1. Introduction
The Central Asian Orogenic Belt (CAOB), located between the Siberian Craton to the north, European Craton to the west and Tarim–North China Craton to the south (Fig. 1a), is considered the largest Phanerozoic accretionary orogen in the world. It is characterized by a complicated tectonic evolution and significant crustal growth during Phanerozoic time (Jahn et al. Reference Jahn, Wu and Chen2000; Windley et al. Reference Windley, Alexeiev, Xiao, Kroner and Badarch2007; Xiao et al. Reference Xiao, Han, Yuan, Sun, Lin, Chen, Li, Li and Sun2008; Windley & Xiao, Reference Windley and Xiao2018). As an important component of the southwestern segment of the CAOB, West Junggar in NW China is considered to be a critical area for Phanerozoic crustal growth owing to the occurrence of several early Palaeozoic ophiolitic mélange belts, voluminous Carboniferous volcanic–plutonic magmatism and a complex tectonic evolution (e.g. Jahn et al. Reference Jahn, Windley, Natal’in and Dobretsov2004; Xiao et al. Reference Xiao, Han, Yuan, Sun, Lin, Chen, Li, Li and Sun2008; Geng et al. Reference Geng, Sun, Yuan, Xiao, Xian, Zhao, Zhang, Wong and Wu2009; Zhang et al. Reference Zhang, Xiao, Han, Ao, Yuan, Sun, Geng, Zhao, Guo and Ma2011a ; Choulet et al. Reference Choulet, Faure, Cluzel, Chen, Lin and Wang2012; Xiao & Santosh, Reference Xiao and Santosh2014), and has long been of widespread concern in the geological community. However, the tectonic nature of the Late Carboniferous magmatism in southern West Junggar still remains controversial. Data for Late Carboniferous magmatic rocks showing diverse geochemical signatures and different ages (Fig. 1b) have led to very different interpretations of the Late Carboniferous tectonic setting of West Junggar.

Fig. 1. (a) Simplified tectonic sketch of the Central Asian Orogenic Belt (after Jahn et al. Reference Jahn, Wu and Chen2000) and (b) regional geological map of southern West Junggar, Xinjiang (modified after BGMRXUAR, 1993; Duan et al. Reference Duan, Li, Zhi, Yang and Gao2019).
Some researchers proposed that the West Junggar oceanic basin closed at ~320 Ma and was followed by the onset of post-collisional magmatism since Late Carboniferous time based on research on the detrital zircons in modern river sands and extensively distributed A-type granitoids (e.g. Han et al. Reference Han, Ji, Song, Chen and Zhang2006; Xu et al. Reference Xu, Han, Ren, Zhou, Zhang, Chen, Su, Li and Liu2012; Gao et al. Reference Gao, Xiao, Pirajno, Wang, He, Yang and Yan2014; Liu et al. Reference Liu, Han, Gong and Chen2019). However, many other scholars drew the conclusion that West Junggar was still in a subduction-dominated island arc setting during Late Carboniferous to Early Permian times according to the occurrence of voluminous calc-alkalic I-type granitoids, slab-derived adakites and sanukitic rocks in the region and systematic palaeogeographic and geological studies (e.g. Zhang et al. Reference Zhang, Wan, Jiao and Zhang2006; Xiao et al. Reference Xiao, Han, Yuan, Sun, Lin, Chen, Li, Li and Sun2008; Shen et al. Reference Shen, Shen, Liu, Meng, Dai and Yang2009, Reference Shen, Shen, Pan, Li, Dong, Wang, Zhu, Dai and Guan2012; Duan et al. Reference Duan, Li, Wang, Ji, Cheng and Guo2015, Reference Duan, Li, Wang, Zhi, Chao and Ma2018a ,b,c, 2019; Li et al. Reference Li, Li, Wang, Yang, Wang, Xiang, Liu and Tong2017). Recently, a 321–290 Ma ridge subduction model based on the existence of some tholeiites, A-type granitoids, charnockites, adakites and high-Mg dioritic dykes in West Junggar has also been proposed (e.g. Geng et al. Reference Geng, Sun, Yuan, Xiao, Xian, Zhao, Zhang, Wong and Wu2009; Tang et al. Reference Tang, Wang, Wyman, Li, Zhao, Jia and Jiang2010, Reference Tang, Wang, Wyman, Li, Zhao and Yang2012; Yin et al. Reference Yin, Yuan, Sun, Long, Zhao, Wong, Geng and Cai2010, Reference Yin, Long, Yuan, Sun, Zhao and Geng2013, Reference Yin, Chen, Xiao, Yuan, Sun, Tang, Yu, Long, Cai, Geng, Zhang and Liu2015; Windley & Xiao, Reference Windley and Xiao2018). Obviously, arguments remain concerning the Late Carboniferous tectonic regime of southern West Junggar despite numerous studies having been performed. Previous studies mainly concentrated on the granitoids (e.g. Chen & Arakawa, Reference Chen and Arakawa2005; Han et al. Reference Han, Ji, Song, Chen and Zhang2006; Geng et al. Reference Geng, Sun, Yuan, Xiao, Xian, Zhao, Zhang, Wong and Wu2009; Shen et al. Reference Shen, Shen, Liu, Meng, Dai and Yang2009; Gao et al. Reference Gao, Xiao, Pirajno, Wang, He, Yang and Yan2014; Duan et al. Reference Duan, Li, Wang, Ji, Cheng and Guo2015, Reference Duan, Li, Wang, Zhi, Chao and Ma2018a ,b), ophiolitic mélanges (Gu et al. Reference Gu, Li, Zhang, Tong and Wang2009; Yang et al. Reference Yang, Li, Santosh, Gu, Yang, Zhang, Wang, Zhong and Tong2012; Zhu et al. Reference Zhu, Chen and Qiu2015 and related references therein) and dioritic dykes (e.g. Tang et al. Reference Tang, Wang, Wyman, Li, Zhao, Jia and Jiang2010; Yin et al. Reference Yin, Yuan, Sun, Long, Zhao, Wong, Geng and Cai2010, Reference Yin, Long, Yuan, Sun, Zhao and Geng2013, Reference Yin, Chen, Xiao, Yuan, Sun, Tang, Yu, Long, Cai, Geng, Zhang and Liu2015; Duan et al. Reference Duan, Li, Zhi, Wan and Ren2018c , 2019) in the study area, with less attention paid to the volcanic rocks, especially to the Late Carboniferous volcanic rocks.
Basaltic magmas may be considered relatively reliable for constraining their tectonic settings. This is because basaltic magmas with distinctive geochemical characteristics are associated with specific tectonic settings. Recently, we identified a suite of Late Carboniferous back-arc basin basalts (BABB) from the Chengjisihanshan Formation in southern West Junggar. This study presents new laser-ablation inductively coupled plasma mass spectrometer (LA-ICP-MS) zircon U–Pb ages, major and trace elements, and Sr–Nd–Pb–Hf isotope data for the basaltic rocks. The data are used to shed light on the Late Carboniferous tectonic setting of southern West Junggar, and also provide robust constraints on the tectonic evolution of the Junggar Ocean in Late Carboniferous time.
2. Geological background
2.a. Regional geology
West Junggar is composed of Palaeozoic intra-oceanic arcs and accretionary complexes and records accretionary processes in the southwestern part of the CAOB (Feng et al. Reference Feng, Coleman, Tilton and Xiao1989; Windley et al. Reference Windley, Alexeiev, Xiao, Kroner and Badarch2007; Xiao et al. Reference Xiao, Han, Yuan, Sun, Lin, Chen, Li, Li and Sun2008; Choulet et al. Reference Choulet, Faure, Cluzel, Chen, Lin and Wang2012; Windley & Xiao, Reference Windley and Xiao2018; Zhang et al. Reference Zhang, Xiao, Luo, Chen, Windley, Song, Han and Safonova2018). It can generally be subdivided into northern and southern parts by the Xiemisitai Fault. Northern West Junggar is mainly composed of two nearly E–W-trending magmatic arcs; from north to south they are the late Palaeozoic Zharma-Saur and early–middle Palaeozoic Boshchekul-Chingiz magmatic arcs (Fig. 1b). Its Palaeozoic lithostratigraphy ranges in age from Ordovician to Permian (BGMRXUAR, 1993).
In contrast to the nearly E–W-trending faults in the northern part, southern West Junggar is characterized by several NE-trending faults, including the Barleik, Hatu and Darbut faults from west to east. Moreover, many ophiolitic mélanges are distributed along the major faults in the southern part (Fig. 1b), with their ages varying from late Neoproterozoic to latest Devonian, demonstrating a complicated accretionary history for the Palaeo-Asian Ocean. Additionally, late Palaeozoic intermediate to felsic granitic intrusions and dioritic dykes are also extensively distributed (Fig. 1b). Granitic intrusions mostly consist of A-type and I-type granitoids and adakites, and are characterized by their short emplacement duration (c. 329–287 Ma) and high positive εNd(t) (+4.60 to +9.20) and εHf(t) (+4.60 to +16.8) values with very young Nd and Hf model ages (e.g. Chen & Arakawa, Reference Chen and Arakawa2005; Han et al. Reference Han, Ji, Song, Chen and Zhang2006; Geng et al. Reference Geng, Sun, Yuan, Xiao, Xian, Zhao, Zhang, Wong and Wu2009; Shen et al. Reference Shen, Shen, Liu, Meng, Dai and Yang2009; Tang et al. Reference Tang, Wang, Wyman, Li, Zhao, Jia and Jiang2010, Reference Tang, Wang, Wyman, Li, Zhao and Yang2012; Gao et al. Reference Gao, Xiao, Pirajno, Wang, He, Yang and Yan2014; Duan et al. Reference Duan, Li, Wang, Ji, Cheng and Guo2015, Reference Duan, Li, Wang, Zhi, Chao and Ma2018a ,b). The dioritic dykes intruded most of geologic bodies, with their ages clustering mainly around the Late Carboniferous to earliest Permian period (c. 321–292 Ma); some were reported with sanukitic high-Mg diorite features (Tang et al. Reference Tang, Wang, Wyman, Li, Zhao, Jia and Jiang2010; Yin et al. Reference Yin, Yuan, Sun, Long, Zhao, Wong, Geng and Cai2010, Reference Yin, Long, Yuan, Sun, Zhao and Geng2013, Reference Yin, Chen, Xiao, Yuan, Sun, Tang, Yu, Long, Cai, Geng, Zhang and Liu2015; Duan et al. Reference Duan, Li, Zhi, Wan and Ren2018c , Reference Duan, Li, Zhi, Yang and Gao2019).
The strata in the southern part are dominated by Devonian and Carboniferous volcaniclastic sediments, and are separated by the Hatu Fault, as shown in Figure 1b. The Devonian strata, distributed principally on the northwestern side of the Hatu Fault, consist mainly of neritic-littoral facies pyroclastic sediments and andesitic volcanic rocks. The Carboniferous volcano-sedimentary strata, including the Lower Carboniferous Heishantou, Baogutu and Xibeikulasi formations and Upper Carboniferous Chengjisihanshan Formation, are most widely distributed on both sides of the Darbut and Barleik faults. The Heishantou Formation, mainly distributed on Barleik Mountain, consists of volcaniclastic rocks, clastic rocks and Nb-enriched basalts and basaltic andesites (Li et al. Reference Li, Shen, Wang, Guo, Tong and Yang2014). The Baogutu Formation is composed of deep-sea facies fine-grained clastic rocks consisting of siliceous and muddy siltstone, tuffaceous siltstone, felsic tuff, variegated chert, limestone and basic to intermediate volcanic rocks with zircon U–Pb ages of c. 346–324 Ma (Guo et al. Reference Guo, Liu, Wang, Song, Xu and Su2010; Geng et al. Reference Geng, Sun, Yuan, Zhao and Xiao2011; Shen et al. Reference Shen, Pan, Xiao, Li, Dai and Zhu2013; Yang et al. Reference Yang, Li, Tong, Li, Wu and Wang2016). Previous studies have confirmed that the volcanic rocks within the Baogutu Formation distributed in the northern Karamay region possess alkaline oceanic island basalt characteristics (c. 346 Ma; Yang et al. Reference Yang, Li, Tong, Li, Wu and Wang2016), and ~324 Ma tholeiites erupted in the Hatu region were reported to have BABB affinity (Shen et al. Reference Shen, Pan, Xiao, Li, Dai and Zhu2013). The Xibeikulasi Formation, conformably overlying the Baogutu Formation on the southern side of the Hongshan pluton (Li et al. Reference Li, Tong, Zhang, Liu, Zhang and Wang2010), is characterized by conglomerate, pebbly sandstone and coarse sandstone, representing coarse debris flow deposits (BGMRXUAR, 1993; Li et al. Reference Li, Tong, Zhang, Liu, Zhang and Wang2010).
The studied basaltic rocks are hosted in the Upper Carboniferous Chengjisihanshan Formation. The Chengjisihanshan Formation, established by Xiang et al (Reference Xiang, Li, Xu, Zhang and Tong2013), is characterized by basic to intermediate volcanic rocks consisting mainly of basalt, basaltic andesite and andesite, which are sandwiched within neritic facies of volcano-sedimentary rocks, including strongly cleaved pebbled sandstone, feldspathic lithic sandstone, thin-layered tuff, tuffaceous siltstone, fine sandstone and chert with minor limestone lenses. An angular unconformable contact between the Chengjisihanshan Formation and the Lower Carboniferous strata was observed at the stratotype section of the Chengjisihanshan Formation in the Baijiantan region, while fault contacts were observed in local areas (Xiang et al. Reference Xiang, Li, Xu, Zhang and Tong2013). Meanwhile, Moscovian fossils, such as Choristites sp. and Pseudotimania sp., were found in limestone lenses (Xiang et al. Reference Xiang, Li, Xu, Zhang and Tong2013), indicating that the Chengjisihanshan Formation was formed in Late Carboniferous time.
2.b. Geology and petrology of the Chengjisihanshan Formation
In the Suoerkuduke–Aletunzhawati area of southern West Junggar, we distinguished an assemblage of NE-trending volcanic rocks and their associated sedimentary rocks in the Chengjisihanshan Formation based on the results of a detailed geological survey at a scale of 1:5000 and sampling (Fig. 2a). The measured sections of A–A′ and B–B′ in the Aletunzhawati area preserve a suite of well-exposed volcanogenic–sedimentary rocks composed mainly of tuffaceous sandstone, feldspathic lithic sandstone, pebbled sandstone, conglomerate, chert, basalt and pyroxene basalt (Fig. 2b), presenting a clear red-coloured hue in the field (Fig. 3a, b, c). These volcanogenic–sedimentary rocks were strongly cleaved, while only a small number of interlaminar folds could be observed in the field. Volcanic rocks occurred as stratified or intercalated beds, and were in conformable contact with the chert and tuffaceous siltstone in section A–A′, or were in fault contact with the tuffaceous lithic sandstones in section B–B′ (Fig. 2b). Additionally, the occurrence of interbedded grey-green basalts and dark-red cherts (Fig. 3d) and basalt pillow structures (Fig. 3e) can be observed in some well-preserved outcrops.

Fig. 2. (a) Geological map of the Aletunzhawati area. Lines A–A′ and B–B′ show the location of the measured sections. (b) Geological sections along A–A′ and B–B′ showing the volcanic rocks from the study area.

Fig. 3. Photos showing field outcrops of different components of the Chengjisihanshan Formation. (a–c) Field outcrops showing red-coloured hue; (d) occurrence of interbedded grey-green basalts and dark-red cherts; (e) pillow basalt. Hammer for scale is 285 mm long. Notebook for scale is 175 mm long.
Furthermore, a set of NE- to NEE- and subparallel W–E-striking faults were also developed in the study area. The NE- and NEE-striking faults cut through the eastern and southern boundaries of the Chengjisihanshan Formation, respectively, and separated this stratigraphic unit from the Lower Carboniferous Baogutu and Xibeikulasi formations (Fig. 2a). The strata of the Chengjisihanshan Formation in the study area dip steeply to the SE (Fig. 2b), and the vast majority of the sedimentary rocks are strongly cleaved owing to the influence of massive faults in the area during the later stage (Fig. 2a). Notably, a gabbro body with an outcrop area of c. 0.1 km2 is observed in section B–B′ (Fig. 2b). The gabbro intruded into brecciated tuff and lithic sandstone, and showed a normal mid-oceanic ridge basalt (N-MORB) affinity with a zircon U–Pb age of 296.1 ± 2.7 Ma; it was believed to have originated from a depleted mantle source in an extensional tectonic setting (Zhang & Zhu, Reference Zhang and Zhu2018).
In this contribution, basaltic samples were collected from the Chengjisihanshan Formation in the Aletunzhawati area. The basalt and pyroxene basalt are grey-green in hand specimen and are dominated by massive structures with porphyric textures. In thin-sections, the basaltic samples mainly contain pyroxene and plagioclase, and generally experienced varying degrees of epidotization and chloritization (Fig. 4). The basalt (Fig. 4a, b) and pyroxene basalt (Fig. 4c, d) have fine- to medium-grained or porphyritic textures, with ~10 % euhedral to subhedral phenocrysts of plagioclase and pyroxene up to 0.5 mm long in a groundmass (~90 %) of fine-grained to aphanitic plagioclase, pyroxene, chlorite, epidote and minor Fe–Ti oxides and opaque minerals.

Fig. 4. Photomicrographs of (a, b) basalt and (c, d) pyroxene basalt in the Chengjisihanshan Formation viewed in polarized and cross-polarized light. Px – pyroxene; Pl – plagioclase; Chl – chlorite; Ep – epidote.
3. Analytical methods
3.a. Zircon U–Pb dating
Zircon grains were separated by routine physical approaches employing elutriation, heavy liquids and magnetic techniques, and then carefully hand-picked under a binocular microscope, mounted in epoxy and polished down to their cores for analysis. In order to understand the internal structure of the zircon directly, cathodoluminescence (CL) images were taken using a Quanta 400FEG environmental scanning electron microscope at the State Key Laboratory of Continental Dynamics, Northwest University, China.
LA-ICP-MS zircon U–Pb dating was carried out at the Key Laboratory for the Study of Focused Magmatism and Giant Ore Deposits, MNR, Xi’an Centre of Geological Survey, CGS, China. Laser sampling was performed using a GeoLas Pro. An Agilent 7700x ICP-MS instrument was used to acquire ion-signal intensities. Helium was applied as a carrier gas. Argon was used as the make-up gas and mixed with the carrier gas via a T-connector before entering the ICP-MS. Each analysis incorporated a background acquisition of ~10 s (gas blank) followed by 40 s of data acquisition from the sample. The Agilent Chemstation was utilized for the acquisition of each individual analysis. Off-line selection and integration of background and analyte signals, and time-drift correction and quantitative calibration for trace-element analyses and U–Pb dating were performed using Glitter 4.4. Concordia diagrams and weighted mean calculations were made using Isoplot/Ex_ver 3 (Ludwig, Reference Ludwig2003).
3.b. Whole-rock geochemical analyses
Whole-rock samples were ultrasonically cleaned repeatedly using diluted hydrochloric acid (3–5 wt %) to remove surface impurities, followed by coarse crushing to 2–4 cm. All of the samples were powdered to less than 200 mesh suitable for analytical testing. Major- and trace-element analyses were conducted at the Ministry of Education Key Laboratory of Western China’s Mineral Resources and Geological Engineering, Xi’an, China. The fused glass discs were made for major-element oxide testing by a condensation after the sufficiently homogeneous mixture of lithium borate flux with a 1:8 sample-to-Li2B4O7–LiBO2 flux ratio fused in an auto fluxer between 1050 °C and 1100 °C. Major-element contents were analysed using a Shimadzu LAB CENTER XRF-1800 sequential scanning X-ray fluorescence spectrometer, with analytical uncertainties of < 2 wt %. The loss on ignition (LOI) was weighed after baking for 90 min at a high temperature of 1000 °C in an oven. Trace and rare earth elements (REEs) were analysed using a Thermo-X7 ICP-MS. ICP-MS trace-element analyses were made after a standard acid-digestion procedure using a mixture of HF–HNO3 and a sinter procedure using Na2O2 (for REEs). Standard reference materials SY-2 and MRG-1 were used to monitor the data quality during the course of this study. The precision and accuracy of the trace-element analyses are estimated to be better than 5 wt % (relative), except for Nb and Ta (better than 10 wt %).
3.c. Sr–Nd–Pb isotopic analyses
The whole-rock Sr–Nd isotopic ratios were determined using an IsoProbe-T thermal ionization mass spectrometer (TIMS) at the Analytical Laboratory, Beijing Research Institute of Uranium Geology, China. Sample powders were dissolved in a HF + HNO3 + HClO4 mixture. Digested samples were first dried and dissolved in 6N HCl, then dried again and redissolved in 0.5N HCl for Sr and Nd separation or 0.5N HBr for Pb separation. Sr and Nd fractions were separated following standard chromatographic techniques using AG 50-X8 and PTFE-HDEHP resins with HCl as an eluent (Sato et al. Reference Sato, Tassinari, Kawashita and Petronillo1995), while the Pb fraction was separated using a strong alkali anion exchange resin with HBr and HCl as eluents (Babinski et al. Reference Babinski, VanSchmus and Chemale1999; Marques et al. Reference Marques, Dupré and Piccirillo1999). The mass fractionation corrections for Sr and Nd isotopic ratios are based on the 86Sr/88Sr ratio of 0.1194 and 146Nd/144Nd ratio of 0.7219, respectively. The 87Sr/86Sr ratio of the Standard NBS987 and 143Nd/144Nd ratio of the Standard SHINESTU determined in this study were 0.710250 ± 0.000007 (2σ) and 0.512118 ± 0.000003 (2σ), respectively. For whole-rock Pb isotopic determinations, ~100 mg of powder were weighed into a Teflon beaker, spiked and dissolved in concentrated HF at 180 °C for 7 h. Lead was separated and purified by a conventional cation-exchange technique (AG 1-X8, 20–400 resin). Total procedural blanks were < 50 pg Pb. Isotopic ratios were measured by a VG-354 mass spectrometer. Repeated analyses of SRM 981 yielded average values of 206Pb/204Pb = 16.9325 ± 3 (2σ), 207Pb/204Pb = 15.4853 ± 3 (2σ) and 208Pb/204Pb = 36.6780 ± 9 (2σ). External precisions are less than 0.005, 0.005 and 0.0015.
3.d. In situ zircon Hf isotope analyses
In situ zircon Hf isotope analyses were performed using a GeoLas Pro laser-ablation system coupled to a Neptune multiple-collector ICP-MS at the Key Laboratory for the Study of Focused Magmatism and Giant Ore Deposits, MNR, Xi’an Centre of Geological Survey, CGS, China. A stationary GeoLas Pro laser-ablation spot with a beam diameter of 32 μm was used for the analyses. All the Hf analyses were done on the same spot used for U–Pb laser ablation. The ablated material was transported from the laser-ablation cell using helium as a carrier gas, and then combined with argon in a mixing chamber before being introduced to the ICP-MS plasma. Correction for 176Lu and 176Yb isobaric interferences on 176Hf used 176Lu/175Lu = 0.02658 and 176Yb/173Yb = 0.796218 (Chu et al. Reference Chu, Taylor, Chavagnae, Nesbitt, Boela, Milton, German, Bayon and Burton2002), respectively. Zircon GJ-1 was used as the reference standard and yielded a weighted mean 176Hf/177Hf ratio of 0.282030 ± 40 (2σ) during this study. A decay constant for 176Lu of 1.865 × 10−11/a (Scherer et al. Reference Scherer, Münker and Mezger2001) and the present-day chondritic ratios of 176Hf/177Hf = 0.282772 and 176Lu/177Hf = 0.0332 (Blichert-Toft & Albarède, Reference Blichert-Toft and Albarède1997) were adopted to calculate εHf values. Single-stage model ages were calculated by reference to depleted mantle with a present-day 176Hf/177Hf ratio of 0.28325 and 176Lu/177Hf ratio of 0.0384 (Vervoot & Blichert-Toft, Reference Vervoot and Blichert-Toft1999).
4. Results
4.a. Zircon U–Pb geochronology
Two basaltic samples from the Chengjisihanshan Formation were chosen for LA-ICP-MS zircon U–Pb determination; their sampling locations are shown in Figure 2a, and the analytical results are listed in Table 1. Zircons separated from the basalt (CJSH-1) and pyroxene basalt (CJSH-2) are transparent, colourless or light yellow, and long prismatic in shape. The grains are 40–120 μm long and 30–90 μm wide with length–width ratios of 1:1 to 2.5:1. They have a well-developed but incomplete crystal morphology, and are half-baked with broad growth zoning or no growth zoning in CL images (Fig. 5), which is a typical feature of zircons from magmatic rocks with relatively low silica content (Corfu et al. Reference Corfu, Hanchar, Hoskin and Kinny2003). Moreover, the chondrite-normalized REE distribution patterns of the zircons are consistent, characterized by light rare earth element (LREE) depletions, heavy rare earth element (HREE) enrichments, positive Ce anomalies and negative Eu anomalies (Fig. 6a), which are obviously different from those of hydrothermal zircons and consistent with typical magmatic-origin zircons (Zhao, Reference Zhao2010). Additionally, zircon grains from the two investigated basaltic samples have variable Th (23.3–465 ppm and 32.1–466 ppm, respectively) and U (48.3–887 ppm and 77.2–776 ppm, respectively) concentrations, and all zircons display relatively high Th/U ratios of 0.37–0.75 and 0.33–0.96, respectively (Table 1). They also exhibit favourable positive correlations between Th and U (Fig. 6b), further suggesting a magmatic origin (Belousova et al. Reference Belousova, Griffin, O’Reilly and Fisher2002).
Table 1. LA-ICP-MS zircon U–Pb isotopic analysis of the basaltic rocks in the Chengjisihanshan Formation in southern West Junggar


Fig. 5. Representative CL images and U–Pb apparent ages of zircons from the basaltic rocks in the Chengjisihanshan Formation.

Fig. 6. (a) Chondrite-normalized rare earth element patterns and (b) Th–U diagram for zircons from the basaltic rocks in the Chengjisihanshan Formation (chondrite-normalized values are from Sun & McDonough, Reference Sun, McDonough, Saunders and Norry1989; shaded areas after Zhao, Reference Zhao2010).
Twenty-nine zircon grains were analysed for basalt sample CJSH-1, and all the data are concordant or nearly concordant, yielding 206Pb–238U apparent ages of 304 Ma to 319 Ma (Table 1), with a weighted mean age of 310.3 ± 3.9 Ma (MSWD = 0.16, n = 29) (Fig. 7a). Twenty zircon grains were analysed for pyroxene basalt sample CJSH-2, and the data are also concordant, yielding 206Pb–238U ages of 301 Ma to 326 Ma (Table 1), with a weighted mean age of 313.0 ± 5.7 Ma (MSWD = 0.27, n = 20) (Fig. 7b). All tested zircon grains lack visible inherited cores, and, thus, we consider the c. 313–310 Ma age to be the crystallization age of the basaltic rocks in the Chengjisihanshan Formation, which is consistent with the Moscovian fossil ages reported by Xiang et al. (Reference Xiang, Li, Xu, Zhang and Tong2013).

Fig. 7. LA-ICP-MS zircon U–Pb concordia diagrams for the basaltic rocks in the Chengjisihanshan Formation.
4.b. Major-element contents
Major-element data for eight samples in this study are listed in Table 2, and their sampling locations are shown in Figure 2b. The investigated basalts and pyroxene basalts from the Chengjisihanshan Formation display minor variations in SiO2 and Al2O3 ranging from 47.76 to 52.06 wt % and from 13.26 to 14.39 wt %, respectively. The contents of MgO are 6.55–7.68 wt %, with Mg no. (100 × molar Mg/(Mg + ΣFe)) values varying from 52.9 to 58.9. These rocks have relatively high TiO2 (1.09–1.36 wt %) and TFe2O3 (11.22–13.58 wt %) contents but low K2O (0.05–0.74 wt %) and P2O5 (0.06–0.09 wt %) contents (Table 2), and plot in the sub-alkaline basalt field on the Nb/Y–Zr/TiO2 diagram (Fig. 8a; Winchester & Floyd, Reference Winchester and Floyd1977) and define a typical tholeiite compositional trend on the SiO2–FeOT/MgO diagram (Fig. 8b; Miyashiro, Reference Miyashiro1974).
Table 2. Geochemical compositions of the basaltic rocks from the Chengjisihanshan Formation

Note: LOI – loss on ignition; Mg no. = (100 × molar Mg/(Mg + ΣFe)); Eu/Eu* = EuN/(SmN × GdN)1/2; normalization values from Sun & McDonough (Reference Sun, McDonough, Saunders and Norry1989).

Fig. 8. (a) 0.0001 × Zr/TiO2–Nb/Y (Winchester & Floyd, Reference Winchester and Floyd1977) and (b) FeOT/MgO–SiO2 (Miyashiro, Reference Miyashiro1974) diagrams for the basaltic rocks. Data from Mariana BABB (Pearce et al. Reference Pearce, Stern, Bloomer and Fryer2005) and Hatu BABB (Shen et al. Reference Shen, Pan, Xiao, Li, Dai and Zhu2013) are shown for comparison.
4.c. Trace-element compositions
The studied tholeiitic rocks are low in REE concentrations (∑REE = 37.8–49.8 ppm) and display nearly flat REE patterns ((La/Yb)N = 0.87–1.07) with no to slightly positive Eu anomalies (Eu/Eu* = 0.99–1.16, only one sample 1.48) on the chondrite-normalized REE diagram (Fig. 9a), suggesting an N-MORB affinity. These rocks also have ratios of Lu/Yb (~0.15), Zr/Y (~2.53) and Y/Tb (~34) similar to those of N-MORB (Sun & McDonough, Reference Sun, McDonough, Saunders and Norry1989), but are relatively enriched in LREEs and large ion lithophile elements (LILEs) such as U, and depleted in Nb, Ta, Ti, Zr and Hf compared to the neighbouring incompatible elements on the N-MORB-normalized trace-element spidergrams (Fig. 9b), sharing an island arc basalt affinity (e.g. Perfit et al. Reference Perfit, Gust, Bence, Arculus and Taylor1980).

Fig. 9. (a) Chondrite-normalized rare earth element and (b) N-MORB-normalized trace-element patterns for the basaltic rocks. Chondrite, normal mid-ocean ridge basalt (N-MORB), enriched mid-ocean ridge basalt (E-MORB) and ocean island basalt (OIB) compositions are from Sun & McDonough (Reference Sun, McDonough, Saunders and Norry1989). Data for the Mariana FAB are from Reagan et al. (Reference Reagan, Ishizuka, Stern, Kelley, Ohara, Blichert-toft, Bloomer, Cash, Fryer and Hanan2010) and for the Okinawa, Mariana and Hatu BABBs are from Shinjo et al. (Reference Shinjo, Chung, Kato and Kimura1999), Pearce et al. (Reference Pearce, Stern, Bloomer and Fryer2005) and Shen et al. (Reference Shen, Pan, Xiao, Li, Dai and Zhu2013), respectively.
4.d. Sr–Nd–Pb–Hf isotope compositions
Two basaltic samples from the Chengjisihanshan Formation were chosen for Sr–Nd–Pb isotope analyses. Measured and initial isotopic ratios (back-calculated to 310 Ma) are reported in Table 3. All of the samples show a limited range in their 143Nd/144Nd and 87Sr/86Sr ratios. Initial 87Sr/86Sr ratios of the basaltic rocks are 0.70571 and 0.70573. Their initial Nd isotopes display small variations of 0.51245 and 0.51246 and calculated εNd(t) values of +4.21 and +4.36 (Table 3). The measured 206Pb/204Pb, 207Pb/204Pb and 208Pb/204Pb ratios are 18.415 and 18.537, 15.536 and 15.552, 38.367 and 38.770, respectively, and their corresponding initial Pb isotopic ratios are less variable with (206Pb/204Pbi) = 17.775–17.812, (207Pb/204Pbi) = 15.504–15.512 and (208Pb/204Pbi) = 37.687–37.781 (Table 3).
Table 3. Whole-rock Sr–Nd–Pb isotopic compositions of the basaltic rocks in the Chengjisihanshan Formation

Note: 87Rb/86Sr and 147Sm/144Nd ratios are calculated using Rb, Sr, Sm and Nd concentrations; decay constants used are 1.42 × 10−11/a for 87Rb (Steiger & Jäger, Reference Steiger and Jäger1977) and 6.54 × 10−12/a for 147Sm (Lugmair & Marti, Reference Lugmair and Marti1978); εNd(t) values are calculated using present-day (147Sm/144Nd)CHUR = 0.1967 and (143Nd/144Nd)CHUR = 0.512638 (Wasserburg et al. Reference Wasserburg, Jacobsen, DePaolo, McCulloch and Wen1981); λ238U = 1.55125 × 10−10/y, λ235U = 9.8485 × 10−10/y, λ232Th = 4.9475 × 10−11/y (Steiger & Jäger, Reference Steiger and Jäger1977).
Two samples of zircons dated by U–Pb methods were also analysed for their in situ Lu–Hf isotope compositions, and the results are presented in Table 4. Seven zircon spot analyses were obtained for the basalt sample CJSH-1 (c. 310 Ma), yielding variable εHf(t) values in the range between +13.2 and +15.7 and initial 176Hf/177Hf ratios between 0.282959 and 0.283027 with juvenile two-stage model ages (T DM2) mainly from 321 to 481 Ma. Similarly, six spot analyses were also made for the pyroxene basalt sample CJSH-2 (c. 313 Ma). The determined positive εHf(t) values for this sample vary between +8.06 and +14.9, and their initial 176Hf/177Hf ratios vary between 0.282811 and 0.283013, corresponding to T DM2 model ages in the range of 373 to 810 Ma.
Table 4. Zircon Hf isotopic compositions of the basaltic rocks in the Chengjisihanshan Formation

5. Discussion
5.a. Crustal contamination and fractional crystallization
The basaltic samples exhibit negative Nb–Ta anomalies on the N-MORB-normalized trace-element spidergrams (Fig. 9b). The Nb–Ta troughs are typical characteristics of arc-related magma, but rocks formed by the contamination of crustal materials can also develop this anomaly (e.g. Pearce & Peate, Reference Pearce and Peate1995). However, their high Mg no. values (52.9–58.9), low La/Nb (1.51–1.67 < 12), Th/Ce (~0.06 < 0.15) and Th/La (0.06–0.07 < 0.3) ratios, together with the absence of inherited zircons (Fig. 5), indicate that crustal contamination did not play a significant role in the generation of the magmas (Lassiter & DePaolo, Reference Lassiter, DePaolo, Mahoney and Coffin1997; Plank, Reference Plank2005). Moreover, the presence of basaltic samples with higher contents of Na2O than K2O (Table 2) also supports this view, which is incompatible with remarkable involvement of crustal materials. More importantly, the samples have high positive εHf(t) and εNd(t) values, which are typical features of the depleted mantle, and further preclude the possibility of significant crustal contamination. We therefore consider that the trace-element characteristics most likely reflect the mantle source signature and magmatic process during the magmas’ ascent.
The basaltic rocks show relatively low CaO/Al2O3 ratios of 0.22–0.69, which, together with the positive correlation between the CaO/Al2O3 ratios and MgO contents suggest the possibility of clinopyroxene fractionation (Fig. 10a; Naumann & Geist, Reference Naumann and Geist1999). However, the consistent Mg no. (52.9–58.9) values and Cr (175–209 ppm) and Ni (88.3–104 ppm) concentrations and the lack of correlation between Cr, Ni and MgO are indicative of insignificant olivine fractionation (Fig. 10b, c). In addition, the basaltic rocks show no to slightly positive Eu anomalies (Eu/Eu* = 0.99–1.48) on the chondrite-normalized REE diagram (Fig. 9a), demonstrating negligible plagioclase fractionation. Moreover, the negative correlation between TiO2 and MgO indicates that Fe–Ti oxide fractionation did not play any major role in the magma evolution (Fig. 10d).

Fig. 10. Plots of selected elements and their ratios for the basaltic rocks.
5.b. Origins of the basaltic rocks
Petrographically, the basaltic rocks from the Chengjisihanshan Formation underwent varying degrees of low-temperature hydrothermal alteration, as verified by the occurrence of secondary minerals such as epidote and chlorite (Fig. 4) and relatively high LOI values of 2.33 to 5.10 wt % (Table 2). Therefore, the immobile high field strength elements (e.g. Ti, Zr, Y, Nb and REEs) will be used in the following discussion to characterize the origin and possible tectonic environment of the basaltic rocks.
The tholeiitic rocks from the Chengjisihanshan Formation display low SiO2 (47.76–52.06 wt %) but high MgO (6.55–7.68 wt %) contents and Mg no. (52.9–58.9) values with relatively high Sc, Co and Ni (43.1–52.3 ppm, 46.2–54.1 ppm and 88.3–104 ppm, respectively) concentrations, and also show subparallel REE patterns comparable to those of N-MORB (Fig. 9), indicative of a similar mantle source (Rapp & Watson, Reference Rapp and Watson1995). However, the studied basaltic rocks are characterized by high field strength element depletions relative to LILEs with negative Nb, Ta, Ti, Zr and Hf anomalies in the N-MORB-normalized multi-element plots (Fig. 9b) in comparison with the N-MORB-derived magma, which is a typical feature of volcanic arc basalts (e.g. Perfit et al. Reference Perfit, Gust, Bence, Arculus and Taylor1980), reflecting the addition of slab-derived components in a subduction zone (Pearce & Peate, Reference Pearce and Peate1995; Pearce et al. Reference Pearce, Stern, Bloomer and Fryer2005; Hémond et al. Reference Hémond, Hofmann, Vlastélic and Nauret2006). Moreover, they have low total REE concentrations (37.8–49.8 ppm) with their Nb/La (0.74–0.87) and Nb/Ta (12.0–13.0) ratios being lower than those of N-MORB (0.93 and 17.7, respectively; Sun & McDonough, Reference Sun, McDonough, Saunders and Norry1989), further suggesting that additional slab-derived components were added into their mantle source, and these samples have experienced a subduction-related enrichment process (Pearce & Peate, Reference Pearce and Peate1995).
Subduction-related components generally comprise slab-derived fluids or melts, and the input of such components would produce different geochemical signatures in the magma. Partial melting of mantle metasomatized by slab-derived fluids would produce magmas with high Ba/Th ratios, while partial melting of mantle metasomatized by slab-derived melts may yield high Th/Ta ratios (Pearce et al. Reference Pearce, Stern, Bloomer and Fryer2005). The high Ba/Th (164–1612, with the exception of only one sample) and low Th/Ta ratios (1.24–1.48) of the studied rocks imply the influence of slab-derived fluids. In addition, their large compositional variations of Rb/Y (0.05–0.63) and Sr/Nd (41.5–153) but low and constant Nb/Y (0.12–0.14) and Th/Yb (0.10–0.13) ratios also strongly suggest that the subduction components were most likely dominated by slab-derived fluids (Hawkesworth et al. Reference Hawkesworth, Turner, McDermott, Peate and Van Calsteren1997; Woodhead et al. Reference Woodhead, Hergt, Davidson and Eggins2001). It was also confirmed that the Nb/U and Ce/Pb ratios of magma derived from the mantle will significantly decrease with the addition of slab fluids (Klein & Karsten, Reference Klein and Karsten1995). The results show that the Nb/U (19.4–37.5; 29.1 on average) and Ce/Pb (11.0–31.6; 22.9 on average) ratios of the basaltic rocks are remarkably lower than those of depleted mantle (47 ± 10 and 25 ± 5, respectively; Hofmann et al. Reference Hofmann, Jochum, Seufert and White1986), further indicating the involvement of slab fluids in the magma-generation process (Klein & Karsten, Reference Klein and Karsten1995). More insights into the amount of involvement of slab fluids come from the εNd(t) versus (Nd/Hf)PM diagram (Fig. 11; Tang et al. Reference Tang, Qin, Su, Sakyi, Liu, Mao, Santosh and Ma2013). The results show that the primary magma underwent < 5 % slab-derived fluid metasomatism in the mantle source.

Fig. 11. Plots of εNd(t) versus (Nd/Hf)PM (after Tang et al. Reference Tang, Qin, Su, Sakyi, Liu, Mao, Santosh and Ma2013) for the basaltic rocks. DMM – depleted mantle-derived melt; AOCF – altered oceanic crust fluid; GLOSS – global subducting sediment; SF – slab fluid; SSF – subducted sediment fluid.
Based on Nd–Sr–Pb and Hf isotope systematics, the mantle source of the studied tholeiitic rocks can be further limited. The basaltic rocks have high positive εNd(t) (+4.21 to +4.36) and εHf(t) (+13.0 on average) values, indicating their N-MORB-like depleted mantle features (Fig. 12a, b). It should be noted that the relatively high initial 87Sr/86Sr ratios make the plots of the basaltic samples deviate away from the mantle array in the Nd–Sr isotope diagram (Fig. 12a), probably suggesting the incorporation of Sr from seawater in the source (Lin et al. Reference Lin, Yuan, Zhang, Sun, Long, Wang and Huang2019). In the 207Pb/204Pb and 208Pb/204Pb versus 206Pb/204Pb diagrams (Fig. 12c, d), all the basaltic samples plotted in the Indian MORB field (Kempton et al. Reference Kempton, Pearce, Barry, Fitton, Langmuir and Christie2002), consistent with the Nd–Hf isotope characteristics. Notably, the Sr–Nd isotopes of the basaltic rocks are similar to those of Early Carboniferous subduction-related volcanic rocks in the Miaoergou and Karamary areas (c. 331–344 Ma; Geng et al. Reference Geng, Sun, Yuan, Zhao and Xiao2011) and BABB in Hatu area (c. 324 Ma; Shen et al. Reference Shen, Pan, Xiao, Li, Dai and Zhu2013) of southern West Junggar (Fig. 12a), probably suggesting their genetic affinities.
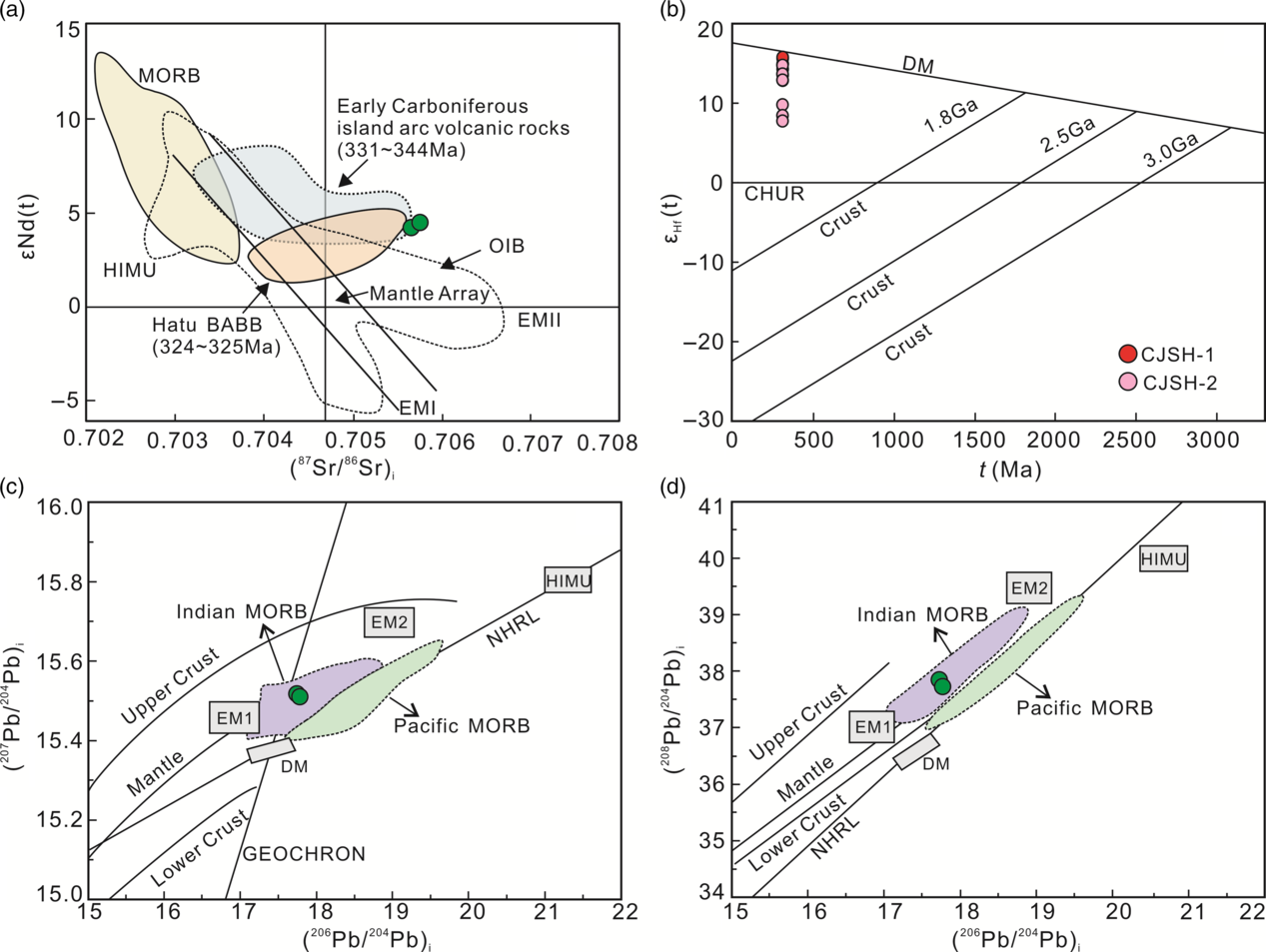
Fig. 12. (a) Sr–Nd, (b) zircon Hf, and (c, d) Pb isotopic compositions for the basaltic rocks. Data for the Hatu BABB are from Shen et al. (Reference Shen, Pan, Xiao, Li, Dai and Zhu2013), for the Early Carboniferous island arc volcanic rocks in southern West Junggar are from Geng et al. (Reference Geng, Sun, Yuan, Zhao and Xiao2011), and for the Indian MORB and Pacific MORB are from Kempton et al. (Reference Kempton, Pearce, Barry, Fitton, Langmuir and Christie2002). CHUR – chondritic uniform reservoir; DM – depleted mantle; EMI/1 – enriched mantle I; EMII/2 – enriched mantle II; HIMU – high μ; MORB – mid-ocean ridge basalt; NHRL – Northern Hemisphere Reference Line; OIB – ocean island basalt.
A more useful approach to the mantle source nature is the use of plots of incompatible elements and their ratios. As shown in the Sm/Yb versus Sm and Sm/Yb versus La/Sm diagrams (Fig. 13; Aldanmaz et al. Reference Aldanmaz, Pearce, Thirlwall and Mitchell2000), all the samples plotted on the spinel lherzolite partial melting curve, which shows that the samples were formed by ~5–10 % partial melting of a spinel lherzolite facies mantle source. Additionally, the low (Gd/Yb)N, (Dy/Yb)N and La/Yb ratios (1.05–1.50, 1.06–1.17 and 1.21–1.49, respectively) and relatively high HREE concentrations (14.5–18.0 ppm; > 15 times chondrite) of the basaltic rocks further imply that these rocks originated from partial melting of spinel lherzolite mantle (Chung, Reference Chung1999). Therefore, we infer that the generation of the parental magmas of the basaltic rocks in the Chengjisihanshan Formation were most likely derived from ~5–10 % partial melting of a depleted spinel lherzolitic mantle source metasomatized by < 5 % slab-derived fluids.

Fig. 13. (a) Plots of Sm/Yb–Sm and (b) Sm/Yb–La/Sm (after Aldanmaz et al. Reference Aldanmaz, Pearce, Thirlwall and Mitchell2000) for the basaltic rocks. DMM – depleted MORB mantle; E-MORB – enriched mid-ocean ridge basalt; N-MORB – normal mid-ocean ridge basalt; PM – primitive mantle.
5.c. Tectonic setting and geological significance
It is generally believed that the mafic magmas possessing both MORB-like and arc-like compositional characteristics formed in a fore-arc basin or back-arc basin above the subduction zone (e.g. Gribble et al. Reference Gribble, Stern, Bloomer, Stüben, O’Hearn and Newman1996; Shinjo et al. Reference Shinjo, Chung, Kato and Kimura1999; Taylor & Martinez, Reference Taylor and Martinez2003; Reagan et al. Reference Reagan, Ishizuka, Stern, Kelley, Ohara, Blichert-toft, Bloomer, Cash, Fryer and Hanan2010); therefore, data for the Okinawa BABB from a typical modern intra-continental back-arc basin (Shinjo et al. Reference Shinjo, Chung, Kato and Kimura1999), the Mariana BABB from a typical modern intra-oceanic back-arc basin (Pearce et al. Reference Pearce, Stern, Bloomer and Fryer2005) and the Early Carboniferous Hatu BABB in southern West Junggar (c. 324 Ma; Shen et al. Reference Shen, Pan, Xiao, Li, Dai and Zhu2013) as well as the Mariana fore-arc basin basalt (FAB) (Reagan et al. Reference Reagan, Ishizuka, Stern, Kelley, Ohara, Blichert-toft, Bloomer, Cash, Fryer and Hanan2010) were added to Figures 8 and 9 for comparison. The results show that the tholeiitic rocks from the Chengjisihanshan Formation are analogous to those of the Mariana BABB (Pearce et al. Reference Pearce, Stern, Bloomer and Fryer2005) and Hatu BABB (Shen et al. Reference Shen, Pan, Xiao, Li, Dai and Zhu2013) both in major- and trace-element compositional aspects, but differ from those of the Okinawa BABB (Shinjo et al. Reference Shinjo, Chung, Kato and Kimura1999) and Mariana FAB (Reagan et al. Reference Reagan, Ishizuka, Stern, Kelley, Ohara, Blichert-toft, Bloomer, Cash, Fryer and Hanan2010) (Figs 8, 9). Additionally, the asthenospheric MORB-type mantle occurring in a fore-arc basin would likely be characterized by high oxygen fugacity, transforming vanadium into a high valence form with strong incompatibility, and partial melting of this mantle would yield relatively low Ti/V ratios (Shervais, Reference Shervais1982; Reagan et al. Reference Reagan, Ishizuka, Stern, Kelley, Ohara, Blichert-toft, Bloomer, Cash, Fryer and Hanan2010). However, the basaltic rocks in this study yielded Ti/V ratios (25.9–27.9) much higher than those of typical FAB (15.3–16.4; Reagan et al. Reference Reagan, Ishizuka, Stern, Kelley, Ohara, Blichert-toft, Bloomer, Cash, Fryer and Hanan2010) but comparable to BABB from the Mariana Trough (16.8–34.9; Pearce et al. Reference Pearce, Stern, Bloomer and Fryer2005). Moreover, our samples also have similar (La/Yb)N (0.87–1.07), Sm/Nd (0.32–0.35), Th/Yb (0.10–0.13) and Th/Nb (0.10–0.12) ratios to those from the Mariana Trough (Pearce et al. Reference Pearce, Stern, Bloomer and Fryer2005).
More importantly, trace and body fossils together with turbidites clearly reveal a deep-sea to littoral shallow marine environment in West Junggar during Late Carboniferous time (e.g. Li & Jin, Reference Li and Jin1989; Jin & Li, Reference Jin and Li1998). In addition, regional palaeogeographic and geological study similarly indicates that the northern Xinjiang region still remained an active continental margin until Late Carboniferous to Early Permian times (Xiao et al. Reference Xiao, Han, Yuan, Sun, Lin, Chen, Li, Li and Sun2008). Furthermore, the Upper Carboniferous Chengjisihanshan Formation in the Aletunzhawati area consists of tuffaceous siltstones, tuffaceous sandstones, feldspar lithic sandstones, lenticular limestone containing marine fossils, and a minor amount of pebbly sandstones and conglomerates with intercalated beds of chert and basalt in the field (Fig. 2b), indicating the existence of an oceanic basin in Late Carboniferous time. The aforementioned geochemical data and the above lines of geological evidence jointly suggest that the studied basaltic rocks were more likely generated in a back-arc basin setting, which is also consistent with the plots of 2*Nb–Zr/4–Y (Fig. 14a; Meschede, Reference Meschede1986), V–Ti/1000 (Fig. 14b; Shervais, Reference Shervais1982) and La/Nb–Y (Fig. 14c; Floyd et al. Reference Floyd, Kelling, Gökçen and Gökçen1991). On the basis of this study and taking previous interpretations into consideration, we here prefer to suggest that the studied Late Carboniferous tholeiitic rocks from the Chengjisihanshan Formation in the Aletunzhawati area were formed in an intra-oceanic back-arc basin tectonic setting (Fig. 15). The identification of Late Carboniferous BABB in the Chengjisihanshan Formation indicates that the arc-basin evolutionary system during late Early Carboniferous time (~324 Ma; Shen et al. Reference Shen, Pan, Xiao, Li, Dai and Zhu2013) continued until 310 Ma in southern West Junggar, and the Junggar Ocean likely closed after Late Carboniferous time.

Fig. 14. Tectonic setting discrimination diagrams with trace elemental plots for the basaltic rocks in the Chengjisihanshan Formation. (a) 2*Nb–Zr/4–Y (after Meschede, Reference Meschede1986); (b) V–Ti/1000 (after Shervais, Reference Shervais1982); (c) La/Nb–Y (after Floyd et al. Reference Floyd, Kelling, Gökçen and Gökçen1991). Data for the Mariana BABB are from Pearce et al. (Reference Pearce, Stern, Bloomer and Fryer2005) and for the Hatu BABB are from Shen et al. (Reference Shen, Pan, Xiao, Li, Dai and Zhu2013). IAT – island arc tholeiites; OFB – ocean floor basalt; VAB – volcanic arc basalt; WPA – within-plate alkali basalt; WPT – within-plate tholeiitic basalt.
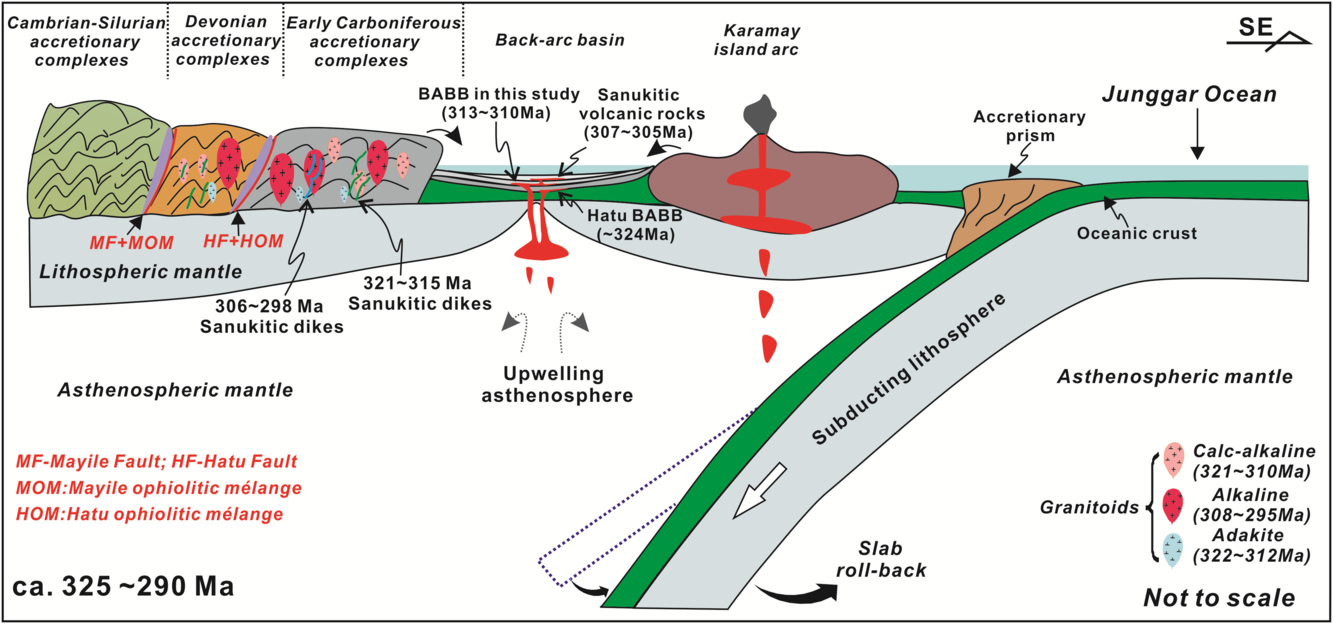
Fig. 15. Schematic map showing the tectonic setting of the Late Carboniferous to Early Permian period (c. 325–290 Ma) in southern West Junggar (modified after Duan et al. Reference Duan, Li, Zhi, Yang and Gao2019). Age data shown in this figure are from Chen & Arakawa (Reference Chen and Arakawa2005), Han et al. (Reference Han, Ji, Song, Chen and Zhang2006), Geng et al. (Reference Geng, Sun, Yuan, Xiao, Xian, Zhao, Zhang, Wong and Wu2009), Gao et al. (Reference Gao, Xiao, Pirajno, Wang, He, Yang and Yan2014), Shen et al. (Reference Shen, Shen, Liu, Meng, Dai and Yang2009, Reference Shen, Shen, Pan, Li, Dong, Wang, Zhu, Dai and Guan2012, Reference Shen, Pan, Xiao, Li, Dai and Zhu2013), Tang et al. (Reference Tang, Wang, Wyman, Li, Zhao, Jia and Jiang2010, Reference Tang, Wang, Wyman, Li, Zhao and Yang2012), Yin et al. (Reference Yin, Yuan, Sun, Long, Zhao, Wong, Geng and Cai2010, Reference Yin, Long, Yuan, Sun, Zhao and Geng2013, Reference Yin, Chen, Xiao, Yuan, Sun, Tang, Yu, Long, Cai, Geng, Zhang and Liu2015), Duan et al. (Reference Duan, Li, Wang, Ji, Cheng and Guo2015, Reference Duan, Li, Wang, Zhi, Chao and Ma2018a ,b,c, 2019), Li et al. (Reference Li, Li, Wang, Yang, Wang, Xiang, Liu and Tong2017).
The Carboniferous is a crucial period for the tectonic transition of West Junggar. Massive Carboniferous–Permian magmatic rocks are extensively distributed in the southern part, which provide favourable material conditions for understanding the characteristics of Late Palaeozoic magmatic activities and the tectonic evolution of the Junggar Ocean. In the recent tectonic models, West Junggar has been linked to multiple intra-oceanic accretionary episodes from Devonian to Late Carboniferous time (e.g. Xiao et al. Reference Xiao, Han, Yuan, Sun, Lin, Chen, Li, Li and Sun2008; Zhang et al. Reference Zhang, Xiao, Han, Mao, Ao, Guo and Ma2011b ; Li et al. Reference Li, Li, Wang, Yang, Wang, Xiang, Liu and Tong2017; Duan et al. Reference Duan, Li, Zhi, Wan and Ren2018c , Reference Duan, Li, Zhi, Yang and Gao2019). During Early Carboniferous time, a large number of volcanic rocks generated in the subduction process have been reported in southern West Junggar, such as Nb-enriched arc basalts found in the Barleik Mountain area (~349 Ma; Li et al. Reference Li, Shen, Wang, Guo, Tong and Yang2014), island arc volcanic rocks distributed throughout the Miaoergou–Baijiantan region (~344 Ma; Geng et al. Reference Geng, Sun, Yuan, Zhao and Xiao2011) and oceanic island basalts formed by upwelling of asthenospheric mantle through a slab window in a fore-arc setting in the northern Karamay region during consumption of the West Junggar Ocean (~345 Ma; Yang et al. Reference Yang, Li, Tong, Li, Wu and Wang2016). These special rock associations indicate that there should be a subduction-dominated setting in southern West Junggar during Early Carboniferous time. The discovery of c. 324 Ma BABB in the Hatu area (Shen et al. Reference Shen, Pan, Xiao, Li, Dai and Zhu2013) suggests that a back-arc extensional setting occurred in southern West Junggar at the end of Early Carboniferous time.
In the early to middle stage of Late Carboniferous time, as consumption of the Junggar oceanic lithosphere proceeded, subduction-related intermediate-acid intrusive rocks were widely distributed in southern West Junggar, such as c. 322–312 Ma slab-derived adakitic rocks exposed in the Baogutu, Dulunhe and Huangliangzi regions (Zhang et al. Reference Zhang, Wan, Jiao and Zhang2006; Shen et al. Reference Shen, Shen, Liu, Meng, Dai and Yang2009, Reference Shen, Shen, Pan, Li, Dong, Wang, Zhu, Dai and Guan2012; Tang et al. Reference Tang, Wang, Wyman, Li, Zhao, Jia and Jiang2010; Duan et al. Reference Duan, Li, Wang, Ji, Cheng and Guo2015, Reference Duan, Li, Yang, Zhi, Li, Tao, Gao and Chen2018b ), and contemporaneous sanukitic dykes from the Bieluagaxi and Karamay regions (c. 321–315 Ma; Yin et al. Reference Yin, Yuan, Sun, Long, Zhao, Wong, Geng and Cai2010; Duan et al. Reference Duan, Li, Zhi, Yang and Gao2019), as well as calc-alkaline I-type granitic stocks distributed on both sides of the Darbut Fault (c. 319–310 Ma; e.g. Tang et al. Reference Tang, Wang, Wyman, Li, Zhao and Yang2012; Duan et al. Reference Duan, Li, Wang, Zhi, Chao and Ma2018a ). The confirmation of c. 313–310 Ma intra-oceanic BABB in this study indicates that a remnant oceanic basin under an extensional environment still occurred in southern West Junggar during the middle stage of Late Carboniferous time. Thereafter, volcanic rocks sharing some affinities with sanukitoids were erupted in Hala’alate Mountain (c. 307–305 Ma; Li et al. Reference Li, Li, Wang, Yang, Wang, Xiang, Liu and Tong2017), and contemporaneously, massive dioritic dykes carrying sanukitoid compositions were widely distributed in the Miaoergou region (c. 306–298 Ma; Yin et al. Reference Yin, Long, Yuan, Sun, Zhao and Geng2013, Reference Yin, Chen, Xiao, Yuan, Sun, Tang, Yu, Long, Cai, Geng, Zhang and Liu2015; Duan et al. Reference Duan, Li, Zhi, Wan and Ren2018c ); voluminous alkaline A-type granitic batholiths (c. 308–295 Ma; e.g. Chen & Arakawa, Reference Chen and Arakawa2005; Han et al. Reference Han, Ji, Song, Chen and Zhang2006; Geng et al. Reference Geng, Sun, Yuan, Xiao, Xian, Zhao, Zhang, Wong and Wu2009; Gao et al. Reference Gao, Xiao, Pirajno, Wang, He, Yang and Yan2014) were also generated in the Devonian–Early Carboniferous accretionary complexes (Fig. 15).
6. Conclusions
-
(1) The basaltic rocks from the Chengjisihanshan Formation in southern West Junggar were formed in Late Carboniferous time (c. 313–310 Ma).
-
(2) The basaltic rocks are tholeiitic and exhibit both N-MORB-like and arc-like geochemical signatures, and were likely derived from ~5–10 % partial melting of a depleted spinel lherzolitic mantle source metasomatized by < 5 % slab-derived fluids within an intra-oceanic back-arc basin setting.
-
(3) An arc-basin evolutionary system still existed in southern West Junggar at c. 310 Ma, and the Junggar Ocean closed after Late Carboniferous time.
Acknowledgements
Constructive and thorough comments and suggestions from two anonymous reviewers and Prof. Kathryn Goodenough are gratefully acknowledged, which enhanced the final form of the paper. This study was supported by the National Key R & D Program of China (grant No. 2018YFC0604001), the Xinjiang Geological Exploration Fund (grant No. A16-1-LQ14), and the Fundamental Research Funds for the Central Universities, CHD (grant No. 300102270709) to Qian Zhi.