereas the latter are more abundant in the lower parts. As for the erratics, conodonts seem slightly more abundant than scolecodonts.
5. The Tvären polychaetes
The Upper Ordovician Tvären scolecodonts, just like most Palaeozoic ones, belong to jawed polychaetes of the order Eunicida. These are characterized by having a jaw apparatus generally composed of two massive, ventral mandibles (Md) and a complex, dorsal, multi-element apparatus. The latter comprises a number of hollow maxillae (M) that may be associated with complementary elements, such as the posteriormost carriers (Cr), basal plate (Bp), intercalary teeth (It) and lateral teeth (Lt). The maxillae are numbered in roman numerals from the posterior first maxilla (MI) to the anterior ones (commonly MV), and referred to as occupying the left or right hand side of the apparatus. Because very few jaw apparatuses have been recorded that comprise the mandibles found together with the dorsal maxillary apparatus, our taxonomic knowledge of the former is relatively poor. Depending on the architecture of the jaw apparatuses, they are commonly referred to as one of five different types: labidognath, placognath, ctenognath, xenognath and prionognath, representing different grades of evolution. For further information and reviews on jaw apparatus architecture, see Kielan-Jaworowska (Reference Kielan-Jaworowska1966), Szaniawski (Reference Szaniawski, Jansonius and McGregor1996) and Eriksson, Bergman & Jeppsson (Reference Eriksson, Bergman and Jeppsson2004).
The post-impact Dalby Limestone strata of the Tvären Bay yielded an abundant, but relatively low-diversity polychaete assemblage. Figure 5 is a chart showing the stratigraphical ranges of identified polychaete taxa from the drill core and the erratics. In total, the collection comprises scolecodonts predominantly belonging to four genera: Oenonites Hinde, Reference Hinde1879; Mochtyella Kielan-Jaworowska, Reference Kielan-Jaworowska1961; Vistulella Kielan-Jaworowska, Reference Kielan-Jaworowska1961; and ‘Xanioprion’. These genera, in turn, belong to three families: Polychaetaspidae, Mochtyellidae and probably Xanioprionidae, all of which are characteristic components of Upper Ordovician polychaete assemblages of Baltoscandia (e.g. Kielan-Jaworowska, Reference Kielan-Jaworowska1966; Hints, Reference Hints2000; Hints & Eriksson, Reference Hints and Eriksson2007a). In addition, there are a few genera that are considerably less common, such as Atraktoprion Kielan-Jaworowska, Reference Kielan-Jaworowska1962; Pteropelta Eisenack, Reference Eisenack1939; Kozlowskiprion Kielan-Jaworowska, Reference Kielan-Jaworowska1966; Xanioprion Kielan-Jaworowska, Reference Kielan-Jaworowska1962; Lunoprionella Eisenack, Reference Eisenack1975; and Protarabellites Stauffer, Reference Stauffer1933. Characteristic taxa for the Dalby Limestone of the Tvären region are shown in Figures 7 and 8.

Figure 7. SEM micrographs of selected representative scolecodonts and jaw apparatuses from the Tvären-2 drill core. All specimens belong to the genus Oenonites of the labidognath family Polychaetaspidae, except (j) and (k), which may belong to closely related genera. All specimens are in dorsal view except (a3): left lateral view. Scale bars are 100 μm. (a1–a11) Oenonites aff. tuberculatus. (a1) Left MI, LO 10692t, sample TTERR; (a2) left MI, LO 10693t, sample ÅW148; (a3) left MI, LO 10694t, sample TTERR; (a4) right MI, LO 10695t, sample TTERR; (a5) right MI with anteriormost part broken off, LO 10696t, sample TTERR; (a6) right MI with posterior part broken off, LO 10697t, sample TTERR; (a7) basal plate, LO 10698t, sample TTERR; (a8) basal plate, LO 10699t, sample ÅW148; (a9) left MII, LO 10700t, sample TTERR; (a10, a11) semi-articulated dorsal maxillary apparatus of a relatively early ontogenetic stage, probably belonging to O. aff. tuberculatus, LO 10701t, sample ÅW120; (a11) close-up of the anterior portion of LO 10701t. Note the comb-like anterior maxilla. (a12) Carrier, LO 10702t, sample TTERR. (b1–b8) Oenonites gadomskae. (b1) Left MI with Lt, LO 10703t, sample ÅW120; (b2) right MI with Bp, Lt and It, LO 10704t, sample ÅW120; (b3) right MI with Bp and parts of Lt and MII, LO 10705t, sample ÅW120; (b4) semi-articulated dorsal maxillary apparatus, LO 10706t, sample ÅW120; (b5) left MI and MII with lateral teeth, LO 10707t, sample ÅW91; (b6) right MI, LO 10708t, sample ÅW120; (b7) left MI, LO 10709t, sample TTERR; (b8) right MI, LO 10710t, sample TT18. (c1–c3) Oenonites sp. A. (c1) Left MI, LO 10711t, sample TT18; (c2) left MI, LO 10712t, sample TT18; (c3) possible right MI of Oenonites sp. A, LO 10713t, sample ÅW148. (d–k) A selection of MI showing different morphotypes of Oenonites (included as Oenonites spp. in Fig. 5). (d) Left MI, LO 10714t, sample TT36; (e) large and somewhat compressed right MI possibly belonging to O. aff. tuberculatus, LO 10715t, sample TT36; (f) right MI, LO 10716t, sample ÅW137; (g) right MI, LO 10717t, sample ÅW137; (h) right MI, LO 10718t, sample ÅW91; (i) right MI, LO 10719t, sample ÅW91; (j) somewhat deformed right MI with a morphology grading towards Kozlowskiprion, LO 10720t, sample ÅW91; (k) right MI with a morphology intermediate between Oenonites and Protarabellites, LO 10721t, sample ÅW148.
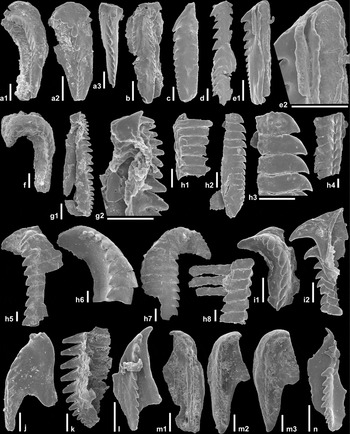
Figure 8. SEM micrographs of selected representative scoleocodonts from the Tvären-2 drill core. All specimens are in dorsal view except (c, e, g, h2, h3, h8, i2, k): in lateral view. Scale bars are 100 μm. (a1–a3) Vistulella kozlowskii. (a1) Left MI with basal ridge, LO 10722t, sample ÅW120; (a2) left MI, LO 10723t, sample ÅW148; (a3) possible right MI, LO 10724t, sample TT18. (b) Mochtyella cristata, left MI, LO 10725t, sample ÅW148. (c) Mochtyella sp. A right MI, LO 10726t, sample ÅW148. (d) Mochtyella? sp. left MI, LO 10727t, sample ÅW91. (e1, e2) Mochtyella sp. A, right MI, LO 10728t, sample ÅW91; (e2) close-up of anterior portion of (e1); note the jaw-in-jaw structure. (f) Mochtyella aff. fragilis, left MI, LO 10729t, sample TT14. (g1, g2) Placognath? Left MI, LO 10730t, sample ÅW148; (g2) close-up of (g1); note the lamellae-like anterior denticle plates. (h1–h8) A selection of maxillae and fragments assigned to ‘Xanioprion’ sp. A. Note the very well-pronounced lamellae-like structure and prolonged denticle boundaries. (h1) LO 10731t, sample ÅW148; (h2, h3) LO 10732t, sample ÅW148; (h3) close-up of (h2); (h4) LO 10733t, sample TT18; (h5) LO 10734t, sample TT18; (h6) LO 10735t, sample TT14; (h7) LO 10736t, sample TT16; (h8) LO 10737t, sample TT18. (i1, i2) Xanioprion cf. borealis. (i1) Left MII, LO 10738t, sample ÅW120; (i2) possible basal plate, LO 10739t, sample ÅW148. (j) Atraktoprion sp., right MI, LO 10740t, sample TT18. (k) Lunoprionella sp., LO 10741t, sample TTERR. (l) Protarabellites? sp. B, LO 10742t, sample ÅW91. (m1–m3) Pteropelta cf. kielanae. (m1) Left MI, LO 10743t, sample TT36; (m2) right MI, LO 10744t, sample TT36; (m3) right MI, LO 10745t, sample TT36. (n) Kozlowskiprion brevialatus, left MI, LO 10746t, sample ÅW91.
5.a. The Tvären-2 drill core assemblage
The taxonomic composition of the polychaete assemblage, as well as the abundance and relative frequency of different taxa, change successively throughout the Dalby Limestone in the Tvären-2 drill core. While some taxa range through the entire sequence, others have more restricted occurrences, possibly as a consequence of being more stenotopic in nature.
In particular, the assemblages recorded from about the lowermost 8 m of the Dalby Limestone have a relatively homogeneous composition and differ from those occurring higher up in the sequence. In general, the former interval is characterized by relatively scolecodont-rich samples dominated by four genera: Oenonites, Mochtyella, Vistulella and ‘Xanioprion’ (Fig. 5). Oenonites is the single most common genus throughout the sequence. In the lower part of the study interval it generally comprises approximately 50 % of the scolecodonts (MI) in a sample. Higher up in the core the faunal composition changes slightly and the genus becomes even more common, comprising 60–70 % of the assemblages (Fig. 5). Oenonites is species-rich and obviously represents a ‘wastebasket genus’, currently housing a variety of species and morphologies, and in need of rigorous analysis. Although beyond the scope of the present study, future studies most probably will subdivide this genus further (see also Hints, Reference Hints2000; Eriksson & Hints, Reference Eriksson and Hints2009). Because of this and also the variability in terms of preservation and degree of deformation, counting and identification of these specimens sometimes posed a problem. This is the reason why some were lumped together as Oenonites spp. (Fig. 5). Altogether the genus may be represented by five to six different species in the drill core, a few of which are more distinct than others (see various species and morphotypes in Fig. 7).
Two species are particularly common and characteristic: Oenonites aff. tuberculatus (Fig. 7a) with its relatively large-sized jaws, and the considerably smaller O. gadomskae (Kielan-Jaworowska, Reference Kielan-Jaworowska1966) (Fig. 7b). The first species is closely similar to O. tuberculatus as described by Kozłowski (Reference Kozłowski1956) and Kielan-Jaworowska (Reference Kielan-Jaworowska1966), from erratic boulders, but differs in some details, such as the posterior-most termination of the basal plate and the MI. Both these taxa, and O. aff. tuberculatus in particular, were recorded in almost all samples and seem to have been hardy, eurytopic taxa. O. gadomskae and O. tuberculatus are known from several localities in Baltoscandia (Kielan-Jaworowska, Reference Kielan-Jaworowska1966; Hints, Reference Hints2000; Hints & Eriksson, Reference Hints and Eriksson2007a) but have previously not been recorded from Sweden. A few specimens, assigned to Oenonites sp. A, have a characteristic left MI with a diagnostic posterior termination (Fig. 7c). Similar forms are known for example from the Silurian of Gotland (Eriksson, Reference Eriksson1997). In addition to these taxa, there are a number of considerably less common representatives (treated as morphotypes) of Oenonites, with a scattered occurrence throughout the drill core (Fig. 7d–i). One right MI recovered (Fig. 7k) has an interesting morphology intermediate between Oenonites and the ramphoprionid genus Protarabellites.
The placognath genera Vistulella, Mochtyella and possibly Pistoprion (belonging to the family Mochtyellidae) together usually comprise some 20–30 % of the scolecodonts. Vistulella kozlowskii Kielan-Jaworowska, Reference Kielan-Jaworowska1961 is one of the most common and characteristic mochtyellids in the Tvären-2 sequence (Fig. 8a). This long-ranging species is well known from the Ordovician and Silurian of Baltoscandia (Kielan-Jaworowska, Reference Kielan-Jaworowska1966; Hints, Reference Hints1998). It commonly comprises 10–20 % of the scolecodonts in the samples from the lower part of the studied sequence and becomes slightly less common upwards with its last occurrence recorded at approximately 91 m core depth. Pistoprion Kielan-Jaworowska, Reference Kielan-Jaworowska1966 was identified with uncertainty. The remaining mochtyellids are identified as species of Mochtyella, the most species-rich genus of the family (see, e.g. Kielan-Jaworowska, Reference Kielan-Jaworowska1966; Szaniawski, Reference Szaniawski1970; Hints, Reference Hints2000; Hints & Eriksson, Reference Hints and Eriksson2007a). Based on the better-preserved specimens, the characteristic species Mochtyella cristata Kielan-Jaworowska, Reference Kielan-Jaworowska1961 was identified (Fig. 8b). At least two additional Mochtyella species occur in the sequence. One was tentatively assigned to the M. fragilis (Fig. 8f). A relatively common form includes the simple, saw-blade-shaped MI assigned to Mochtyella sp. A (Fig. 8c, e).
As mentioned above, ‘Xanioprion’ is one of the most common genera in the lower metres of the sampled core, where it can form more than 25 % of the scolecodonts (Fig. 5). This genus most probably belongs to a taxon with a placognath type of jaw apparatus and exhibits characteristic maxillae with thin, prolonged denticle boundaries giving them a lamellae-like appearance (Fig. 8h). Such maxillae have been observed in a number of different polychaete genera, such as Lunoprionella (see Eisenack, Reference Eisenack1975), Xanioprion (see Kielan-Jaworowska, Reference Kielan-Jaworowska1966; Hints, Reference Hints1998) and ‘Xanioprion’ of Hints & Nõlvak (Reference Hints and Nõlvak2006). However, because its jaw apparatus architecture is not known, it cannot be confidently assigned to a known genus at present. Because the specimens at hand show most resemblance to those of xanioprionids, they are here tentatively treated as ‘Xanioprion’, although they may require an exclusive generic assignment in the future. ‘Xanioprion’ sp. A, the sole species represented, is common in approximately the lowermost 8 m of the core and then it almost disappears. A few specimens were recorded at approximately 110 m, above which it completely disappears from the drill core (Fig. 5).
In addition to ‘Xanioprion’ there are a number of elements that belong to the normal type xanioprionids (see Kielan-Jaworoska, Reference Kielan-Jaworowska1962, Reference Kielan-Jaworowska1966). Xanioprion (Fig. 8i) occurs relatively regularly in the lower part of the core and disappears at approximately 105 m core depth. It is generally rare with only a few specimens occurring in each productive sample. The specimens recorded seem to be conspecific to Xanioprion borealis Kielan-Jaworowska, Reference Kielan-Jaworowska1962, or a closely related species. xanioprionids form a common component in Upper Ordovician and Silurian assemblages (e.g. Kielan-Jaworowska, Reference Kielan-Jaworowska1966; Eriksson, Bergman & Jeppsson, Reference Eriksson, Bergman and Jeppsson2004; Hints & Eriksson, Reference Hints and Eriksson2007a).
Prionognath taxa and their allies (atraktoprionids, skalenoprionids and kalloprionids) are exceedingly rare and only one incomplete right MI, assigned to Atraktoprion, was recorded in sample TT18, from the lowermost part of the core (Figs 5, 8j). Although appearing already in the Darriwilian in Baltoscandia, atraktoprionids become considerably more diverse in Silurian strata.
Lunoprionella is represented by one or possibly two species (Figs 5, 8k). This genus was established on material from Baltic erratics (Eisenack, Reference Eisenack1975) and has not been thoroughly revised since its description. We agree with Hints (Reference Hints1998) that some of the species names of Eisenack (Reference Eisenack1975) most probably are synonymous. Neither the full apparatus architecture nor the position of the isolated elements is yet known.
As mentioned above, the upper half of the drill core contains some polychaete taxa not present in the lower part, such as, for example, representatives of the labidognath families Ramphoprionidae and Polychaeturidae, and Kozlowskiprion of the family Polychaetaspidae. The samples from this part of the core generally are less abundant in scolecodonts than those from the lower part, and the uppermost metres of the core only yielded very few specimens in total (Fig. 6).
The Ramphoprionidae are very rare in the material at hand and only two specimens, tentatively assigned to Protarabellites, were recorded in two samples from the uppermost 10 m or so of the core (Fig. 5). The right MI shown in Figure 8l has an overall morphology resembling that of Protarabellites but also, to some extent, Symmetroprion Kielan-Jaworowska, Reference Kielan-Jaworowska1966.
From an interval between 111.17 m and up through about 95 m, characteristic polychaeturid specimens were recorded from three samples (Figs 5, 8m). The left MI correspond well to those of Pteropelta kielanae (Hints, Reference Hints1998). However, the right MI have some characters similar also to Pteropelta sp. A of Hints & Eriksson (Reference Hints and Eriksson2010); see also Eriksson & Hints (2009, fig. 5n). Although rare in the Tvären-2 core, both polychaeturids and ramphoprionids are characteristic of the Upper Ordovician of Baltoscandia, and the former became particularly abundant in slightly younger strata (Hints, Reference Hints2000; Hints & Eriksson, Reference Hints and Eriksson2007a, Reference Hints and Eriksson2010; Eriksson & Hints, Reference Eriksson and Hints2009; see also Section 6).
Kozlowskiprion is another polychaetaspid genus that may be quite abundant, but considerably less species-rich than Oenonites, in Upper Ordovician and Silurian strata (Kielan-Jaworowska, Reference Kielan-Jaworowska1966; Eriksson, Reference Eriksson1997; Hints, Reference Hints1998; Eriksson, Bergman & Jeppsson, Reference Eriksson, Bergman and Jeppsson2004). In the material at hand, however, it is merely represented by a few MI, assigned to K. brevialatus Kielan-Jaworowska, Reference Kielan-Jaworowska1966 (Fig. 8n). All of them were recorded from one sample in the upper part of the drill core (Fig. 5). In addition to K. brevialatus, there are a few specimens which appear intermediate between Kozlowskiprion and Oenonites (see, e.g. Fig. 7j).
In addition to the taxa mentioned above, the Tvären-2 drill core yielded a few specimens of uncertain affinity, even at the family level. Most of these are more or less fragmentary elements belonging to placognath or possibly ctenognath type taxa (see Fig. 8d, g). Their presence is indicated as ‘placo-/ctenognatha indet.’ in Figure 5.
5.b. The Ringsön island erratics polychaete assemblage
The sample from the digested erratics (TTERR) collected from the island of Ringsön yielded a medium abundant (124.4 MI/kg of rock) and relatively low-diversity polychaete assemblage (Figs 5, 6). Several specimens are relatively large, and preservation is surprisingly good considering the texture and relatively coarse grain size of these rocks. The assemblage is chiefly composed of four taxa: Vistulella kozlowskii, ‘Xanioprion’ sp. A, O. gadomskae and, in particular, O. aff. tuberculatus. The latter species alone forms approximately 65 % of the Ringsön assemblage. In addition, a few specimens of Mochtyella, Lunoprionella and another Oenonites morphotype were recorded. Thus, in terms of taxonomic composition and genus-level relative frequency, the assemblage from the erratics most closely resembles that recorded from the lowermost part of the Dalby Limestone of the Tvären-2 drill core, although a few of the rare taxa recorded from the latter are missing (Fig. 5).
5.c. Cluster analysis, abundance and relative frequency
A genus-level relative frequency (%) analysis was performed on the data at hand (Fig. 5), in which the specimens (MI) were counted as belonging to either one of these five categories: Oenonites, Mochtyella, Vistulella, ‘Xanioprion’ and Other. The results are shown as pie charts for a select number of productive samples in Figure 5. Based on this, it is obvious that Oenonites is by far the single most abundant genus, generally forming > 50 % of the scolecodonts in a sample. It is also apparent that the samples from the lower part of the succession generally have fewer specimens belonging to this genus than those deriving from higher up in the sequence. Albeit highly variable, the abundance values generally decrease upwards in the core (cf. Fig. 6). A cluster analysis was performed in order to test which samples had the highest degree of correspondence in terms of genus-level relative frequency. After running the data through the PAST software and performing the Paired Group, Brey-Curtis cluster analysis, the resulting tree shows a topography in which there are two main clusters, one grouping the samples from the lowermost part of the core and the other grouping samples from the succeeding part (Fig. 9). The samples of the former cluster all share a similar taxonomic composition and relative frequency of genera, including a relatively large percentage of ‘Xanioprion’, which is rare or absent in the samples forming the latter cluster (see also Fig. 5). The Ringsön material clusters most tightly to the TT samples in the lowermost part of the core (Fig. 9). Virtually the same results were achieved when a trial run was performed including all samples.
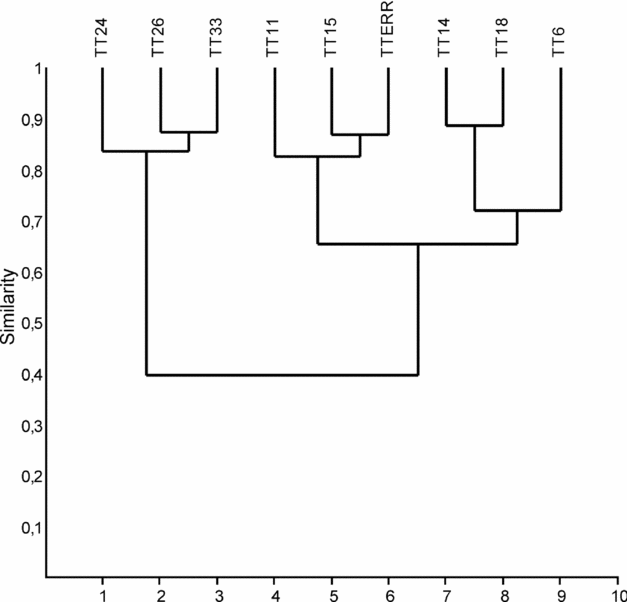
Figure 9. Dendrogram of a cluster analysis run on samples from the Tvären-2 drill core and the Ringsön erratics, using the paired group algorithm and Bray-Curtis similarity measure of the PAST software (see Hammer, Harper & Ryan, Reference Hammer, Harper and Ryan2001). A rarefaction curve was established which made us remove samples with less than 20 specimens recorded. The same set of variables, as used for calculating the genus-level relative frequency (see Fig. 5), was applied.
6. Comparison with coeval polychaete faunas from other regions
At the global scale, the jawed polychaete faunas had become relatively diverse by the early Late Ordovician (Fig. 10; Hints & Eriksson, Reference Hints and Eriksson2007a). The Kukruse, during which the Tvären impact occurred, corresponds to Time Slice 5a of Webby et al. (Reference Webby, Cooper, Bergström, Paris, Webby, Paris, Droser and Percival2004), in which Hints & Eriksson (Reference Hints and Eriksson2007a, table 2) recorded at least 27 polychaete genera. Because of the relatively limited number of taxonomic studies published on these fossils, this figure probably is greatly underestimated and should be considered only as a very conservative estimate. It should also be noted that most data on Ordovician polychaetes are derived from the palaeocontinents of Laurentia and Baltica (e.g. Eriksson & Bergman, Reference Eriksson and Bergman2003; Hints & Eriksson, Reference Hints and Eriksson2007a,Reference Hints and Erikssonb). The Tvären assemblage shows closest affinity to the known Baltoscandian polychaete faunas, which are addressed below.

Figure 10. The global genus-level diversity pattern of Ordovician polychaetes, as based on the scolecodont record (modified from Hints & Eriksson, Reference Hints and Eriksson2007a; Bergström et al. Reference Bergström, Chen, Gutiérrez-Marco and Dronov2009). Time slices (TS) after Webby et al. (Reference Webby, Cooper, Bergström, Paris, Webby, Paris, Droser and Percival2004). Swedish Ordovician scolecodont record: Ö – Öland; T – Tvären; S – Sularp; L – Lockne; G – subsurface Gotland (see the main text for further details).
In the early 1960s, Kielan-Jaworowska analysed scolecodonts recovered from a large number of glacially transported erratic boulders (many probably deriving from Sweden and Estonia) collected in northern Poland (see Kielan-Jaworowska, Reference Kielan-Jaworowska1966 and references therein). Based on that material she outlined a Llandeilo–lower Caradoc (Darriwilian–Sandbian) polychaete assemblage that included 31 species belonging to 13 genera (Kielan-Jaworowska, Reference Kielan-Jaworowska1966, table 1). She noted (p. 26) that the boulders comprising rocks of Kukruse or Idavere age predominantly included species of Oenonites (= Polychaetaspis) and/or Ramphoprion Kielan-Jaworowska, Reference Kielan-Jaworowska1962. The abundant polychaetaspid assemblages were dominated by four species: O. tuberculatus, O. gadomskae, O. wyszogrodensis (Kozłowski, Reference Kozłowski1956) and O. varsoviensis (Kielan-Jaworowska, Reference Kielan-Jaworowska1966).
Hints (Reference Hints1998, fig. 6) recorded 22 species belonging to at least 13 genera from the Kukruse Stage and 25 species from the overlying Idavere Substage of Estonia and the St Petersburg region. Hints (Reference Hints2000, p. 47) noted that the Lasnamägi to Idavere interval was characterized by a rapidly increasing number of genera and species and concluded that ramphoprionids are particularly characteristic of this interval in Estonia and surrounding areas. In another paper, Hints (Reference Hints and Põldvere2001) studied the distribution of scolecodonts in the deeper shelf setting represented in the Valga drill core (Estonia) and concluded that the Kukruse interval was particularly rich in polychaetaspids (Oenonites), ramphoprionids and mochtyellids. He also mentioned that a species of Pistoprion Kielan-Jaworowska, Reference Kielan-Jaworowska1966, not recorded in the Valga core, is common in this interval in northern Estonia. The latter genus is common also in younger (Pirgu) strata in Sweden (Eriksson & Hints, Reference Eriksson and Hints2009) but has not been recorded with certainty in the material at hand.
The Tvären polychaete assemblage is of relatively low diversity, particularly in terms of genera, compared to many other coeval ones from Baltoscandia. It contains some faunal elements that are typical of the Upper Ordovician strata of this palaeocontinent, that is, with a general dominance of polychaetaspids and mochtyellids and other placognaths. However, there are also similarities at the species level, especially with the occurrence of O. aff. tuberculatus and O. gadomskae, but also P. cf. kielanae, K. brevialatus, M. cristata, and possibly X. borealis. These seem to represent hardy taxa that easily spread within the Baltoscandian Basin. Several taxa characteristic of the lower Upper Ordovician of Estonia, Poland and the St Petersburg region are, however, absent or very rare in the post-impact Dalby Limestone sequence of Tvären. For example, considering the abundance of ramphoprionids in this interval mentioned by both Kielan-Jaworowska (Reference Kielan-Jaworowska1966) and Hints (Reference Hints2000, Reference Hints and Põldvere2001), their scarcity in Tvären is anomalous. Perhaps their environmental preferences were not optimal in the studied area.
The difference observed between the Tvären assemblages and those recorded from other regions of Baltoscandia could be related to local palaeoenvironmental factors. The Tvären crater may, despite its relative abundance in scolecodonts, have offered a somewhat unstable and stressed environment that allowed opportunistic taxa to bloom, but a low-diversity polychaete fauna to prevail.
7. The Swedish Ordovician scolecodont record
The record of Ordovician scolecodont-bearing polychaetes from Sweden is meagre and, with one possible exception, not precisely coeval to the Kukruse Tvären assemblage described herein. Eisenack (Reference Eisenack1976) described a few specimens from the Darriwilian (Kunda Stage) Vaginatum Limestone (currently known as the Holen Limestone) at Hälludden on the island of Öland, southeastern Sweden. Most specimens were assigned to Xanioprion and Anicocerasites Eller, Reference Eller1955, of which the latter form genus needs revision. A few Mochtyella-reminiscent placognath type maxillae and a basal plate of Oenonites can be identified among his illustrated specimens (see Eisenack, Reference Eisenack1976, pl. 1–2).
Schallreuter (Reference Schallreuter1983) recorded a microfossil assemblage from erratics found in Westphalia, Germany, with a provenance determined to the Sularp Formation (or Shale) of Scania, the southernmost province of Sweden. The Sularp Formation is of middle Viru Age (upper Kukruse through Haljala) and, thus, partly coeval with the Dalby Limestone (Bergström et al. Reference Bergström, Huff, Kolata, Yost and Hart1997, Reference Bergström, Larsson, Pålsson and Ahlberg2002). Two scolecodonts assigned to Mochtyella? sp. and Vistulella? sp. were illustrated (Schallreuter, Reference Schallreuter1983, pl. 1, figs 9, 10). The latter specimen has denticles that are secondarily denticulated, which may suggest that it rather belongs to Rakvereprion balticus (Eisenack, Reference Eisenack1975), a species common in deeper-water settings (see Mierzejewski, Reference Mierzejewski1978; Hints, Reference Hints2000). Interestingly, Bergström et al. (Reference Bergström, Huff, Kolata, Yost and Hart1997, p. 233) noted that the Sularp Formation is a deeper-water equivalent of the Dalby Limestone which would fit well also with a record of R. balticus.
Most recently, Eriksson & Hints (Reference Eriksson and Hints2009) described an Upper Ordovician assemblage associated with mud-mounds from subsurface Gotland, southeastern Sweden, belonging to the Katian (Pirgu Stage) Klasen Member. Albeit significantly smaller, that collection recorded a more diverse fauna, dominated by members of Oenonites, Mochtyella and Pistoprion, than the one described here.
Studies of numerous sections, by ÅMF, in the nearly contemporaneous impact crater at Lockne, central Sweden (Lindström et al. Reference Lindström, Sturkell, Torberg and Ormö1996; Lindström et al. Reference Lindström, Ormö, Sturkell, von Dalwigk, Koeberl and Henkel2005 and references therein) have not recorded any scolecodonts. However, Sturkell et al. (Reference Sturkell, Ormö, Nõlvak and Wallin2000) reported infrequent fragments from the youngest, pre-impact beds of the Dalby Limestone and from the oldest, post-impact strata at the locality Hallen. At that locality the most distal part of the impact strata has been found and the resurge presumably merely generated minor erosion of the still existing pre-impact sediments, owing to the distance of 45 km from the centre of the crater to Hallen. This would be the minimum distance, as the Caledonian thrust movement shortened the original distance between the localities. Although preliminary, these observations suggest that environments were less suitable for jaw-bearing polychaetes in the Lockne region as compared to those of the Tvären Bay, possibly related to the considerably deeper-water setting inferred for the former (e.g. Lindström, Shuvalov & Ivanov, Reference Lindström, Shuvalov and Ivanov2005; Shuvalov, Ormö & Lindström, Reference Shuvalov, Ormö, Lindström, Koeberl and Henkel2005; Ormö, Sturkell & Lindström, Reference Ormö, Sturkell and Lindström2007).
8. Discussion
As soon as the deposition of the Dalby Limestone commenced in the Tvären crater, the vacant ecospace was successively exploited by a range of organisms, including vagrant and sessile benthos, nekton and planktonic organisms. As the crater filled in, an ecological succession can be observed with an increasing number of higher taxa and tiering levels, both in terms of infauna and epifauna (Fig. 3). Hence, the post-impact environments of Tvären region offered environments suitable for a plethora of marine organisms, of which jawed polychaetes obviously played a significant part. The palaeoecological succession observed within the post-impact sequence of the Tvären-2 drill core indicates a successively changing environment, presumably shallowing upwards as the crater gradually filled with sediments (Lindström et al. Reference Lindström, Flodén, Grahn and Kathol1994). The comparatively high rates of sedimentation of the post-impact strata permit a prominent resolution of the records of palaeoenvironmental development following soon after the impact.
According to Lindström et al. (Reference Lindström, Flodén, Grahn and Kathol1994), members of the plankton population were first to be established in the sediment column and they recorded graptolites and chitinozoans as the sole fossil groups represented in the mudstone intercalated with coarse sand in the interval 161.4–149.0 m that was formed very early after the impact. They referred to the interval above 149 m as the ‘normal’ part of the core sequence and it is represented by the Dalby Limestone. Scolecodonts first appeared at this level and immediately came to form one of the numerically most abundant groups of fossils. This does not, however, necessarily imply that the crater floor was already colonized by polychaetes by that time. Lindström et al. (Reference Lindström, Flodén, Grahn and Kathol1994, p. 101) noted that bioturbation first becomes apparent just below 140 m and more or less frequent from 130 m and upwards, which to them indicated the initial colonization of the crater floor by a benthic endofauna. Because scolecodonts appear well before the earliest bioturbation, Lindström et al. (Reference Lindström, Flodén, Grahn and Kathol1994), moreover, assumed that the first polychaetes to appear, and possibly many of the later ones, were not necessarily burrowers or that their scolecodonts had been transported there. The latter alternative was preferred by those authors.
Our results support this scenario and we believe that the initial Dalby Limestone scolecodont assemblages (or at least the bulk of them) were indeed transported into the crater floor from the crater wall or rim. Data in support of this are the correspondence in taxonomic composition between the shallow water rim facies material of the Ringsön erratics and that recorded in the deepest part of the crater as represented by the lowermost sampled 8–10 m or so of the drill core. Those assemblages both include a relatively high percentage of ‘Xanioprion’ (Figs 5, 9). The level at which this typical ‘Xanioprion’-rich assemblage shifts in nature in the drill core thus roughly corresponds with the first signs of bioturbation (Figs 3, 5), which in turn may suggest that this was the time of polychaete crater colonization. Moreover, the topmost samples of the core are not as abundant in scolecodonts, which fits with a decrease in bioturbation. Thus, there seems to be a roughly positive correlation between scolecodont abundance and bioturbation, except for the lowermost part of the core which is relatively rich in scolecodonts, although those are supposedly transported. These results also indicate that many of the other fossils in the lower part of the drill core, at least those with hydrodynamic properties similar to that of scolecodonts, were transported into the crater floor. It would also suggest that ‘Xanioprion’, even though common in the deepest part of the core, was more closely associated with shallower water environments such as those inferred at the crater rim.
Some sample levels in the core (e.g. TT6, TT24, TT26 and TT29; Fig. 6) have a lithology and general macrofossil content consistent with that of the rim facies of the erratics. The scolecodont association in some of those samples does not fit as well with that from the erratics (TTERR sample) as would perhaps be expected, indicating that the relationship between the erratics and the crater sequence turbidites is more complex than previously thought. These biocalcarenite beds formed through settling of turbidity currents, and because turbidites are apparent already at approximately 145 m core depth, it is likely that the rim facies was established already by that time. As the succession of the rim facies biota is expected to change from its initial colonization, we should anticipate different faunal elements in the turbidites. Material slumping from the crater rim may, moreover, have ripped up additional material from the crater wall during its downward movement. The mixture of components of various depths from upper to lower parts of the rim stratigraphy may have been incorporated into a single turbidite bed, showing a mixed assemblage, including lower to upper crater rim species from the rim facies. The first 20 m, as well as the levels between 102.0 and 95.0 m, are abundant in actual turbidites. Depending on the steepness of the inner crater, rim sediments ought to have been transported downslope and inwards to the crater depression and, hence, sediments would have been deposited at different positions in the crater. Many of the biocalcarenitic turbidite beds show pronounced grading upwards.
In contrast to the aforementioned Lockne crater, the magnitude of the Tvären impact and its possible effects on the regional environment are poorly known, since no impact strata are preserved in situ exterior to the crater structure. Hence, there is only evidence of local environmental response to the impact. Because few studies have dealt with the post-impact event colonization phase and instead focused on the extinction of taxa, comparable studies are not straightforward. Moreover, our knowledge of fossil polychaetes is patchy, and many taxonomic studies and revisions are pending, making it difficult to evaluate their general response to environmental changes, including impact and extinction events. None the less, there are some data available that shed some light onto these issues. Several studies on large-scale catastrophes and following mass extinctions in the Phanerozoic record are focused on the Cretaceous–Palaeogene (K–P) boundary and the Permian–Triassic boundary. At the Permian–Triassic boundary a majority of marine genera disappeared, but Kozur (Reference Kozur1998) showed that a large part of them, belonging to warm-water benthos, such as polychaetes (as represented by scolecodonts), holothurians, siliceous sponges, ostracods, bivalves and gastropods, reappeared as Lazarus taxa in the late Olenekian or in the Middle Triassic. According to Kozur, 100 % of the polychaete genera reappeared after the Permian–Triassic boundary.
Hints et al. (Reference Hints, Hints, Meidla and Sohar2003) showed that the Late Ordovician ash fall resulting in a thick K-bentonite, the Kinnekulle Bed, did not affect the polychaete faunas to a very large extent and less than, for example, ostracods. Although there are indications (primarily based on the general composition of Ordovician and Silurian assemblages, respectively) suggesting that the end-Ordovician extinction event led to re-organization of the polychaete faunas, the data presently at hand suggest that there was no major drop in genus-level diversity, at least not compared to many other groups of fossils (Eriksson, Bergman & Jeppsson, Reference Eriksson, Bergman and Jeppsson2004; Hints & Eriksson, Reference Hints and Eriksson2007a). During the Silurian, the oceanic events that were associated with conspicuous extinctions among various metazoans, such as the Llandovery–Wenlock Ireviken Event and the late Ludlow Lau Event, had an impact on the polychaete faunas, although seemingly not to the same extent as for planktonic and nektonic organisms such as conodonts, graptolites and fish (e.g. Aldridge, Jeppsson & Dorning, Reference Aldridge, Jeppsson and Dorning1993; Jeppsson, Reference Jeppsson, Landing and Johnson1998, Reference Jeppsson2006; Eriksson, Bergman & Jeppsson, Reference Eriksson, Bergman and Jeppsson2004; Eriksson, Reference Eriksson2006; Hints et al. Reference Hints, Killing, Männik and Nestor2006; Eriksson, Nilsson & Jeppsson, Reference Eriksson, Nilsson and Jeppsson2009 and references therein). Although more detailed analyses are certainly needed, this indicates that polychaetes were relatively hardy taxa that perhaps were less affected by environmental changes and extinction events than a number of other clades. This fits well with their documented long evolutionary ranges and representatives considered to be living fossils (e.g. Szaniawski & Imajima, Reference Szaniawski and Imajima1996).
This study obviously raises new questions regarding the polychaete faunas of the Tvären region. Did the impact event lead to biological destruction with ecosystem reorganizations and extinctions or did it cause organismal, ecosystem or evolutionary benefits in the shape of new habitats and niches, environmental conditions, and improved nutrient availability? In order to assess the ‘beneficial’ influence or biological destructiveness (cf. Cockell & Bland, Reference Cockell and Bland2005) to the polychaete faunas, the pre-impact Dalby Limestone would need to be analysed from adjacent localities, as it is not preserved in the studied region. Although local environmental conditions may have influenced the faunas, such studies would elucidate the taxonomic composition of the polychaete faunas just prior to the impact and provide clues as to the subsequent changes, if any, they went through.
9. Conclusions
The results of this study can be summarized as follows:
(1) Both the depression and the rim of the post-impact Kukruse Tvären crater offered suitable environmental conditions for a number of metazoan clades, including a thriving jawed polychaete fauna, even though it is not particularly diverse taxonomically.
(2) Scolecodonts are among the first members of the non-planktonic groups to appear in the post-event strata and belong to the numerically most abundant groups of fossils represented in the Tvären-2 drill core.
(3) The overall assemblage recorded from the post-event Dalby Limestone has a composition characteristic of that of the Upper Ordovician of Baltoscandia. It is similar to such assemblages previously described from Poland and Estonia, with Oenonites, Vistulella and Mochtyella representing the most common genera together with ‘Xanioprion’. In addition, species of Pteropelta, Protarabellites?, Atraktoprion and Xanioprion occur in lower frequency.
(4) The Tvären assemblage differs from other coeval ones reported from Baltoscandia, particularly in its poorly represented ramphoprionid fauna and, at least in parts of the succession, a relatively high frequency of ‘Xanioprion’.
(5) A succession of different taxa is observed from the deepest part of the crater and upwards towards more shallow, higher energy, water settings.
(6) The initial post-impact Dalby Limestone polychaete assemblage does not necessarily represent a crater floor colonization of vagrant benthos. Instead, the lowermost assemblage seems to be a taphocoenosis, as indicated by its taxonomic correspondence to the rim facies fauna recovered from the Ringsön erratics.
(7) There is a rough positive correlation between scolecodont occurrence and abundance and levels of increased bioturbation in the Tvären-2 drill core.
(8) The Tvären strata have yielded considerably richer scolecodont assemblages than hitherto recorded from the approximately coeval Lockne crater, possibly as a consequence of shallower water settings in the former area.
Acknowledgements
Thanks to Åsa Wallin (Lund) for allowing us to study some HF-processed sample residues from the Tvären-2 drill core. This work has been supported by grants from Lars Hierta Memorial Foundation, The Swedish Research Council (Vetenskapsrådet), The Royal Swedish Academy of Sciences (KVA), IAS postgraduate grant, Bjurzons travel grant, and a Leksand Municipal Scholarship. Funding from the Swedish Research Council and Magnus Bergwalls Stiftelse to Lars Holmer, Uppsala University, is also acknowledged by ÅMF. We are grateful towards Stig M. Bergström (Columbus) and Olle Hints (Tallinn) for critically reading and improving the manuscript.