1. Introduction
The Aravalli–Delhi Fold Belt (ADFB) in NW India preserves a record of a protracted period of crustal evolution spanning from ~3.3 Ga to 1.0 Ga (Fig. 1a; Crawford & Compston, Reference Crawford and Compston1970; Sivaram & Odom, Reference Sivaraman and Odom1982; MacDougall et al. Reference MacDougall, Gopalan, Lugmair and Roy1983; Choudhary, Gopalan & Sastry, Reference Choudhary, Gopalan and Sastry1984; Sarkar, Barman & Corfu, Reference Sarkar, Barman and Corfu1989; Volpe & Mcdougall, Reference Volpe and Macdougall1990; Gopalan et al. Reference Gopalan, Macdougall, Roy and Murli1990; Sastry, Reference Sastry1992; Guha & Garkhal, Reference Guha and Garkhal1993; Goswami, Wiedenbeck & Roy, Reference Goswami, Wiedenbeck, Roy, Lanphere, Dalrymple and Turin1994; Tobisch et al. Reference Tobisch, Collerson, Bhattachrya and Mukhopadhyay1994; Wiedenbeck & Goswami, Reference Wiedenbeck and Goswami1994; Sharma, Reference Sharma, Sinha-Roy and Gupta1995; Lopez et al. Reference Lopez, Mukhopadhyay, Bhattacharyya and Tobisch1996; Wiedenbeck, Goswami & Roy, Reference Wiedenbeck, Goswami and Roy1996 a,Reference Wiedenbeck, Goswami and Roy b ; Roy & Kröner, Reference Roy and Kröner1996; Fareeduddin & Kröner, Reference Fareeduddin, Kröner and Paliwal1998; Mukhopadhay et al. Reference Mukhopadhyay, Bhattacharyya, Chattopadhyay, Lopez and Tobisch2000; Deb & Thorpe, Reference Deb, Thorpe, Deb and Goodfellow2004; Roy et al. Reference Roy, Kröner, Bhattacharya and Rathore2005, Reference Roy, Kröner, Rathore, Laul and Purohit2012; Buick et al. Reference Buick, Allen, Pandit, Rubatto and Hermann2006, Reference Buick, Clark, Rubatto, Hermann, Pandit and Hand2010; Kaur et al. Reference Kaur, Chaudhri, Biju-Sekhar and Yokoyama2006, Reference Kaur, Chaudhri, Raczek, Kröner and Hofmann2007, Reference Kaur, Chaudhri, Raczek, Kroner, Hofmann and Okrusch2011 a,b; Bhowmik, Bernhardt & Dasgupta, Reference Bhowmik, Bernhardt and Dasgupta2010). The NNE-trending polymetamorphic ADFB sandwiched between the Marwar Craton in the west and the Meso/Neoarchaean Bundelkhand Craton in the east records two major orogenies, i.e. (i) the 1.8–1.7 Ga Aravalli Orogeny and the (ii) Grenvillian-age Delhi Orogeny (Volpe & Mcdougall, Reference Volpe and Macdougall1990; Kaur et al. Reference Kaur, Chaudhri, Biju-Sekhar and Yokoyama2006, Reference Kaur, Chaudhri, Raczek, Kröner and Hofmann2007, Reference Kaur, Chaudhri, Raczek, Kroner, Hofmann and Okrusch2011 a,b; Buick et al. Reference Buick, Allen, Pandit, Rubatto and Hermann2006, Reference Buick, Clark, Rubatto, Hermann, Pandit and Hand2010; Bhowmik, Bernhardt & Dasgupta, Reference Bhowmik, Bernhardt and Dasgupta2010). The Palaeoproterozoic Aravalli Orogeny is marked by (i) the emplacement of rift-related A-type granitoids around Khetri and Jasrapura in the North Delhi Fold Belt (NDFB; Kaur et al. Reference Kaur, Chaudhri, Raczek, Kröner and Hofmann2007, Reference Kaur, Chaudhri, Raczek, Kroner and Hofmann2009, Reference Kaur, Chaudhri, Raczek, Kroner, Hofmann and Okrusch2011 a), (ii) mid-crustal amphibolite-facies metamorphism of the Rajpura–Dariba and the Pur–Banera schist belts (Hazarika, Upadhyay & Mishra, Reference Hazarika, Upadhyay and Mishra2013; Ozha et al. Reference Ozha, Mishra, Hazarika, Jeyagopal and Yadav2016) and (iii) sillimanite-facies crustal anatexis along the NE–SW-trending Sandmata Complex (SC) and Mangalwar Complex (MC, constituting BGC-II; Heron, Reference Heron1953; Buick et al. Reference Buick, Allen, Pandit, Rubatto and Hermann2006, Reference Buick, Clark, Rubatto, Hermann, Pandit and Hand2010; Saha et al. Reference Saha, Bhowmik, Fukuoka and Dasgupta2008; Bhowmik, Bernhardt & Dasgupta, Reference Bhowmik, Bernhardt and Dasgupta2010; Roy et al. Reference Roy, Kröner, Rathore, Laul and Purohit2012). Besides, detrital zircons from the Alwar quartzite unit (the oldest stratigraphic unit in the NDFB) and the greenschist-facies schists of the Aravalli Supergroup around Jhamarkotra (Fig. 1b) yield ages of ~1.8–1.7 Ga (Kaur et al. Reference Kaur, Chaudhri, Raczek, Kroner, Hofmann and Okrusch2011 b; McKenzie et al. Reference McKenzie, Hughes, Myrow, Banerjee, Deb and Planavsky2013). These sedimentary successions are believed to have formed in rift-related basins under extensional tectonic regimes (Kaur et al. Reference Kaur, Chaudhri, Raczek, Kroner, Hofmann and Okrusch2011 b; McKenzie et al. Reference McKenzie, Hughes, Myrow, Banerjee, Deb and Planavsky2013). Earlier tectonic models proposed for crustal evolution of the ADFB include the (i) two Wilson cycles model of Sinha-Roy (Reference Sinha-Roy and Roy1988) and Vijaya Rao et al. (Reference Vijaya Rao, Rajendra Prasad, Reddy and Tewari2000), (ii) one Wilson cycle model of Sugden, Deb & Windley (Reference Sugden, Deb, Windley and Naqvi1990), (iii) ensialic orogenesis by Roy (Reference Roy and Naqvi1990) and Sharma (Reference Sharma, Sinha-Roy and Gupta1995), and (iv) inversion tectonics by Verma & Greiling (Reference Verma and Greiling1995). Bhowmik & Dasgupta (Reference Bhowmik and Dasgupta2012) proposed that rift-related granite magmatism and sedimentation in the NDFB and crustal anatexis in the SC and MC occurred as a result of switching between subduction and extensional tectonics (in back-arc settings) during the Palaeoproterozoic Aravalli Orogeny.

Figure 1. (a) General tectonic map of Peninsular India (modified after Radhakrishna, 1989) showing the location of Archaean cratons and Proterozoic mobile belts. Abbreviations: ADFB – Aravalli–Delhi Fold Belt; AC – Aravalli Craton; BuC – Bundelkhand Craton; SC – Singhbhum Craton; BC – Bastar Craton; EDC – Eastern Dharwar Craton; WDC – Western Dharwar Craton; CITZ – Central Indian Tectonic Zone; CGC – Chotanagpur Gneissic Complex; SPGC – Shillong Plateau Gneissic Complex; EGMB – Eastern Ghats Mobile Belt; SIG – South Indian Granulite terrain; HOB – Himalayan Orogenic Belt; DV – Deccan Volcanics; 1 – Mahandi Rift; 2 – Godavari Rift; 3 – Closepet Granite. Study area in the ADFB is shown in the box. (b) Geological map of Aravalli–Delhi Fold Belt, NW India (modified after Roy, Reference Roy1988; Roy & Jakhar, Reference Roy and Jakhar2002) showing ages of important events. Age data sources: 1 – Deb & Sarkar (Reference Deb and Sarkar1990), Kaur et al. (Reference Kaur, Chaudhri, Raczek, Kröner and Hofmann2007, Reference Kaur, Chaudhri, Raczek, Kroner and Hofmann2009, Reference Kaur, Zeh, Chaudhri, Gerdes and Okrusch2011); 2 – Biju-Sekhar et al. (Reference Biju-Sekhar, Yokoyama, Pandit, Okudaira, Yoshida and Santosh2003); 3 – Deb & Thorpe (Reference Deb, Thorpe, Deb and Goodfellow2004); 4 – Mukhopadhyay et al. (Reference Mukhopadhyay, Bhattacharyya, Chattopadhyay, Lopez and Tobisch2000); 5 – Deb, Thorpe & Krstic (Reference Deb, Thorpe and Krstic2002); 6 – Roy et al. (Reference Roy, Kröner, Bhattacharya and Rathore2005); 7 – Buick et al. (Reference Buick, Allen, Pandit, Rubatto and Hermann2006); 8 – Sarkar, Barman & Corfu (Reference Sarkar, Barman and Corfu1989), Fareeduddin & Kröner (Reference Fareeduddin, Kröner and Paliwal1998), Bhowmik, Bernhardt & Dasgupta (Reference Bhowmik, Bernhardt and Dasgupta2010); 9 – Wiedenbeck, Goswami & Roy (Reference Wiedenbeck, Goswami and Roy1996 a); 10 –Gopalan et al. (Reference Gopalan, Macdougall, Roy and Murli1990); 11 – Wiedenbeck, Goswami & Roy (Reference Wiedenbeck, Goswami and Roy1996 b), Roy & Kröner (Reference Roy and Kröner1996); 12 – Gopalan et al. (Reference Gopalan, Macdougall, Roy and Murli1990), Wiedenbeck & Goswami (Reference Wiedenbeck and Goswami1994), Roy & Kröner (Reference Roy and Kröner1996); 13 – Roy & Kröner (Reference Roy and Kröner1996); 14 – Bhowmik, Bernhardt & Dasgupta (Reference Bhowmik, Bernhardt and Dasgupta2010); 15 – Ozha et al. (Reference Ozha, Mishra, Hazarika, Jeyagopal and Yadav2016). Abbreviation: BGC – Banded Gneissic Complex. BGC-I and BGC-II after Heron (Reference Heron1953), respectively, include the Archaean gneissic terrain and the central gneissic terrain.
Reworking of the ~1.8–1.7 Ga gneissic crust in the SC and MC occurred at ~12–14 kbar pressures and >750°C as a result of collisional tectonics during the Grenvillian Delhi Orogeny (Buick et al. Reference Buick, Allen, Pandit, Rubatto and Hermann2006, Reference Buick, Clark, Rubatto, Hermann, Pandit and Hand2010; Bhowmik, Bernhardt & Dasgupta, Reference Bhowmik, Bernhardt and Dasgupta2010; Bhowmik & Dasgupta, Reference Bhowmik and Dasgupta2012). The studies are based on the anatectic high-grade SC and the Rampura–Agucha anatexites in the MC, the non-anatectic amphibolite-facies Rajpura–Dariba and Pur–Banera schist belts, and the granitoids of the NDFB and SDFB (Fig. 1b). However, the metamorphic evolutionary history of the vast swathes of anatectic migmatites exposed, albeit poorly, eastwards of the Rampura–Agucha anatexites and the non-anatectic schists of the Pur–Banera, and extending up to the Hindoli Supergroup rocks in the east and the Archaean Berach granite (Fig. 2) remain largely unknown. Naha & Roy (Reference Naha and Roy1983) suggested the mesoscale structures in these anatectic migmatites are similar to the ones in the Sandmata and the Mangalwar complexes. Roy & Jakhar (Reference Roy and Jakhar2002) considered these gneisses to be the high-grade equivalents of rocks in the Aravalli Supergroup. However, in the absence of metamorphic characterization and geochronology in these anatectic migmatites, the relevance of these migmatites in the Proterozoic crustal evolution history of the Aravalli craton remains speculative. In this study based on field geological studies, P–T estimation obtained using thermodynamic modelling of reaction equilibria and monazite dating, we address if these anatectic migmatites shared the polycyclic history recorded in the SC and MC migmatites. A consequence of this study is to examine the eastward extent of Grenvillian-age tectonism along the intensely tectonized Delwara Lineament formed owing to the welding of the MC with the SC in the west.

Figure 2. Geological map of the central and eastern Aravalli–Delhi Fold Belt (modified after Geological Survey of India quadrangle maps) showing lithology, sample locations (along different transects) and pervasive foliation data. Abbreviations: GF – Grenvillian Front; GG – granite gneiss; CG – calc-silicate gneiss; SG – garnet–biotite–sillimanite gneiss; MG – muscovite gneiss; TG – tourmaline–muscovite gneiss. Delhi Supergroup on this map is the stratigraphic name for the South Delhi Fold Belt shown in Figure 1.
2. Geological setting
The Aravalli Supergroup of rocks in the Bhilwara Sector are distributed along two NE–SW-trending subparallel belts: (i) an eastern belt consisting of the low-grade volcano-sedimentary units of the Hindoli Group, and (ii) a western belt consisting of the amphibolite-facies mica schist–quartzite sequence of the Rajpura–Dariba and Pur–Banera schist belts in the south and migmatites in the north (Fig. 2), whose stratigraphic relationships are not established owing to lack of geochronological data. The eastern low-grade belt is separated from the Vindhyan Supergroup of rocks in the east by the Great Boundary Fault and from the migmatite belt in the west by the Jahazpur lineament (Fig. 2; Gupta et al. Reference Gupta, Arora, Mathur, Iqballuddin Prasad, Sahai and Sharma1980; Sinha-Roy, Malhotra & Mohanty, Reference Sinha-Roy, Malhotra and Mohanty1998; Verma, Reference Verma1999). The Jahazpur lineament has been identified as a thrust belt comprising the amphibolite-facies lithologies of the Jahazpur Belt along the northern margin of the low-grade metamorphic rocks of the Hindoli Group (Sinha-Roy & Malhotra, Reference Sinha-Roy and Malhotra1989; Tiwari et al. Reference Tiwari, Divakar rao, Narayana, Dixit, Madhavrao, Murthy, Rajendra prasad, Reddy, Venkateswarlu, Rao, Mishra and Gupta1998). The low-grade units of the Hindoli Group form a volcano-sedimentary succession comprising interlayered quartzite, pelite, carbonates, lava flows and tuffs of both felsic and mafic volcanic rocks (Gupta et al. Reference Gupta, Arora, Mathur, Iqballuddin Prasad, Sahai and Sharma1980; Bose & Sharma, Reference Bose and Sharma1992).The mica schist–quartzite sequence in the southern part of the western belt forms a NE–SW-trending folded belt extending from the Rajpura–Dariba in the southwest to Pur–Banera in the northeast (Fig. 2; Hazarika, Upadhyay & Mishra, Reference Hazarika, Upadhyay and Mishra2013; Ozha et al. Reference Ozha, Mishra, Hazarika, Jeyagopal and Yadav2016). Mica schists from the belt comprise a garnet–biotite–muscovite–kyanite–quartz–feldspar–chlorite assemblage (Hazarika, Upadhyay & Mishra, Reference Hazarika, Upadhyay and Mishra2013; Ozha et al. Reference Ozha, Mishra, Hazarika, Jeyagopal and Yadav2016).
In the course of this study along transects across the anatectic migmatites of the Bhilwara Sector (Fig. 2), garnet–biotite–sillimanite gneisses appear to be the most dominant lithologies, albeit poorly exposed, extending from Bhilwara in the southwest, Agucha in the northwest, and Kekri and Deoli in the arc between the northeast and southeast, respectively (Figs 1b, 2). These migmatites are locally interleaved with amphibolites and calc-silicate gneisses and intruded by variably deformed porphyritic granites (this study; Roy, Somani & Sharma, Reference Roy, Somani and Sharma1981; A. B. Roy, Hindusthan Zinc Ltd, unpub. project report, 2000). Lithological variations are best observed along the Deoli–Shahpura transect (Fig. 2). Along this transect, outcrops of anatectic migmatites were observed at two localities at Deoli, BJ-28 (25°38.301ʹN, 75°6.879ʹE) and BJ-30 (25°34.162ʹN, 74°50.087ʹE; Fig. 2). The migmatite near Deoli occurs at the contact of the phyllite–quartzite-carbonates of the Jahazpur thrust belt. The gneissic banding in the migmatite is defined by muscovite–biotite–tourmaline-bearing mesocratic bands alternating with quartz–feldspar–tourmaline-bearing leucocratic bands (Fig. 3a). At ~22 km SW, small scattered outcrops of muscovite–biotite–garnet-bearing migmatite (BJ-28) occur (Fig. 3b). The migmatite layering in these rocks is composed of garnet–muscovite–biotite-bearing mesocratic bands and quartz–feldspar-bearing leucocratic bands. The first outcrop of biotite–garnet–sillimanite–feldspar-bearing gneiss (BJ-30) occurs about 40 km SW of BJ-28, near Shahpura (Fig. 2). At this locality, the migmatitic banding is formed by garnet–biotite–sillimanite–quartz-bearing mesocratic bands and quartz–feldspar-bearing leucocratic bands (Fig. 3c). We have chosen these two anatectic migmatite samples (BJ-28 and BJ-30) to understand the spatial variations, if any, in P–T conditions of anatexis in the two localities, or whether bulk compositional variations were responsible for muscovite melting and biotite melting in the two localities. Monazite age dating was carried out on these samples to determine if partial melting in both localities was coeval or not.

Figure 3. Field photographs showing migmatitic bandings in (a) tourmaline-bearing muscovite gneiss occurring around Deoli, (b) garnet–muscovite–biotite-bearing gneiss (BJ-28) and (c) field photograph of garnet–biotite–sillimanite–feldspar-bearing gneiss (BJ-30). Mineral abbreviations used in this figure are after Kretz (1983). Same abbreviations are also used for other figures and tables. Hammer for scale is 38 cm long; hand lens for scale is 4.5 cm long.
3. Results
3.a. Petrography and mineral chemistry
3.a.1. Garnet–muscovite–biotite gneiss (BJ-28)
The mesocratic domain in the sample comprises garnet, muscovite, biotite and quartz (Fig. 4a). Garnet porphyroblasts contain inclusion trails (Si) of quartz and biotite. Pervasive foliation in the matrix (Se) is formed by oriented muscovite and biotite (Fig. 4a). Inclusion trails (Si) in garnet porphyroblasts occur at a high angle with respect to the matrix foliation. Coarse muscovite grains overgrow matrix foliation (Fig. 4b). Leucocratic domains consist mainly of quartz and K-feldspar, and minor plagioclase. Foliation formed by muscovite wraps around a coarse K-feldspar in the leucosome (Fig. 4b). Overgrowths of muscovite on the foliation and replacement of feldspar by muscovite are also common. Microstructural observations like inclusions of biotite and quartz in the garnet porphyroblasts indicate breakdown of biotite and quartz during garnet formation (Fig. 4a). Additionally, the presence of muscovite in the leucocratic bands indicates participation of muscovite in the following partial melting reaction:


Figure 4. (a) BSE image of mesocratic band of BJ-28 in which inclusion trails in garnet (Si) are formed by biotite + quartz and Se marks the pervasive foliation in the matrix formed by biotite + muscovite + quartz. (b) Thin-section photograph of leucocratic band of BJ-28 showing a coarse potash feldspar grain wrapped around by muscovite forming foliation Se. (c, d) BSE images of BJ-30 showing presence of garnet + biotite + sillimanite + feldspar in the mesocratic band (c & d) and quartz–potash feldspar-bearing leucocratic bands (c). Inclusion trails in garnet porphyroblasts (Si) are formed by biotite + sillimanite + quartz; biotite fills up fractures in garnet (blue arrows); biotite + sillimanite-bearing symplectites replace potash feldspar in leucocratic bands and also occur at the margins of garnet porphyroblasts against the leucocratic bands (yellow arrows). (e) BSE image of BJ-30 showing occurrence of plagioclase at margins of garnet. (f–h) Thin-section images of leucocratic bands of BJ-30 showing perthite, antiperthite texture and recrystallization of quartz and feldspar grains. Note presence of inclusion trails (Si) of biotite + sillimanite in an antiperthite grain.
Muscovite-defined foliation wrapping around garnet porphyroblasts in the mesocratic bands and coarse K-feldspars in the leucocratic bands indicate that formation of the foliation post to melting and replacement of K-feldspar by muscovite is due to retrogression.
Garnet porphyroblasts are mostly solid solutions of pyrope and almandine with minor grossular and spessartine contents (Table 1a; analytical methods are given in the Appendix). Two sets of data from core to rims (marked as profile 1 and 2 in Table 1a) of a garnet porphyroblast indicate compositional homogeneity from core (away from any biotite inclusion) to the outer rims (Alm0.81–0.82Prp0.11–0.10Grs0.04–0.03Sps0.05–0.04 and XMg ~0.12–0.11) with pyrope contents decreasing significantly at the edge of the garnet (XPrp ~0.08) against matrix biotite (Table 1a). XMg of the garnet edge at the contact with matrix biotite is ~0.08–0.09, which is ~2–3 mol.% lower than that in the garnet core. On the contrary, compositions of the garnet core at the contacts with biotite inclusions are similar to those at the edges of the garnet against matrix biotite (Table 1a). XMg values of matrix biotite show no significant variation from cores (~0.34–0.37) to rims (~0.34–0.36; Table 1a). Titanium contents of the individual matrix biotite grains do not show any variation from their cores (~1.672–1.738 apfu based on 11 O) to rims (~1.689–1.723 apfu based on 11 O; Table 1a). XMg values of the biotite inclusions in garnet porphyroblasts (~0.43–0.46) are higher than those of the matrix biotite porphyroblasts (Table 1b). Titanium contents of the biotite inclusions vary from 0.135 to 0.156 (Table 1a). Si contents of muscovite grains from the foliation domains vary from 3.085–3.132 apfu (based on 11 O), their total aluminium contents varying from 2.640–2.768 apfu (based on 11 O; Table 1a).
Table 1a. Representative mineral chemical analyses of garnet from BJ-28

I – inclusion; M – matrix; C – core; R – rim; IR – inner rim; OR – outer rim; E – edge; Fr – fracture; F – foliation; ^ – against; XMg=Mg/(Mg+Fe2+); XSps =Mn/(Mn+Ca+Fe2++Mg); XAlm=Fe2+/(Fe2++Mg+Ca+Mn); XPrp=Mg/(Fe2+ +Ca+Mn+Mg); XGrs=(Al/(Fe3++Ti+Al+Cr))*(Ca/(Fe2++Mg+Ca+Mn)).
Table 1a. (Continued) Representative mineral chemical analyses of biotite from BJ-28

Table 1a. (Continued) Representative mineral chemical analyses of muscovite from BJ-28

3.a.2. Garnet–biotite–sillimanite gneiss (BJ-30)
The sample consists of garnet, biotite, sillimanite, quartz and feldspars (Fig. 4c). Garnet porphyroblasts are elongated, with inclusion trails formed by oriented biotite–sillimanite and globular quartz grains (Figs 4c–d). Leucocratic bands consist of plagioclase–K-feldspar, quartz and sillimanite (Fig. 4c). Plagioclase commonly occurs at the contact of garnet porphyroblasts and matrix biotite (Fig. 4e). Important textural features in the leucocratic bands include the presence of perthite and antiperthite with sillimanite- and biotite-bearing inclusion trails (Si; Fig. 4f). Plagioclase at the interstices of coarse quartz grains is interpreted to be crystallized melt films (Fig. 4g). Extensive recrystallization of coarse quartz and feldspar grains is noted in the leucocratic bands (Fig. 4h).
The presence of biotite, sillimanite and quartz inclusions in garnet porphyroblasts, and antiperthites in the leucosomes point to the following partial melting reaction during peak metamorphism:

The formation of biotite- and sillimanite-bearing symplectites replacing K-feldspar (yellow arrows, Fig. 4c) and biotite replacing garnet along fractures (blue arrows, Fig. 4c) indicate retrogression. Symplectites of biotite + sillimanite also occur at the contact of peritectic garnet and the leucocratic layers (yellow arrows, Fig. 4c).
Garnet porphyroblasts in the mesocratic layers are mostly solid solutions of pyrope and almandine with negligible spessartine and very low grossular contents (Table 1b; analytical methods are given in the Appendix). Garnet porphyroblasts show no or only very weak compositional variation from cores (Prp0.20–0.22 Alm0.73–0.75 Sps0.01Grs0.04–0.05 and XMg ~0.21–0.23) to outer rims (Prp0.22Alm0.73Sps0.01Grs0.04 and XMg ~0.23; Table 1b). At the contacts with biotite inclusions and at the edges against matrix biotite grains, garnet compositions show a decrease in pyrope contents (Prp0.16–0.19 Alm0.75–0.79 Sps0.01Grs0.04–0.05 and XMg ~0.17–0.20; Table 1b). Titanium contents of biotite inclusions in garnet porphyroblasts vary from 0.189–0.223 apfu and their XMg values range from 0.66–0.69 (Table 1b). Titanium contents of cores and rims of matrix biotite grains at contacts with garnet rims vary from 0.211–0.239 and 0.202–0.231 apfu, respectively (Table 1b). XMg of the cores and rims of matrix biotite grains at contacts with garnet grains vary from 0.59–0.61 and 0.61–0.65, respectively (Table 1b). XAn of plagioclase grains within the leucocratic layers (0.33–0.37) are higher than those of the plagioclase which occurs in the garnet fractures (0.25–0.27; Table 1b).
Table 1b. Representative mineral chemical analyses of garnet from BJ-30

I – inclusion; M – matrix; C – core; IR – inner rim; OR – outer rim; E – edge; Fr – fracture; ^ – against; XMg=Mg/(Mg+Fe2+); XSps=Mn/(Mn+Ca+Fe2++Mg); XAlm=Fe2+/(Fe2++Mg+Ca+Mn); XPrp=Mg/(Fe2++Ca+Mn+Mg); XGrs=(Al/(Fe3++Ti+Al+Cr))*(Ca/(Fe2++Mg+Ca+Mn)).
Table 1b. (Continued) Representative mineral chemical analyses of biotite from BJ-30
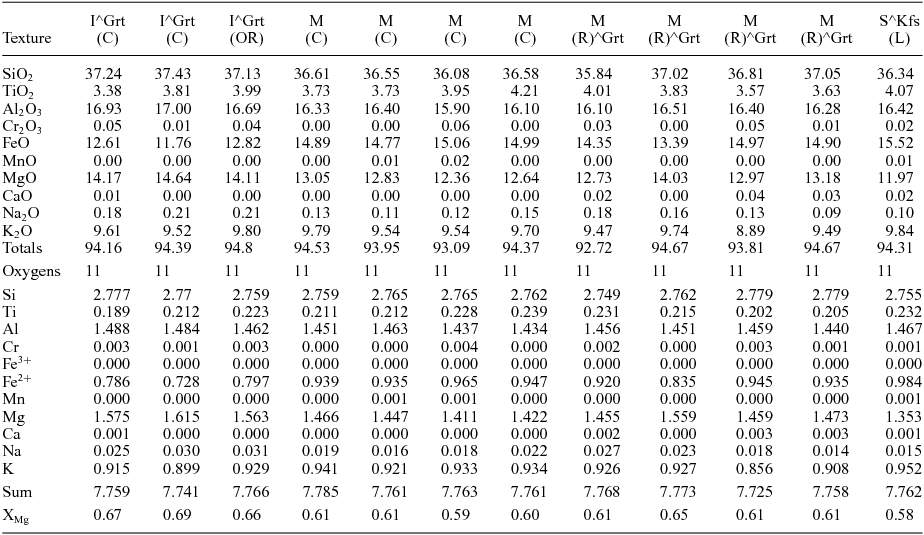
I – inclusion; M – matrix; C – core; R – rim; OR – outer rim; L – leucosome; S – symplectite; ^ – against; XMg=Mg/(Mg+Fe2+).
Table 1b. (Continued) Representative mineral chemical analyses of feldspar from BJ-30

I – inclusion; M – matrix; C – core; R – rim; Fr – fracture; ^ – against; _ – within; L – leucosome; XAn=Ca/(Ca+Na+K).
3.b. P–T conditions of anatexis
3.b.1. Thermodynamic modelling
Microtextures and mineral assemblages of the investigated gneisses (BJ-28 and BJ-30) of the Aravalli Supergroup rocks (shown in Fig. 4a–h) point to muscovite and/or biotite breakdown reactions during extensive anatexis and garnet formation. The investigated rocks show migmatitic textures where melt migrated from the reaction sites and segregated as distinct domains and layers resulting in complete recrystallization and obliteration of the palaeosomes (Fig. 3a–c). This imparts difficulty in estimation of the palaeosome bulk composition for pseudosection analysis. In order to address this problem, in previous petrological studies of migmatites, two different methods have been adopted for constraining the bulk composition for pseudosection analyses. In one method, pseudosections have been constructed with various hypothetical bulk compositions of the pelites (White, Powell & Holland, Reference White, Powell and Holland2001, Reference White, Powell and Holland2007; Johnson & Brown, Reference Johnson and Brown2004), and in the second method, bulk compositions were reconstituted from mineral modes and compositions from different microdomains (Kelsey et al. Reference Kelsey, White, Wilson and Quinn2003). Melt reintegration is problematic for the migmatite samples BJ-28 and BJ-30 owing to strong deformation and the cumulate origin of some of the leucocratic layers or domains (e.g. Johnson & Brown, Reference Johnson and Brown2004). So we have considered modelling of the melting reactions by constructing P–T pseudosections using the average hypothetical pelite bulk composition of White, Powell & Holland (Reference White, Powell and Holland2001) in the model system Na2O–CaO–K2O–FeO–MgO–Al2O3–SiO2–H2O (NCKFMASH) in order to estimate the (i) temperature of melting, and (ii) P–T ranges of stability of the assemblages formed during peak or near-peak metamorphism for each rock. Additionally, in order to estimate P–T conditions of equilibration of restite phases during peak and post-peak metamorphic conditions, P–T pseudosections have been constructed from the refractory bulk compositions (determined by XRF) of the mesocratic bands of BJ-28 and BJ-30. Analytical conditions of XRF analysis are given in the Appendix.
Pseudosections have been constructed using PERPLE_X (Connolly, Reference Connolly2005) and the thermodynamic database of Holland & Powell (Reference Holland and Powell1998), modified in 2004. Solution models of phases used for pseudosection modelling are: (i) garnet: hybrid model of Holland & Powell (Reference Holland and Powell1998) and Engi & Wersin (Reference Engi and Wersin1987); (ii) biotite: White, Powell & Holland (Reference White, Powell and Holland2007); (iii) muscovite: hybrid model of Coggon & Holland (Reference Coggon and Holland2002) and Auzanneau et al. (Reference auzanneau, Schmidt, Vielzeuf and Connolly2010); (iv) feldspar: Benisek, Dachs & Kroll (Reference Benisek, Dachs and Kroll2010), Newton, Charlu & Kleppa (Reference Newton, Charlu and Kleppa1980) and Waldbaum & Thompson (Reference Waldbaum and Thompson1968); (v) melt: hybrid model of Holland & Powell (Reference Holland and Powell2001) and White, Powell & Holland (Reference White, Powell and Holland2001).
3.b.2. Results of P–T pseudosection analyses
At first, P–T pseudosections were constructed using the hypothetical metapelite bulk composition of White, Powell & Holland (Reference White, Powell and Holland2001). Both the ternary feldspar model of Benisek, Dachs & Kroll (Reference Benisek, Dachs and Kroll2010) and plagioclase model of Newton, Charlu & Kleppa (Reference Newton, Charlu and Kleppa1980), and K-feldspar model of Waldbaum & Thompson (Reference Waldbaum and Thompson1968) were used to model formation of perthite and/or antiperthite and the occurrence of plagioclase and K-feldspar as separate phases either in the mesocratic or in the leucocratic layers of BJ-28 and BJ-30 (Fig. 5a–d). In the P–T pseudosections in Figure 5a, b the melt-in curve has positive slopes with slopes becoming very steep at >8 kbar. At the pressure range of 4–12 kbar, which has been chosen for the P–T pseudosections, the partial melting reaction initiates by muscovite breakdown at lower temperatures and by biotite breakdown at higher temperatures (Fig. 5a–d).

Figure 5. (a) P–T pseudosection for the average pelite composition used in White, Powell & Holland (Reference White, Powell and Holland2001). The bulk composition in mol.% is Al2O3=30.66, FeO=23.74, MgO=12.47, CaO=0.97, Na2O=1.94, K2O=9.83 and H2O=20.39; SiO2 has been taken as a saturated component. The ternary feldspar solution model of Benisek, Dachs & Kroll (Reference Benisek, Dachs and Kroll2010) has been used for this P–T pseudosection. Abundances of melt are shown as isopleths.
The aluminosilicate-absent biotite + muscovite + garnet + quartz + feldspar + melt-bearing assemblage in BJ-28 formed during peak metamorphism is stable at pressures >10 kbar (Fig. 5a, c). XMg isopleths of garnet (0.08–0.14) and biotite inclusions in garnet (0.46) corresponding to the values measured in BJ-28 intersect at temperatures of ~725°C and at lower pressures (~7.5 kbar) in the fields where sillimanite is stable with biotite + melt (~12 modal%) + feldspar(s) + muscovite + garnet + quartz (Fig. 5b, d). Such a disparity in mineral assemblage may be attributed to some difference in hypothetical bulk composition and the real palaeosome bulk composition. Maximum melt abundance at the temperature calculated from the isopleth thermobarometry of garnet and biotite is ~20 mol.% (Fig. 5a, c).

Figure 5. (b) Compositional isopleths of garnet and biotite in the P–T pseudosection of (a).

Figure 5. (c) P–T pseudosection for the average pelite composition used in White, Powell & Holland (Reference White, Powell and Holland2001) with feldspar model of Newton, Charlu & Kleppa (Reference Newton, Charlu and Kleppa1980) and Waldbaum & Thompson (Reference Waldbaum and Thompson1968) to model occurrence of plagioclase and potash feldspar as separate phases. (d) Compositional isopleths of garnet, biotite and feldspar in the P–T pseudosection of (c).
The peak assemblage of BJ-30 is biotite + garnet + sillimanite + plagioclase + K- feldspar + melt + quartz (Fig. 4c). This assemblage is stable in the P–T range of 4–9 kbar and 740–825°C, in the P–T pseudosection constructed from the hypothetical bulk composition of White, Powell & Holland (Reference White, Powell and Holland2001; Fig. 5a, c). XMg isopleths of garnet and biotite corresponding to the measured compositions (garnet ~0.21 and biotite ~0.6–0.7; Table 1b) plot at ~780–800°C along the biotite-out curve within the garnet + biotite + melt + feldspar + quartz-bearing field and possibly indicate near-peak temperature (T Max; Fig. 5b, d). Maximum pressure in BJ-30 during peak metamorphism is estimated from the intersections of the compositional isopleths with the sillimanite to kyanite transition curve, i.e. at ~8 kbar (Fig. 5b, d). This pressure is similar to that noted for the Palaeoproterozoic sillimanite-facies partial melting event recorded from the SC by Saha et al. (Reference Saha, Bhowmik, Fukuoka and Dasgupta2008). The computed melt volume at the estimated peak conditions (P Max= 8 kbar and T Max=780–800°C) is ~42% (Fig. 4a, b).
As the second approach, P–T pseudosections were constructed from the bulk composition of the mesocratic domains of the rock which represent the refractory bulk compositions after extensive melt segregation. The bulk rock compositions determined by X-ray fluorescence analysis are given in Table 2. The refractory bulk composition of the muscovite-bearing BJ-28 has higher XMg (0.43) than that of the biotite-bearing BJ-30 (0.20) and of the average hypothetical bulk composition of the pelitic rocks (0.26). Al2O3 and K2O contents of the hypothetical bulk composition are higher than that of BJ-28, BJ-30 and the hypothetical bulk composition (Appendix Table A1). On the contrary its CaO content is lower than that of BJ-28 and BJ-30 (Appendix Table A1).
Table 2. X-ray fluorescence analyses of sample in weight per cent

Pseudosections constructed from the XRF bulk composition of the mesocratic band of BJ-28 (in the NCKFMASH system using the identical water content to that of White, Powell & Holland Reference White, Powell and Holland2001) is shown in Figure 6a, b. P–T pseudosections demonstrate that melting reactions occur from 650°C at 4 kbar to c. 600°C at 12 kbar, i.e. within a very narrow temperature range, and at >600–650°C vapour-absent melting is prevalent (Fig. 6a). The peak assemblage of biotite + garnet + quartz + melt is stable between 7–12 kbar and 600–800°C (Fig. 6a). Garnet XMg isopleths corresponding to those measured from a garnet porphyroblast (both core and rims) plot along the 650–700°C range (Fig. 6b). XMg isopleths similar to the measured composition of the biotite inclusions (~0.43–0.46; Table 1a) in the garnet core also plot in the same temperature range. However, the isopleths corresponding to the composition of the matrix biotite (~0.34) do not match this stability field (Fig. 6b). Si isopleths for muscovite values (~3.09), similar to the measured composition, intersect with isopleths of garnet and biotite at ~8 kbar and 700°C (Fig. 6b). The calculated abundance of melt at ~650–700°C is ~30% (Fig. 6a). Melt volume percentages at the peak P–T conditions are ~30–31% (Fig. 6a), which is much higher than the melt escape threshold value of ~20 vol.% estimated by Vigneresse, Barbey & Cuney (Reference Vigneresse, Barbey and Cuney1996). As the bulk composition used for the pseudosection analyses is refractory, it may be predicted that the protolith (expected to be more fertile) would have yielded higher volumes (>30%) of melt leading to complete migration and segregation of the melt as has been observed in the outcrop (Fig. 3b).

Figure 6. (a) P–T pseudosections in NCKFMASH system from bulk composition of mesocratic band of BJ-28. The bulk composition in mol.% is SiO2=69.43, Al2O3=11.12, FeO=6.53, MgO=5.00, CaO=2.087, Na2O=2.42, K2O=3.39 and H2O=20.39. Isopleths show melt abundance. (b) Compositional isopleths of garnet and biotite in the P–T pseudosection of (a).
The P–T pseudosection for BJ-30 from the bulk composition derived by XRF analysis (Table 2) and calculated in the (Na2O–CaO–K2O–FeO–MgO–Al2O3–SiO2–H2O–TiO2) NCKFMASHTi system ( Fig. 7a, b), depicts P–T conditions for water-saturated partial melting. The peak metamorphic assemblage garnet + biotite ± plagioclase + melt + sillimanite + quartz + ilmenite according to the pseudosection (Fig. 7a) is stable at 4–7 kbar and 725–780°C. Isopleths of garnet XMg corresponding to the measured values (~0.20; Table 1b) plot at ~755°C (Fig. 7b).

Figure 7. (a) Pseudosections in NCKFMASHTi system from bulk composition of mesocratic domain of BJ-30. The bulk composition in mol.% is SiO2=54.57, TiO2=1.45, Al2O3=13.29, FeO=21.89, MgO=5.36, CaO=1.41, Na2O=0.16, K2O=1.23 and H2O=20.39. Melt abundance is shown as isopleths. (b) Compositional isopleths of garnet, biotite and feldspar in the P–T pseudosection of (a).
3.b.3. Conventional geothermometry
Garnet–biotite thermometry has been conducted from the samples BJ-30 and BJ-28, in order to estimate temperatures of garnet formation during partial melting and post-peak re-equilibration. The thermometry has been applied at a ~8 kbar reference pressure, estimated from the pseudosection analysis. Additionally, the titanium-in-biotite geothermometer of Henry, Guidotti & Thomson (Reference Henry, Guidotti and Thomson2005) was also applied to determine temperatures of partial melting and cooling.
Garnet–biotite thermometry from the garnet cores and biotite inclusions in BJ-28 yields temperatures of ~500–516°C and from the garnet edges against matrix biotite rims ~507–560°C (Table 3a). Temperatures obtained from titanium contents in biotite inclusions in garnet porphyroblasts range between ~638 and 659°C and those from matrix biotite grains between 638 and 672°C (Table 3b). Although the highest temperature obtained from titanium-in-biotite thermometry (~672°C) is close to the temperatures of muscovite melting and garnet formation at ~710°C obtained from pseudosection analyses (Fig. 6a, b), temperatures obtained from garnet–biotite thermometry are significantly lower (more than ~100°C), which is interpreted as a result of strong diffusion rates of Fe and Mg at supra-solidus conditions.
Table 3a. Garnet–biotite thermometry at reference pressure 8 kbar

I – inclusion; M – matrix; C – core; E – edge; OR – outer rim; R – rim; ^ – against.
Table 3b. Titanium-in-biotite thermometry after Henry, Guidotti & Thomson (Reference Henry, Guidotti and Thomson2005)

I – inclusion; M – matrix; C – core; S – symplectite; OR – outer rim; ^ – against; L – leucosome.
For sample BJ-30, temperatures obtained from garnet–biotite thermometry from the garnet core compositions and biotite inclusions of ~500–509°C are similar to those obtained from the compositions of garnet edges and matrix biotite rims (~500–515°C; Table 1a). Titanium-in-biotite thermometry conducted from biotite inclusions in garnet yields temperatures ranging from 743–765°C and from the matrix biotite grains yields temperatures of ~741–754°C (Table 1b). A temperature range of ~740–765°C obtained from titanium contents in biotite grains is close to ~780°C, obtained from garnet isopleth thermometry in the pseudosection (Fig. 7a, b). On the contrary, garnet–biotite thermometry yields the minimum blocking temperature between garnet and biotite at ~500°C similar to that obtained in BJ-28.
4. Monazite dating
Both studied metapelite samples are rich in monazite. In sample BJ-28, monazite is found only in the matrix either enclosed by biotite, muscovite (Fig. 8d) and quartz, or intergrown with apatite. Monazite from sample BJ-30 also most commonly occurs in the fine-grained, quartz–biotite, quartz–K-feldspar or sillimanite matrix (Fig. 8g), but some grains (mnz7, 11–14) occur enclosed by garnet (Fig. 8h). Monazite crystals are mostly anhedral to subhedral, typically 40–100 μm (BJ-28) or 80–130 μm in size (BJ-30). Back-scattered electron (BSE) images from both samples show that they are either homogeneous or may contain a darker (Y-enriched) phase in the centres (Fig. 8a, b, g). Several larger grains from BJ-28 show a more complicated structure with bright patches enriched in Th.

Figure 8. BSE images of the dated monazites and their enclosing minerals.
Monazites were dated by electron microprobe Cameca SX-100 in Bratislava using the ‘age calibration’ approach (Petrík & Konečný, Reference Petrík and Koneč ný2009), which includes spot analyses yielding apparent ages. These are then corrected against five age standards dated isotopically by SHRIMP and TIMS methods. Analytical settings were set as follows: counting times for Pb 150 s, Th 45 s, U 75 s, Y 45 s, and for all other elements 25–35 s; sample current 100–130 nA; beam diameter as large as possible, up to 10 μm, but mostly 3 μm to get maximum counts and to minimize surface damage. Standards used were: rare earth elements (REEs) and Y from phosphates XPO4, Th from ThO2, Pb from galena, U from UO2, Ca and Si from wollastonite, and Al from Al2O3. All errors are given as 2σ. Weighted isochrons and deconvolution histograms were calculated using Isoplot 4.15 (Ludwig Reference Ludwig2001).
Almost 100 spots were analysed in c. 40 crystals from two migmatite samples; analytical data for the dated monazites are shown in Table 4. While sample BJ-30 contains a simple population of monazites with ages around 1740 Ma the other sample BJ-28 is more complicated, showing at least two populations (c. 1740 and 800 Ma). The six spots with apparent ages between 1100 and 1400 Ma are interpreted as mixed ages of the spots between old and young domains. The monazites from BJ-30 (n=27 points) give a weighted mean age of 1741±7 Ma or U/Pb v. Th/Pb isochron centroid age of 1746±14 Ma (Cocherie & Albarede, Reference Cocherie and Albarede2001). The increased value of MSWD=3.6 indicates that the U–Th–Pb system was partly disturbed, as indicated by the presence of three spots with higher ages 1780–1827 Ma. Omitting these spots the isochron gives an age of 1739±9 Ma with MSWD 1.7 (Fig. 9). Both isochrons are concordant. The monazites from the other sample BJ-28 record more than one event. Dating yielded two apparent ages, higher at c. 1700 Ma and lower at c. 780 Ma. The older monazites form centres of crystals forming their dominant part. The U/Pb v. Th/Pb isochron gives a centroid age of 1688±25 Ma, but a high MSWD=11 indicates disturbance of the system, presumably due to younger overprint.
Table 4. Analytical data for the dated monazites (samples BJ-28, BJ-30)

Notes: The units in the table are wt. % (Th, U, Y, Pb). Pbcorr, Ucorr values corrected against standards, for details see methods. Th* equivalent Th, errors are 2 sigma.

Figure 9. U/Pb–Th/Pb isochron ages of monazites from sample BJ-30.
The lower ages are found at the rims of larger grains; some small monazites show only the lower age (mnz 5, 21, both enclosed in muscovite). The weighted average is 782±13 Ma for n=24 spots and a high MSWD=7.5. A similar age is also obtained from the U/Pb v. Th/Pb isochron (788±20 Ma). The apparently inhomogeneous population may involve domains with mixed ages as shown by the deconvolution histogram (Fig. 10).

Figure 10. Histogram of the monazite ages from sample BJ-28.
5. Discussion
5.a. P–T conditions of crustal anatexis
To constrain the P–T conditions of partial melting in the migmatites, we carried out pseudosection analyses using the hypothetical bulk metapelite composition of White, Powell & Holland (Reference White, Powell and Holland2001; Fig. 5a–d). The results of the pseudosection analyses are compared with those obtained from the P–T pseudosections computed using the bulk compositions of mesocratic layers in the samples (Figs 6a, b, 7a).
Isopleth thermometry from the bulk composition of BJ-30 yields a T Max of ~750°C at 8 kbar necessary for garnet stability (Fig. 7b). The result is similar to the corresponding value obtained from the pseudosection analyses from the hypothetical metapelite bulk composition of White, Powell & Holland (Reference White, Powell and Holland2001) and from titanium-in-biotite thermometry (Table 3b). The maximum temperature obtained from the isopleth thermometry of the biotite composition in Figure 5b, d is ~800°C, ~50°C higher than that obtained from garnet thermometry (~750°C). Hence, we conclude that the P–T conditions for stability of the peak assemblage garnet + sillimanite + biotite + quartz + feldspar + melt were ~8 kbar and 750–800°C. Textural observations from BJ-30 indicate that biotite–sillimanite–quartz inclusions in the garnet (Fig. 4c) were the phases participating in the prograde reactions. On the other hand, matrix biotite, sillimanite and quartz (Fig. 4c–h) formed during retrogression. Thus, we propose a clockwise P–T path (tight-loop) with decompression during retrogression (Fig. 7b). A decrease in pressure to ~6.5 kbar is derived from the intersection of garnet isopleths with XAn isopleths of plagioclase occurring in the garnet fractures (Fig. 7b; Table 1c).
Pseudosection analyses from the BJ-28 bulk composition indicate stability of the muscovite–biotite–garnet–quartz–feldspar–melt-bearing peak assemblage in water-undersaturated conditions at ~700°C and above 6.5 kbar pressure (Fig. 6a). Although the temperature estimated from the isopleth thermometry is in agreement with that obtained from titanium-in-biotite thermometry (Table 3b), it is about ~100–150°C higher than that predicted from the garnet–biotite thermometry (~500–560°C; Table 3a). A lower temperature range for garnet–biotite thermometry (using conventional thermometers and THERMOCALC) as compared to the single element titanium-in-biotite thermometer and isopleths thermometry is attributed to compositional resetting between garnet and biotite during post-peak cooling in the presence of melt. Hence, the minimum temperature predicted for retrogression was ~500°C. However, owing to compositional homogeneity of the phases it is difficult to estimate whether the post-peak cooling was isobaric or accompanied by decompression.
5.b. Late Palaeoproterozoic anatexis in the gneisses
Metamorphic monazites in the foliation domains in garnet–biotite–muscovite gneiss (BJ-28) occurring in the eastern part of the study area yield two age populations, i.e. monazite cores ~1.74 Ga, and monazite rims ~0.8 Ga (Figs 9, 10). In the biotite–sillimanite–garnet-bearing gneiss (BJ-30) in the southwestern part, monazites in the matrix as well as monazites sequestered in garnet porphyroblasts (Figs 8e, f) yield a single, well-constrained age population of ~1.73 Ga (Fig. 9). The ~1.73 Ga age coincides with the timing of mid-crustal anatexis and charnockite emplacement in the SC, and A-type granite magmatism in the NDFB (Roy et al. Reference Roy, Kröner, Bhattacharya and Rathore2005; Buick et al. Reference Buick, Allen, Pandit, Rubatto and Hermann2006; Saha et al. Reference Saha, Bhowmik, Fukuoka and Dasgupta2008; Kaur et al. Reference Kaur, Chaudhri, Raczek, Kroner, Hofmann and Okrusch2011 a,b). Evidently, anatexis in the gneisses in the eastern part of the ADFB was contemporaneous with the Late Palaeoproterozoic high-grade metamorphism and felsic magmatism reported in the western part of the ADFB. This work, therefore, provides a framework to demonstrate that the ~1.8–1.7 Ga high-grade metamorphism recorded in the BGC-II comprising the Sandmata and the Mangalwar complexes is not restricted to the west of the Delwara Lineament, but extends within the anatectic gneisses of the Aravalli Supergroup until the Jahazpur Belt (Figs 1b, 2). Hence, the BGC-II and the anatectic gneisses of the Aravalli Supergroup (of Roy & Jakhar, Reference Roy and Jakhar2002) shared a common tectono-metamorphic history in Late Palaeoproterozoic time.
The rarity of 1.0–0.9 Ga ages – obtained in a couple of spots on monazite grains (Fig. 9) – in the two anatectic gneisses, suggests the effect of Grenvillian-age tectonics is prominent in the Sandmata and the Mangalwar complexes in the western part of the ADFB and along the Delwara Lineament but does not extend eastwards of Pur–Banera (Buick et al. Reference Buick, Allen, Pandit, Rubatto and Hermann2006, Reference Buick, Clark, Rubatto, Hermann, Pandit and Hand2010; Bhowmik, Bernhardt & Dasgupta, Reference Bhowmik, Bernhardt and Dasgupta2010; Hazarika, Upadhyay & Mishra, Reference Hazarika, Upadhyay and Mishra2013; Ozha et al. 2015). The Neoproterozoic monazite chemical ages (~780–800 Ma) in the rims of the monazites in BJ-28 are similar to the ages of the felsic volcanic rocks in the Malani Igneous Suite (~770 Ma) in the Marwar Craton in the west of the ADFB (Gregory et al. Reference Gregory, Meert, Binge, Pandit and Torsvik2009). This age population is lacking in BJ-30. However, we are unable to correlate these monazite growth ages with any particular metamorphic/deformation event. The interpretation of the Neoproterozoic ages, therefore, remains uncertain, and needs further investigation. It is possible that the monazite chemical age in BJ-28 located close to the Hindoli Group, but lacking in BJ-30, may correspond with the Jahazpur thrust or a younger reactivation event along the thrust.
5.c. Inconsistencies in monazite ages in the Aravalli Craton
The present study demonstrates that the ~1.7 Ga high-grade metamorphism in the BGC-II (Wiedenbeck, Goswami & Roy, Reference Wiedenbeck, Goswami and Roy1996 Reference Wiedenbeck, Goswami and Roy b ; Roy et al. Reference Roy, Kröner, Bhattacharya and Rathore2005; Buick et al. Reference Buick, Allen, Pandit, Rubatto and Hermann2006) is not restricted to the west of the Delwara Lineament but extends eastwards within the expansive anatectic gneisses of the Aravalli Supergroup. This implies that the BGC-II and the anatectic gneisses of the Aravalli Supergroup shared a common tectono-metamorphic history since Late Palaeoproterozoic time. In this context, the monazite chemical ages reported in the supracrustal belts of Pur–Banera (Ozha et al. Reference Ozha, Mishra, Hazarika, Jeyagopal and Yadav2016), Rajpura–Dariba and Rampura–Agucha (Hazarika, Upadhyay & Mishra, Reference Hazarika, Upadhyay and Mishra2013) lead to several contradictions. In the Pur–Banera Complex, Ozha et al. (Reference Ozha, Mishra, Hazarika, Jeyagopal and Yadav2016) correlate three metamorphic stages (M1 (peak P–T at ~5.5 kbar, 520–550°C), M2 (peak P–T at ~7.5 kbar and 580–660°C) and M3 (with peak P–T at ~8.0 kbar and 590–640°C)) with monazite chemical ages at ~1.82 Ga, 1.35–1.37 Ga and ~1.0 Ga, respectively. On the other hand, in the Rajpura–Dariba supracrustal rocks, monazite chemical ages are populated at ~1.62 Ga and ~1.87 Ga only; the authors correlate the more populous older age cluster with the ~1.8 Ga Pb–Pb age reported by Deb et al. (Reference Deb, Thorpe, Cumming and Wagner1989) for syn-sedimentary sulfide mineralization in the supracrustal rocks. By contrast in the Rampura–Agucha anatectic gneisses (Hazarika, Upadhyay & Mishra, Reference Hazarika, Upadhyay and Mishra2013), the mean values of the overwhelmingly large number of chemical spot ages in three monazite-dated samples occur at 1006±13 Ma (AM-370/3), 1041±14 Ma (AM-5) and 1004±12 Ma (AM-370/12A); in the samples, minor age populations occur at ~1.36 Ga, ~1.66 Ga (AM-370/3) and ~1.57 Ga (AM-5). Based on the data, the authors suggest that the Rampura–Agucha supracrustal rocks experienced two amphibolite-facies tectono-thermal events, the older event at ~1.6 Ga (largely obliterated) and the younger high-grade metamorphism at ~1.0 Ga.
Assuming that the polymetamorphism invoked by the authors is induced by regional tectono-thermal events, unless conclusive evidence of local heat sources (heat release from younger magma emplacements, and heating induced by brittle/ductile shearing) proves to the contrary, several inconsistencies are evident in the monazite age data. Notable among these are: (i) the ~1.3 Ga amphibolite-facies M2 metamorphic event recorded in Pur–Banera is lacking in the Rajpura–Dariba supracrustal belt, and in the Deoli–Shahpura anatectic gneisses that arguably form the basement for the supracrustal belts, and also in the BGC-II; (ii) the ~1.7–1.6 Ga tectono-metamorphic event lacking in Pur–Banera is recorded in the BGC-II, the Deoli–Shahpura anatectic gneisses (this study), and in the supracrustal rocks of Rajpura–Dariba and Rampura–Agucha; and (iii) the expansive ~1.7 Ga metamorphism in the BGC-II and the Deoli–Shahpura migmatite gneisses occurred at anatectic conditions, but the Rajpura–Dariba lithologies overlying the gneisses reached middle-amphibolite-facies conditions. Several lines of evidence may suggest that the supracrustal belts may have been tectonically accreted to the basement gneisses or were deposited on the basement gneisses post ~1.3 Ga, and deformed-metamorphosed subsequently at ~1.0 Ga, e.g. (i) the lack of ~1.3 Ga metamorphic monazite in the supracrustal belts (barring Pur–Banera) and in the anatectic gneisses of the Aravalli Supergroup, and (ii) the prevalence of ~1.7 Ga metamorphic monazites in the Aravalli Supergroup and the lack of this age in Pur–Banera. In the former case (tectonic accretion), the pre-Grenvillian monazites are likely to record pre-accretionary metamorphism in the allochthonous block; in the second case (deposition on gneiss basement), the >1.0 Ga monazites possibly qualify as detrital grains of igneous/metamorphic origin inherited from the source rocks. A critical structural analysis of the basement–cover relationships in the supracrustal belts, combined with robust age determination to ascertain the time of accretion/deposition of the supracrustal belts is likely to distinguish between the two possible scenarios in the ADFB.
Acknowledgements
LS acknowledges the Faculty Initiation Grant 2013, sponsored by IIT Roorkee for conducting field trips and analyses. This research was also financially supported by the Slovak Research and Development Agency (projects APVV-0080-11 to MJ and APVV-14-0278 to IP), and the Slovak Scientific Grant Agency VEGA (projects 2/0060/16 to MJ and 2/0067/16 to IP). The authors acknowledge two anonymous reviewers for their suggestions which helped to improve the scientific content of the manuscript.
Appendix. Methods and data
Mineral chemical analyses (EPMA)
Major element mineral chemical analyses have been carried out with a CAMECA SX100 at the Indian Institute of Technology Bombay. Operating conditions for feldspar analyses were 15 kV with 15 nA and 15 kV, 20 nA for other minerals. ZAF corrections were applied.
XRF analyses
Bulk compositions of the samples BJ-28 and BJ-30 were determined by XRF. Analysis was done by an X-ray fluorescence spectrometer (XRF) – Bruker S8 Tiger – WD system at the Wadia Institute of Himalayan Geology, India.
Table A1. Bulk compositions (molar proportions) normalized to 100
