1. Introduction
The recycling of materials from a subducting slab back into the overlying crust is arguably the most important geochemical cycle on Earth (Jarrard, Reference Jarrard1986; Anderson, Reference Anderson1989, Reference Anderson2001; Turcotte & Schubert, Reference Turcotte and Schubert2002; Conrad & Lithgow-Bertelloni, Reference Conrad and Lithgow-Bertelloni2003; Anderson, Reference Anderson2006; Spandler & Pirard, Reference Spandler and Pirard2013). The component exchange between the subducting slab and the overlying depleted mantle takes place in subduction zones. Experimental investigations (Tatsumi et al. Reference Tatsumi1989; Brenan et al. Reference Brenan, Shaw, Ryerson and Phinney1995; You et al. Reference You, Castillo, Gieskes, Chan and Spivack1996; Kessel et al. Reference Kessel, Schmidt, Ulmer and Pettke2005) and studies of active subduction arcs (McCulloch & Gamble, Reference McCulloch and Gamble1991; Pearce & Peate, Reference Pearce and Peate1995; Münker et al. Reference Münker, Worner, Yogodzinski and Churikova2004; Turner et al. Reference Turner, Handler, Bindeman and Suzuki2009; Handley et al. Reference Handley, Turner, Macpherson, Gertisser and Davidson2011) have suggested that both Nd and Hf are relatively fluid-immobile elements (e.g. compared to Sr). Recent work by Chauvel et al. (Reference Chauvel, Marini, Plank and Ludden2009) showed that altered basalts from the western Pacific are indistinguishable from unaltered Pacific MORB (mid-ocean ridge basalt) in their Hf–Nd isotopic ratios. Such results support those of previous studies (e.g. White & Patchett, Reference White and Patchett1984), which demonstrated that hydrothermal alteration has little or no effect on these ratios (cf. Sr isotopes; Staudigel et al. Reference Staudigel, Davies, Hart, Marchant and Smith1995) and, more importantly, provided a means of constraining the contribution of subducting sediments to island arc magmatism (Handley et al. Reference Handley, Turner, Macpherson, Gertisser and Davidson2011).
The Central Asian Orogenic Belt (CAOB), also known as the Altaids, represents the largest Phanerozoic accretionary orogenic belt in the world, situated between the Siberian, East European, Tarim and North China cratons (Fig. 1a; Jahn et al. Reference Jahn, Windley, Natalin's and Dobretsov2004; Safonova et al. Reference Safonova, Buslov, Iwata and Kokh2004; Yakubchuk, Reference Yakubchuk2004; Yuan et al. Reference Yuan, Sun, Xiao, Li, Chen, Lin, Xia and Long2007; Xiao et al. Reference Xiao, Han, Yuan, Sun, Lin, Chen, Li, Li and Sun2008). It is widely accepted that the CAOB developed via the episodic accretions of island arcs, ophiolites, accretionary complexes, seamounts and microcontinental blocks during the Palaeozoic (Chen & Jahn, Reference Chen and Jahn2004; Xiao, Kröner & Windley, Reference Xiao, Kröner and Windley2009; Biske & Seltmann, Reference Biske and Seltmann2010; Xiao et al. Reference Xiao, Huang, Han, Sun and Li2010; Su et al. Reference Su, Qin, Sakyi, Li, Yang, Sun, Tang, Liu, Xiao and Malaviarachchi2011, 2012).
Previous studies have shown that more than 50% of this growth involved the addition of mantle-derived juvenile materials (Jahn, Wu & Chen, Reference Jahn, Wu and Chen2000a; Kröner et al. Reference Kröner, Kovach, Belousova, Hegner, Armstrong, Dolgopolova, Seltmann, Alexeiev, Hoffmann, Wong, Sun, Cai, Wang, Tong, Wilde, Degtyarev and Rytsk2014). However, numerous crustal fragments composed of ancient continental materials are also believed to have played an important role in the accretionary process (Jahn, Wu & Chen, Reference Jahn, Wu and Chen2000b; Hong et al. Reference Hong, Zhang, Wang, Wang and Xie2004; Kröner et al. Reference Kröner, Hegner, Lehmann, Heinhorst, Wingate, Liu and Ermelov2008). Kröner et al. (Reference Kröner, Kovach, Belousova, Hegner, Armstrong, Dolgopolova, Seltmann, Alexeiev, Hoffmann, Wong, Sun, Cai, Wang, Tong, Wilde, Degtyarev and Rytsk2014) and He et al. (Reference He, Sun, Mao, Zong and Zhang2015) proposed that the evolution of the CAOB involved both the generation of juvenile material and the extensive reworking of older crust, and have argued that the production of juvenile crust during the amalgamation of the CAOB has been grossly overestimated.
The Chinese Altai, in the central part of the CAOB, is commonly considered to be part of Palaeozoic subduction–accretion terrane (or massif), which preserves a record of the accretion of a peri-Siberian orogenic system (Fig. 1b; Şengör et al., Reference Sengör, Natal'in and Burtman1993; Şengör & Natal'in, Reference Sengör and Natal'in1996; Windley et al. Reference Windley, Kröner, Guo, Qu, Li and Zhang2002; Cai et al. Reference Cai, Sun, Yuan, Zhao, Xiao, Long and Wu2011a; Long et al. Reference Long, Yuan, Sun, Xiao, Wang, Cai and Jiang2012). Granites make up c. 40% of exposed rocks in the Chinese Altai, and arc-related magmatism was almost continuous during the Palaeozoic, reaching a peak in the Devonian (Cai et al. Reference Cai, Sun, Yuan, Zhao, Xiao, Long and Wu2011a,b, Reference Cai, Sun, Yuan, Xiao, Zhao, Long and Wu2012, Wang et al. Reference Wang, Yuan, Long, Sun, Xiao, Zhao, Cai and Jiang2011; Lv et al. Reference Lv, Zhang, Tang and Guan2012). Recent studies have shown a decoupling between whole-rock Nd isotopes and zircon Hf isotopes in Early to Middle Palaeozoic granites (478–380 Ma; e.g. Yuan et al. Reference Yuan, Sun, Xiao, Li, Chen, Lin, Xia and Long2007; Sun et al. Reference Sun, Yuan, Xiao, Long, Xia, Zhao, Lin, Wu and Kröner2008; Wang et al. Reference Wang, Jahn, Kovach, Tong, Hong and Han2009; Cai et al. Reference Cai, Sun, Yuan, Zhao, Xiao, Long and Wu2011a,b; Yu et al. Reference Yu, Sun, Long, Li, Zhao, Kröner, Broussolle and Yang2016), leading to contentious debate over the significance of this finding with respect to crustal growth of the Chinese Altai (Yu et al. Reference Yu, Sun, Long, Li, Zhao, Kröner, Broussolle and Yang2016).
Based mainly on the negative ε Nd(t) values of some granites, Wang et al. (Reference Wang, Hong, Jahn, Tong, Wang, Han and Wang2006, Reference Wang, Jahn, Kovach, Tong, Hong and Han2009) proposed that the Chinese Altai is a Precambrian microcontinent derived from eastern Gondwana. In contrast, a number of other studies (Sun et al. Reference Sun, Yuan, Xiao, Long, Xia, Zhao, Lin, Wu and Kröner2008; Xiao et al. Reference Xiao, Han, Yuan, Sun, Lin, Chen, Li, Li and Sun2008; Long et al. Reference Long, Yuan, Sun, Xiao, Zhao, Wang and Cai2010; Cai et al. Reference Cai, Sun, Yuan, Zhao, Xiao, Long and Wu2011b) have argued that the Chinese Altai is an active continental margin, based on the predominantly positive zircon ε Hf(t) values present in both Palaeozoic granites and detrital zircons from metasedimentary rocks. In this study, we present zircon U–Pb ages, major and trace element geochemistry, and Sr, Nd and Hf isotopic compositions from representative samples of five granite plutons in the Chinese Altai, to better understand the crustal growth and tectonic evolution of the Chinese Altai.
2. Regional geology
The Altai Orogenic Belt, a major part of the CAOB in China, consists of clastic sedimentary and volcanic rocks of Ordovician to Devonian age and their metamorphosed equivalents, and extends eastward into Mongolia and westward into Kazakhstan and Russia (Fig. 1b; Windley et al. Reference Windley, Kröner, Guo, Qu, Li and Zhang2002, Reference Windley, Alexeiev, Xiao, Kroner and Badarch2007; Xiao et al. Reference Xiao, Windley, Badararch, Li, Sun, Qin and Wang2004; Long et al. Reference Long, Sun, Yuan, Xiao, Lin, Wu, Xia and Cai2007, 2008; Sun et al. Reference Sun, Yuan, Xiao, Long, Xia, Zhao, Lin, Wu and Kröner2008). As the central part of the Altai Orogenic Belt, the Chinese Altai has been the subject of extensive study (Sun et al. Reference Sun, Long, Cai, Jiang, Yuan, Zhao, Xiao and Wu2009; Long et al. Reference Long, Yuan, Sun, Xiao, Zhao, Wang and Cai2010).
The Chinese Altai can be divided into five fault-bounded terranes based on differences in stratigraphy, metamorphic grade, deformation patterns, magmatic activity and geochronology. These terranes are numbered 1–5, and are separated by the Hongshanzui, the Kalaxianger, the Abagong–Kurit and the Maerkakuli Faults (Fig. 1b; Sengör, Natal'in & Burtman, Reference Sengör, Natal'in and Burtman1993; Long et al. Reference Long, Sun, Yuan, Xiao, Lin, Wu, Xia and Cai2007; Yuan et al. Reference Yuan, Sun, Xiao, Li, Chen, Lin, Xia and Long2007; Sun et al. Reference Sun, Yuan, Xiao, Long, Xia, Zhao, Lin, Wu and Kröner2008; Cai et al. Reference Cai, Sun, Yuan, Zhao, Xiao, Long and Wu2011a,b). Terrane 1 comprises Late Devonian to Early Carboniferous clastic sediments, limestones and sparse island-arc volcanic rocks metamorphosed to the lower greenschist facies. Terrane 2 is composed of a Middle Ordovician turbidite succession, also metamorphosed to the lower greenschist facies. Terrane 3 is the largest of the terranes, and is composed of early Palaeozoic sediments metamorphosed to a medium to high grade. Terrane 4 consists of Devonian turbiditic sandstone, pillow basalts and some siliceous volcanic rocks. Terrane 5 is composed of a fossiliferous Devonian succession, overlain by Late Carboniferous strata.
The Habahe Group is the oldest metasedimentary unit in the Chinese Altai, and is mainly composed of a thick succession (>6000m) of slate, phyllite and schist (GCRSX, 1981; BGMRX, Reference Xiao, Zhang, Shi, Su, Sakyi, Hu and Zhang1993; Windley et al. Reference Windley, Kröner, Guo, Qu, Li and Zhang2002; Long et al. Reference Long, Sun, Yuan, Xiao, Lin, Wu, Xia and Cai2007, 2008, 2010; Cai et al. Reference Cai, Sun, Yuan, Xiao, Zhao, Long and Wu2012; Wang et al. Reference Wang, Long, Wilde, Xu, Sun, Xiao, Yuan and Cai2014). Recent whole-rock geochemical studies, and U–Pb dating of detrital zircons from metasedimentary rocks in the Habahe Group, suggest that the original sediments were derived from parent rocks generated on an active margin in the early Palaeozoic (Long et al. Reference Long, Sun, Yuan, Xiao, Lin, Wu, Xia and Cai2007, 2010). Numerous Early to Middle Palaeozoic arc-related granites and late Palaeozoic post-collisional A-type granites are emplaced within the Habahe Group strata, providing important clues for understanding magmatism in the Chinese Altai.
3. Description of samples
Sample locations are shown in Figure 2, and hand sample photographs and photomicrographs are shown in Figure 3. The Mandalehai granites are composed of quartz (33–42 vol.%), plagioclase (38–43 vol.%), biotite (3–5 vol.%) and muscovite (5–10 vol.%), with accessory cordierite (Fig. 3b). The Huiteng granites consist of quartz (33–38 vol.%), K-feldspar (5–10 vol.%), plagioclase (32–37 vol.%), biotite (5–9 vol.%) and muscovite (3–5 vol.%), with sillimanite as an accessory mineral (Fig. 3d).

Figure 3. Hand specimen photos and photomicrographs (cross-polarized and magnification of 50) of granites in the Chinese Altai: (a, b) the Mandalehai granites; (c, d) the Huiteng granites; (e, f) the Tuergen granites; (g, h) the Zhagesitai granites; and (i, j) the Zhepujilieke granites.
The Tuergen granites consist of K-feldspar (30–35 vol.%), quartz (30–40 vol.%), plagioclase (5–10 vol.%), biotite (2–5 vol.%) and muscovite (5–8 vol.%), with garnet as an accessory mineral (Fig. 3f). The Zhagestai granites are composed of quartz (25–30 vol.%), plagioclase (30–35 vol.%), K-feldspar (20–25 vol.%), biotite (2–4 vol.%) and muscovite (2–5 vol.%); accessory minerals are mainly sillimanite (Fig. 3h). The Zhepujilieke granites consist of K-feldspar (35–45 vol.%), quartz (20–25 vol.%), plagioclase (15–20 vol.%), biotite (4–6 vol.%) and muscovite (5 vol.%), with garnet as an accessory mineral (Fig. 3i).
4. Analytical methods
4.1. Major and trace element analyses
Whole-rock major and trace element compositions of the granite samples were determined at the State Key Laboratory of Ore Deposit Geochemistry, Institute of Geochemistry, Chinese Academy of Sciences (IGCAS) (Table 2). Major elements were analysed using a Leeman Prodigy inductively coupled plasma optical emission spectrometer, with high-dispersion Echelle optics. Analytical uncertainties were generally less than 1% for most oxides, except for TiO2 (1.5%) and P2O5 (2.0%), based on repeated analyses of US Geological Survey (USGS) standards BCR-1 and AVG-2 and the Chinese National Rock Standard GSR-3. Trace elements were analysed using an Agilent-7500a inductively coupled plasma mass spectrometer (ICP-MS). Data quality was assessed via repeated measurements of USGS reference materials BCR-1 and BHVO-1. The analytical precision for most trace elements was more than 95%.
4.2. Zircon U–Pb dating
Zircon grains were isolated using standard heavy liquid and magnetic separation methods. Using a binocular microscope, we selected transparent zircon grains that were free of cracks, then fixed them in epoxy resin and polished the surface to expose the grain centres. The grain mount was then cleaned and photographed. Cathodoluminescence (CL) images were obtained using a LEO1450VP scanning electron microscope, to reveal internal structures within individual zircon grains (Fig. 4). Isotope data were collected using a laser ablation multi-collector (LA-MC) ICP-MS at the State Key Laboratory of Ore Deposit Geochemistry, IGCAS. U and Pb concentrations and isotope ratios were measured simultaneously, with an Agilent 7500a quadrupole (Q)-ICP-MS instrument. Details of the analytical procedure are described in Yuan et al. (Reference Yuan, Gao, Liu, Li, Günther and Wu2004) and Xie et al. (Reference Xie, Zhang, Zhang, Sun and Wu2008)

Figure 4. CL images of zircons from (a) the Mandalehai granites, (b) the Huiteng granites, (c) the Tuergen granites, (d) the Zhagesitai granites and (e) the Zhepujilieke granites.
The 207Pb/206Pb and 206Pb/238U ratios were calculated using the GLITTER 4.0 program (Macquarie University, Australia) and zircon standards 91500, GJ-1 and NIST SRM 610. Age distribution histograms and concordia plots were constructed using the ISOPLOT 3.0 program, with standard Pb corrections as described by Andersen (Reference Andersen2002) (Fig. 5). All zircon U–Pb data are reported in Table 3; all weighted mean ages are expressed with 95% confidence intervals.

Figure 5. U–Pb concordia diagrams for zircons from (a) the Mandalehai granites, (b) the Huiteng granites, (c) the Tuergen granites, (d) the Zhagesitai granites and (e) the Zhepujilieke granites.
4.3. Sr–Nd isotopic analyses
The Sr and Nd isotopic compositions of the studied granite samples were measured at the Experimental Test Center, Tianjin Institute of Geology and Mineral Resources (Tianjin, China), using a VG 354 thermal ionization mass spectrometer (TIMS) in static mode. For each sample, c. 100–150 mg of powdered whole rock was dissolved in an HF–HClO4 solution, in a screw-top Teflon beaker. The Rb, Sr, Sm and Nd concentrations were determined using the isotope dilution method, with a 87Rb–84Sr–149Sm–144Nd spiked solution (Zhang et al. Reference Zhang, Sun, Lu, Zhou, Zhou, Liu and Zhang2001). The 143Nd/144Nd and 87Sr/86Sr ratios were normalized to 146Nd/144Nd=0.7219 and 86Sr/88Sr=0.1194, respectively. Detailed analytical methods are described in Chen & Jahn (Reference Chen and Jahn2002). Measurements were corrected using standards NBS 607, with a 87Sr/86Sr ratio of 1.20032±28 (2σ), and BCR-1, with a 143Nd/144Nd ratio of 0.512626±9 (2σ). The analytical precision of the measurements was ~99% for the 87Rb/86Sr ratio, and ~99.5% for the 147Sm/144Nd ratio. Two-stage depleted mantle (T DM) Nd model ages were calculated using a 143Nd/144Nd ratio of 0.513151 and a 147Sm/144Nd ratio of 0.21357 for the present-day depleted mantle, and an average crustal 147Sm/144Nd ratio of 0.118 (Jahn & Condie, Reference Jahn and Condie1995).
4.4. In situ zircon Hf isotopic analyses
In situ zircon Hf isotopic analyses of granite samples were conducted at Nanjing University, using a Neptune MC-ICP-MS equipped with a 193 nm laser. A laser energy of 100 mJ and a repetition rate of 10 Hz were used for all measurements; spot sizes were 32–63 µm. Raw count rates for 172Yb, 173Yb, 175Lu, 176(Hf+Yb+Lu), 177Hf, 178Hf, 179Hf, 180Hf and 182W were collected, and corrections for the isobaric interference 176Lu and 176Yb on 176Hf were precisely determined. 176Lu was calculated using the 175Lu value, while mean Yb value obtained for each spot was applied when correcting for 176Yb interference; the 176Yb/172Yb ratio was assumed to be 0.5887 (Iizuka & Hirata, Reference Iizuka and Hirata2005). Measured 176Hf/177Hf and 176Lu/177Hf ratios for zircon standard 91500 were 0.282294±15 (2σ, n=20) and 0.00031, respectively. This 176Hf/177Hf value agrees well with the accepted 176Hf/177Hf ratios of 0.282302±8 and 0.282306±8 (2σ), obtained using the solution method (Goolaerts et al. Reference Goolaerts, Mattielli, Jong, Weis and Scoates2004). The notations ε Hf(t), f Lu/Hf, and T DM are defined as in Yang et al. (Reference Yang, Wu, Wilde, Xie, Yang and Liu2007), with single-stage Hf model ages interpreted from positive ε Hf(t) values.
5. Results
5.1. Major and trace elements
The granite samples are strongly peraluminous, with A/CNK ratios of 1.08–1.53 (molar Al2O3/CaO+Na2O+K2O; Fig. 6b). They are composed of 66.54–76.13% SiO2, 2.90–5.64% K2O, 1.24–3.86% Na2O, 1.05–4.90% TFe2O3, 0.02–0.14% MnO, 0.41–1.40% MgO, 0.01–0.67% TiO2 and 0.01–0.22% P2O5. Mg# [Mg/(Mg+Fe)] values of these granites range from 28.69 to 53.33 (Table 1). All of the studied samples plot within the ‘subalkalic granite’ field on the (Na2O+K2O) v. SiO2 plot (Fig. 6a).

Figure 6. Chemical classification diagrams for the studied granite samples. (a) Total alkali (K2O+Na2O) v. SiO2 diagram (compositional fields from Middlemost, 1994); (b) A/NK v. A/CNK diagram (Maniar and Piccoli, 1989).
Table 1. The major chemical compositions (in wt%) and calculated parameters of the five studied granites

Note: A=Al2O3, C=CaO, N=Na2O, K=K2O (all in molar proportion), Mg*=100 Mg2+/(Mg2++Fe2+).
Table 2. Trace element compositions (in ppm) of the five studied granites

The total rare earth element (REE) contents of the five studied granites are quite high, ranging from 92.01 to 282.05 ppm, and all samples show a characteristic enrichment in light REEs relative to heavy REEs, with (La/Yb)N ratios ranging from 4.26 to 11.75 (Fig. 7b). In addition, the granites show strong depletions in Nb and Ta, which are typical characteristics of subduction-related magmas (Fig. 7a).

Figure 7. Primitive mantle-nomalized trace element spidergram patterns and chondrite-normalized REE patterns of the studied granites: (a, b) the Mandalehai granites; (c, d) the Huiteng granite; (e, f) the Tuergen granite; (g, h) the Zhagesitai granite; and (i, j) the Zhepujileke granite.
5.2. Zircon U–Pb ages
Zircon grains in our granite samples are typically pale yellow, transparent, subhedral to euhedral, and 80–100 μm in size. All zircons exhibit bright cathodoluminescence, with clear concentric oscillatory zones (Fig. 4). Th concentrations of all the analysed zircons vary from 36 to 1801 ppm, while U concentrations range from 83 to 2202 ppm, yielding relatively high Th/U ratios (0.33–1.82). Zircon xenocrysts are common in the CL images; the Th/U ratios of these xenocrysts range from 0.65 to 0.93, suggesting that they are magmatic zircons. After excluding discordant ages, LA-ICP-MS U–Pb dating of zircons yielded ages of 408.2±4.8 Ma for the Mandalehai granites, 327.7±5.6 Ma for the Huiteng granites, 400.1±3.5 Ma for the Tuergen granites, 449.8±4.5 Ma for the Zhagesitai granites and 433±5.9 Ma for the Zhepujilieke granites (Fig. 5; Table 3). The U–Pb ages of the xenocrysts yielded a wider range of ages, from 1294 to 913 Ma, indicating zircon crystallization in the source magma (Table 3).
Table 3. U-–Pb dating results of the studied five granites

5.3. Sr–Nd isotopic compositions
All samples yielded high initial 87Sr/86Sr ratios (0.714998–0.743426), and negative ε Nd(t) values (−3.3 to −0.9; Fig. 8a; Table 4). The uniformly negative ε Nd(t) values and wide range of initial 87Sr/86Sr ratios in the samples can be interpreted in the context of a two-component mixing model (Han et al. Reference Han, Wang, Jahn, Hong, Kagami and Dun1997). The depleted mantle Nd model (TDM2) yields quite old ages for the studied granites, ranging from 1120 to 1290 Ma.

Figure 8. Plot of (a) ε Nd(t) v. Age (Ma) and (b) ε Hf(t) v. Age (Ma) for the studied granites from the Chinese Altai.
Table 4. Sr–Nd isotopic compositions of the five studied granites

εNd(t) values were calculated using present-day (147Sm/144Nd)CHUR=0.1967 and (143Nd/ 144Nd)CHUR=0.512638.
T DM values were calculated using present-day (147Sm/144Nd)DM=0.2137 and (143Nd/144Nd) DM=0.51315.
5.4. Zircon Hf isotopic compositions
The measured Hf isotopic compositions of the five studied granite samples are shown in Figure 8b; analytical results are listed in Table 5. The ε Hf(t) values were calculated using the U–Pb ages of the zircons, and range from −7.4 to +8.0. The depleted mantle Hf model ages (T DM2) of the granite samples range from 948 to 1801 Ma. However, the ε Hf(t) values and depleted mantle Nd model ages (T DM2) of the zircon xenocrysts are distinct; ε Hf(t) values range from −30.6 to −13.7, and T DM2 ages range from 2190 to 3243 Ma.
Table 5. Zircon Hf isotopic compositions of the five studied granites

The 176Hf/177Hf and 176Lu/177Hf ratios of chondrite and depleted mantle at present are 0.282772 and 0.0332, 0.28325 and 0.0384; (176Lu/177Hf)LC=0.019; λ=1.867×10−11a−1; t = crystallization time of zircon.
εHf(t)={[(176Hf/177Hf)S−(176Lu/177Hf)S×(eλt−1)]/[(176Hf/177Hf)CHUR−(176Lu/177Hf)CHUR×(eλt−1)]−1}×10000.
T DM1=1/λln{[(176Hf/177Hf)S−(176Hf/177Hf)DM]/[(176Lu/177Hf)S−(176Lu/177Hf)DM]+1}.
T DM2=t+1/λln{[(176Hf/177Hf)S−(176Hf/177Hf)DM]/[(176Lu/177Hf)LC−(176Lu/177Hf)DM]+1}
6. Discussion
6.1. Peraluminous granitic magmatism in the Chinese Altai
The zircon U–Pb dating results for the five peraluminous granites in this study reveal that these intrusions were emplaced between 449 and 327 Ma. Synchronous felsic volcanism is also apparent in the form of peraluminous rhyolites in the study area, which have yielded U–Pb zircon ages of 406 to 412 Ma (Chai et al. Reference Chai, Mao, Dong, Yang, Liu, Geng and Zhang2009; Long et al. Reference Long, Yuan, Sun, Xiao, Zhao, Wang and Cai2010; Wang et al. Reference Wang, Yuan, Long, Sun, Xiao, Zhao, Cai and Jiang2011; Cai et al. Reference Cai, Sun, Yuan, Xiao, Zhao, Long and Wu2012). Other peraluminous and S-type granites have also been dated, and given comparable zircon U–Pb ages (507 Ma to 392 Ma; Tong et al. Reference Tong, Wang, Hong, Dai, Han and Liu2007; Cai et al. Reference Cai, Sun, Yuan, Zhao, Xiao, Long and Wu2011a; Zhang et al. Reference Zhang, Liu, Santosh and Zhang2016). Peraluminous granitic magmatism appears to have occurred over a long period in the Chinese Altai, spanning from 507 to 327 Ma.
All of the studied peraluminous granites plot in the ‘VAG+syn-COLG’ field on the Nb v. Y and ‘VAG’ field Rb v. (Y+Nb) plots (Fig. 9a, b), and in the ‘Classical island arc’ and ‘Normal arc magmas’ field on the (La/Yb)N v. (Yb)N and Sr/Y v. Y plots (Fig. 10a, b), indicating that these granites are most likely petrogenetically associated with arc magmatism. These results agree well with the subduction tectonic setting inferred for the Chinese Altay during the Palaeozoic (Yuan et al. Reference Yuan, Sun, Xiao, Li, Chen, Lin, Xia and Long2007; Sun et al. Reference Sun, Yuan, Xiao, Long, Xia, Zhao, Lin, Wu and Kröner2008; Cai et al. Reference Cai, Sun, Yuan, Zhao, Xiao, Long and Wu2011a,b; Xiao et al. Reference Xiao, Zhang, Shi, Su, Sakyi, Hu and Zhang2011; Xiao & Santosh, Reference Xiao and Santosh2014; Zhang et al. Reference Zhang, Liu, Santosh and Zhang2016).

Figure 9. Trace element diagrams for tectonic discrimination: (a) Nb v. Y and (b) Rb v. Y+Nb.

Figure 10. Trace element discrimination diagrams: (a) (La/Yb)N v. (Yb)N; (b) Sr/Y v. Y.
6.2. Petrogenesis
6.2.1. Magma sources
Previous studies have suggested that peraluminous granites are mainly generated by the partial melting of metasedimentary rocks such as metapelite and metagreywacke, with the possible addition of metaigneous rocks such as orthogneiss, and amphibolite (Miller, Reference Miller1985; Patiño Douce & Johnston, Reference Patiño Douce and Johnston1991; Sylvester, Reference Sylvester1998). All of the peraluminous granites included in this study are compatible with the ‘sediment involvement’ trend on the Ba/Th v. (La/Sm)N plot, indicating a substantial addition of sediments to the source magmas (Fig. 11a). This interpretation is supported by the presence of aluminium-saturated minerals in thin sections, i.e. cordierite, garnet, muscovite and sillimanite (Fig. 3). Previous studies have proposed that the strongly peraluminous felsic rocks in the Chinese Altai were derived from thermal melting of local metasediments (e.g. the Habahe Group), due to heating from the asthenosphere upwelling through a slab window (Cai et al. Reference Cai, Sun, Yuan, Zhao, Xiao, Long and Wu2011b; Yu et al. Reference Yu, Sun, Long, Li, Zhao, Kröner, Broussolle and Yang2016). However, the low zircon saturation temperature (537–765°C; Fig. 11b) and the existence of residual zircon does not support a high-temperature magmatic event in the Chinese Altai. The metasedimentary rocks of the Habahe Group are overwhelmingly Neoproterozoic to Early Palaeozoic in age, with a prominent detrital zircon age peak around 465–542 Ma (with zircon ε Hf(t) values of −25 to +15; Jiang et al. Reference Jiang, Sun, Zhao, Yuan, Xiao, Xia, Long and Wu2011b). Therefore, the oldest zircon xenocrysts in the studied granites, with U–Pb ages from 1294 to 913 Ma and ε Hf(t) values ranging from −30.6 to −13.7 (T DM2=2190–3243 Ma), are likely to be exotic.
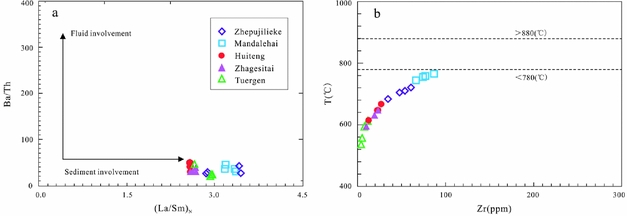
Figure 11. Trace element discrimination diagrams: (a) Ba/Th v. (La/Sm)N; and (b) diagram for variations in zircon saturation temperatures for the studied granites from the Chinese Altai.
Oceanic sediments mixing into the source magma via dehydration melting are expected to have a composition reflecting their origin as weathering products of upper continental crust (Asahara et al. Reference Asahara, Tanaka, Kamioka, Nishimura and Yamazaki1999; Bayon et al. Reference Bayon, German, Boella, Milton, Taylor and Nesbitt2002). The studied granites are characterized by high initial 87Sr/86Sr ratios (0.714998–0.743426), negative ε Nd(t) values (−3.3 to −0.9) and ε Hf(t) values (−30.6 to −13.7), all of which are typical geochemical characteristics of oceanic sediments (Asahara et al. Reference Asahara, Tanaka, Kamioka, Nishimura and Yamazaki1999; Bayon et al. Reference Bayon, German, Boella, Milton, Taylor and Nesbitt2002). Additionally, the Nd/Hf ratios of these granites are both relatively high and quite variable (from 4.26 to 43.57), which is typical of Indian Ocean sediments (Nd/Hf=6–42, n=9; Ben Othman, White & Patchett, Reference Ben Othman, White and Patchett1989; Gasparon & Varne, Reference Gasparon and Varne1998; Plank & Langmuir, Reference Plank and Langmuir1998). These various lines of evidence support the presence of an oceanic sediment component in the magma sources.
However, the isotopic features of Sr and Nd in whole-rock samples, and Hf in zircons, strongly suggest a heterogeneous source. Previous studies have suggested that mantle-derived components or juvenile crust played an important role in the generation of granites in the CAOB (Sengör, Natal'in & Burtman, Reference Sengör, Natal'in and Burtman1993; Hu et al. Reference Hu, Jahn, Zhang, Chen and Zhang2000; Jahn, Wu & Chen, Reference Jahn, Wu and Chen2000b; Windley et al. Reference Windley, Alexeiev, Xiao, Kroner and Badarch2007). The positive zircon ε Hf(t) values (+0.24 to +8.01), young Hf model ages (905–1317 Ma) and high Mg# values (28.69–53.33) of the studied granites strongly suggest a mantle-derived component. In particular, the characteristics of the zircon Hf isotopic system are consistent with those of other Palaeozoic arc granites from the region (ε Hf(t)=−1.4 to +12.9, TDM2=765–2122 Ma, n=14; Cai et al. Reference Cai, Sun, Yuan, Zhao, Xiao, Long and Wu2011b; Wang et al. Reference Wang, Yuan, Long, Sun, Xiao, Zhao, Cai and Jiang2011; Lv et al. Reference Lv, Zhang, Tang and Guan2012). Therefore, we proposed that the granites were derived from mixed magmas, incorporating materials from both the partial melting of subducting oceanic sediments and the overlying mantle wedge.
Sr is a more sensitive indicator of fluid introduction than Hf and Nd (Woodhead et al. Reference Woodhead, Hergt, Davidson and Eggins2001; Münker et al. Reference Münker, Worner, Yogodzinski and Churikova2004; Turner et al. Reference Turner, Handler, Bindeman and Suzuki2009). The initial 87Sr/86Sr ratios of the studied granites (0.706718–0.739768) demonstrate a wider range than the initial 143Nd/144Nd ratios (0.512120–0.512232), possibly reflecting the addition of fluids into the magma sources (Zhang et al. Reference Zhang, Liu, Santosh and Zhang2016). However, as shown by the plots of Ba/Th v. (La/Sm)N (Fig. 11a) and Ba/Nb v. Ba/La (Fig. 12a), the granites are not plotted on the ‘fluid involvement’ or ‘fluid enrichment’ lines. This indicates that, although aqueous fluids were important in the generation of initial magma, they did not significantly alter their chemical compositions.

Figure 12. Trace element discrimination diagrams: (a) Ba/Nb v. Ba/La; (b) Nb/Th v. Nb/La.
6.2.2. Assimilation of continental crust and fractional crystallization
Previous studies have suggested that correlations between selected major and trace element ratios (such as La/Sm, Nb/La, Th/Ta, Sm/Nd, SiO2/MgO and Nb/U) can be used to assess the effects of crustal contamination in magmas (Yin et al. Reference Yin, Chen, Yuan, Yu, Xiao, Long, Li and Sun2015). No correlation is observed in plots of Sm/Nd v. Nb/La, La/Sm v. Nb/La or Nb/U v. SiO2/MgO (not shown), ruling out the possibility of significant crustal contamination. On the Nb/Th v. Nb/La plot (Fig. 12b), peraluminous granites plot well away from the ‘crustal contamination’ trend, indicating that assimilation of the continent crust was minimal. This is further supported by the uniform ε Nd(t) values and low zircon saturation temperatures (537–765°C) of the peraluminous granites, which indicate that the magmas did not suffer from assimilation of the surrounding rocks during its intrusion. Therefore, we conclude that the crustal affinities of the peraluminous granites are mainly attributable to melting of oceanic sediments rather than crustal assimilation.
The peraluminous granites show a wider range in MgO contents (0.41–1.40 wt%) and Mg# (28.69–53.33), suggesting that their precursor magmas probably underwent a higher degree of fractional crystallization. The Rb/Sr v. Sr and Ba v. Sr plots (Fig. 13) indicate that fractional crystallization of plagioclase, K-feldspar and biotite played a dominant role in the evolution of the peraluminous magmas.

Figure 13. Trace element discrimination diagrams: (a) Rb/Sr v. Sr; (b) Ba v. Sr.
6.3. Decoupling of Nd and Hf isotopic systems
All samples included in this study showed significant Nd–Hf decoupling (Fig. 8). This signal may have been inherited from the magma source, or may be the result of disequilibrium melting processes (Su et al. Reference Su, Qin, Lu, Sun and Sakyi2015; Yu et al. Reference Yu, Sun, Long, Li, Zhao, Kröner, Broussolle and Yang2016). Melts may show higher 176Hf/177Hf ratios than the source rock if dissolution of zircons is incomplete during partial melting, because the very low Lu/Hf ratio of zircon can result in low, non-radiogenic 176Hf/177Hf ratios (e.g. Tang et al. Reference Tang, Wang, Shu, Wang, Yang and Gopon2014; Yu et al. Reference Yu, Sun, Long, Li, Zhao, Kröner, Broussolle and Yang2016). The high Nd/Hf ratios (4.87–37.70) and low Hf concentrations (0.75–3.87 ppm) of the studied granites can be explained by sequestration of Hf in residual zircon, which would lower the concentrations of Hf relative to Nd in melts. Zircon xenocrysts often appear in CL images of the studied granites (Fig. 4), suggesting that the decoupled Nd–Hf isotopic features may be inherited from disequilibrium melting processes.
These results suggest that the studied granites were produced by partial melting of subducting oceanic sediments, as well as the overlying mantle wedge. The old Hf model ages (T DM2=2190−3243 Ma) and negative ε Hf(t) values (−30.6 to −13.7) of these xenocrysts suggest a crustal affinity, and a possible derivation from oceanic sediments. Numerous studies have reported significant disequilibrium in the Sr, Nd and Pb isotopic systems during sedimentary or crustal anatexis (e.g. Hogan & Sinha, Reference Hogan and Sinha1991; Hammouda, Reference Hammouda1994; Knesel & Davidson, Reference Knesel and Davidson1996; Tommasini & Davies, Reference Tommasini and Davies1997; Davies & Tommasini, Reference Davies and Tommasini2000). The rate of zircon dissolution is controlled by several factors, including zircon solubility, temperature, zircon crystal size, and the melt and solid phase composition (e.g. Ayres & Harris, Reference Ayres and Harris1997; Zeng, Asimow & Saleeby, Reference Zeng, Asimow and Saleeby2005a; Zeng, Saleeby & Asimow, Reference Zeng, Saleeby and Asimow2005b; Farina & Stevens, Reference Farina and Stevens2011). A variable rate of zircon dissolution in a single magma source may result in variable Hf isotopic compositions in different batches of melts (Tang et al. Reference Tang, Wang, Shu, Wang, Yang and Gopon2014). Tollstrup & Gill (Reference Tollstrup and Gill2005) calculated Zr solubility in a granitic melt using the parameters given by Watson & Harrison (Reference Watson and Harrison1983), and suggested that for subducted sediments containing 65–165 ppm Zr, temperatures must be lower than 780°C for any zircon to survive. This finding was supported by Johnson & Plank (Reference Johnson and Plank1999), who reported sediment melting at 780–825°C for peraluminous to metaluminous material at 3–4 GPa.
Based on this study, we conclude that zircons from oceanic sediments may have survived in the crystal phase during partial melting, then served as nuclei for recrystallization at the low zircon saturation temperature of the studied granites (Fig. 11b). During the melting process, a significant amount of 177Hf was retained at the source by residual zircons (Tang et al. Reference Tang, Wang, Shu, Wang, Yang and Gopon2014), while the 176Hf/177Hf ratio of the melts became correspondingly elevated, and decoupled from the 143Nd/144Nd ratio. Residual zircon can explain the wide range of ε Hf(t) values and Hf model ages seen in these granites, which cannot represent the geochemistry of the primary magma.
In contrast with 177Hf, which can be retained at the source, Nd concentrations cannot be altered by residual zircon during the melting of oceanic sediments. Furthermore, the model ages of the studied granites and their uniform ε Nd(t) values suggest only minor assimilation of continental crust and fluids during magmatic processes. Consequently, whole-rock Nd isotopic ratios likely represent mixing processes in the parent magmas of the studied granites.
6.4. Implications for the recycling of oceanic sediments
The growth of the Chinese Altai was driven by subduction processes along the margins of the Palaeo-Asian ocean, from the Cambrian (or earlier) through the Devonian (Xiao et al. Reference Xiao, Windley, Badararch, Li, Sun, Qin and Wang2004, 2008; Yuan et al. Reference Yuan, Sun, Xiao, Li, Chen, Lin, Xia and Long2007; Sun et al. Reference Sun, Yuan, Xiao, Long, Xia, Zhao, Lin, Wu and Kröner2008, 2009; Cai et al. Reference Cai, Sun, Yuan, Zhao, Xiao, Long and Wu2010, 2011a; Su et al. Reference Su, Qin, Sakyi, Li, Yang, Sun, Tang, Liu, Xiao and Malaviarachchi2011, 2012;). Recent studies have suggested that ridge subduction was probably the dominant tectonic control in the geodynamic evolution of the Chinese Altai. When an actively spreading ocean ridge is dragged into a subduction zone, the oceanic slabs on both sides cease to grow (Dickinson & Snyder, Reference Dickinson and Snyder1979; Thorkelson, Reference Thorkelson1996; Cai et al. Reference Cai, Sun, Yuan, Zhao, Xiao, Long and Wu2010; Santosh & Kusky, Reference Santosh and Kusky2010). High-temperature metamorphism and several types of magmatism will commence, as diverse components of the mantle and downgoing slab are melted by upwelling of hot asthenosphere through the slab window (Geng et al. Reference Geng, Sun, Yuan, Xiao, Xian, Zhao, Zhang, Wong and Wu2009; Zhao et al. Reference Zhao, Xiong, Wang, Bai and Qiao2009; Jiang et al. Reference Jiang, Sun, Zhao, Yuan, Xiao, Xia, Long and Wu2010; Yin et al. Reference Yin, Yuan, Sun, Long, Zhao and Geng2010; Zhang et al. Reference Zhang, Zhao, Santosh, Wang, Dong and Shen2010).
However, the low zircon saturation temperature (537–765°C) of the studied granites does not support the type of high-temperature magmatic event that would be produced by the upwelling of hot asthenosphere in a ridge subduction setting. This is further supported by the appearance of the zircon xenocrysts in CL images (Fig. 4). In addition, Early to Middle Palaeozoic A-type granites and high-temperature metamorphic rocks are scarce in the Chinese Altai (Yuan et al. Reference Yuan, Sun, Xiao, Li, Chen, Lin, Xia and Long2007; Sun et al. Reference Sun, Yuan, Xiao, Long, Xia, Zhao, Lin, Wu and Kröner2008; Cai et al. Reference Cai, Sun, Yuan, Zhao, Xiao, Long and Wu2011a,b, 2012, Wang et al. Reference Wang, Yuan, Long, Sun, Xiao, Zhao, Cai and Jiang2011; Lv et al. Reference Lv, Zhang, Tang and Guan2012), which also suggests ‘cold’ magmatism during this interval. Experimental studies (Osamu, Reference Osamu1995; Karsten, Klein & Sherman, Reference Karsten, Klein and Sherman1996) have revealed that melts formed in a subduction ridge setting typically develop under low-pressure conditions, whereas the major element composition of the studied granites implies that they formed in a high-pressure environment, most likely a normal subduction zone rather than a ridge subduction setting. Therefore, the ridge subduction model is not supported by the studied granites.
It is generally accepted that subduction is a ‘high-pressure’ but relatively ‘low-temperature’ tectonic process, and under most circumstances subducting crustal rocks are too cold to melt. However, recent modelling studies, combined with an improved understanding of the chemistry and petrography of subduction-zone fluids and melts, indicate subduction zones likely meet the conditions for deep slab melt, so long as free fluid is available at sub-arc depths. (Pearce et al. Reference Pearce, Kempton, Nowell and Noble1999; Kempton et al. Reference Kempton, Pearce, Barry, Fitton, Langmuir and Christie2002; Nebel et al. Reference Nebel, Münker, Nebel-Jacobsen, Kleine, Mezger and Mortimer2007; Pearce, Kempton & Gill, Reference Pearce, Kempton and Gill2007; Spandler & Pirard, Reference Spandler and Pirard2013). Subduction zones are sites where chemical components are transferred from the downgoing slab back to the surface (Spandler & Pirard, Reference Spandler and Pirard2013). Experiments by Grassi & Schmidt (Reference Grassi and Schmidt2011) and Hofmann (Reference Hofmann1997) indicated that subducting slab components can be mechanically or diffusively mix into the ambient mantle, or can return to the continent crust, in the form of chemical components within arc magmas.
Previous studies have proposed that only 15% of sediment-derived Hf originating in subducting volcanoclastic debris is transported back to the crust via arc magmatism, whereas ~85% is introduced into the mantle. Since a large amount of Hf can be retained in residual zircons under low-temperature conditions, the proportion of Hf returning to the crust is likely to be lower than 15%. Therefore, Hf may not be an effective tracer in sediment recycling (Chauvel et al. Reference Chauvel, Marini, Plank and Ludden2009; Handley et al. Reference Handley, Turner, Macpherson, Gertisser and Davidson2011; Zhang et al. Reference Zhang, Liu, Santosh and Zhang2016). In contrast, the concentration and isotopic composition of Nd in arc magmas cannot be altered by residual zircon effects. As a result, Nd may more reliably constrain the relative proportion of recycled oceanic sediment in mixed-source arc magmas. In this study, we have outlined a model to explain how Hf is transferred out of the subducting slab. The significant decoupling between Nd and Hf isotopic compositions in the studied peraluminous granites from the Chinese Altai shows that partial melting of oceanic sediment is a major factor controlling the relationship between the two isotopic systems in island arc environments.
Based on these results, we consider oceanic sediment recycled back into the arc crust was an important component of the source magmas for the studied granites. Approximately 40% of exposed rocks in the Altai Orogenic Belt are granite, suggesting that over 40% of continental growth in the orogenic belt is attributable to felsic arc magmatism. This demonstrates that oceanic sediment recycling was an important process in the continental growth of the Chinese Altai, an aspect that has been completely overlooked in previous studies.
7. Conclusions
1. The studied peraluminous granites in the Chinese Altai were emplaced between 449 and 327 Ma. Their parental magmas might be derived from the partial melting of oceanic sediments and the associated mantle wedge in an active subduction zone.
2. A significant amount of 177Hf was retained by residual zircons in the subducting slab, leading to significant decoupling of the Hf and Nd isotopic systems in the peraluminous granites. Whole-rock Nd isotopic ratios can faithfully reflect mixing processes within the parent magma of the peraluminous granites.
3. Oceanic sediment recycling may have played an important role in the crustal growth in the Chinese Altai during the early and middle Palaeozoic.
Acknowledgements
We would like to express our gratitude to Prof. Shengrong Li for his constructive reviews, which significantly improved the manuscript. This work was financially supported by the China University of Petroleum (Beijing) Science Foundation (Project No. 2462014YJRC031), the National Science Foundation of China (Project No. 41502209) and Chinese State Program 973 (Project No. 2015CB250901).