1. Introduction
High-pressure–low-temperature (HP-LT) metamorphic rocks witness ancient subduction or collision zones (Evans & Brown, Reference Evans and Brown1986). Pressure–temperature–time (P-T-t) paths of such rocks provide major constraints on the evolution of orogens and the dynamics of exhumation processes active in these zones (Thompson & England, Reference Thompson and England1984; Ernst, Reference Ernst1988, Reference Ernst2001). However, linking time to P-T conditions requires that geochronology be tightly coupled with thermobarometry through petrology and mineral chemistry (Müller, Reference Müller2003; Villa & Williams, Reference Villa, Williams, Harlov and Austrheim2012; Warren, Hanke & Kelley, Reference Warren, Hanke and Kelley2012).
The Attic–Cycladic region in the Aegean (Greece) is one of the most studied HP-LT belts of the Mediterranean convergence domain. The tectono-metamorphic record in this part of the Hellenides indicates Eocene burial and exhumation in HP-LT conditions followed by Oligo-Miocene exhumation in low-pressure–high-temperature (LP-HT) conditions below crustal-scale brittle–ductile detachment systems (Jolivet & Brun, Reference Jolivet and Brun2010; Ring et al. Reference Ring, Glodny, Will and Thomson2010; Grasemann et al. Reference Grasemann, Schneider, Stöckli and Iglseder2012; Philippon, Brun & Gueydan, Reference Philippon, Brun and Gueydan2012). Within that time framework, two points are still poorly constrained. On the one hand, the meaning of Cretaceous ages determined in HP-LT rocks is debated (Bröcker & Enders, Reference Bröcker and Enders1999, Reference Bröcker and Enders2001; Tomaschek et al. Reference Tomaschek, Kennedy, Villa, Lagos and Ballhaus2003; Bröcker & Franz, Reference Bröcker and Franz2006; Bulle et al. Reference Bulle, Bröcker, Gärtner and Keasling2010; Huyskens & Bröcker, Reference Huyskens and Bröcker2014). This point is important to correlate the tectonic events in the internal Hellenides with those of the Rhodope on the opposite side of the Vardar suture zone (Burg, Reference Burg2012). On the other hand, precise time constraints on the activity of the different detachments systems and their individual segments are still missing. Deciphering P-T-t records in order to distinguish exhumation below detachment systems from earlier phases is a key issue in the understanding of the mechanical behaviour of detachment zones.
In this respect, Andros is a key island of the Attic–Cycladic region as part of the North Cycladic Detachment System, of which the timing and geometry are complex (Brichau et al. Reference Brichau, Ring, Carter, Monie, Bolhar, Stockli and Brunel2007, Reference Brichau, Ring, Carter, Bolhar, Monie, Stockli and Brunel2008; Jolivet et al. Reference Jolivet, Lecomte, Huet, Denele, Lacombe, Labrousse, Pourhiet and Mehl2010). Besides, the significance of Early Cretaceous ages determined there remains unclear (Bröcker & Franz, Reference Bröcker and Franz2006; Huyskens & Bröcker, Reference Huyskens and Bröcker2014). Here we present results of 40Ar–39Ar geochronology coupled with thermobarometry from Andros island. Using also data from the literature, we constrain the local P-T-t record and discuss the implications on the evolution of the Hellenic subduction zone and the behaviour of detachments during exhumation of the HP-LT rocks in the Aegean.
2. Geological settings
2.a. Geology of the Cyclades
The Cycladic archipelago belongs to the Hellenides–Taurides belt that resulted from convergence between Africa and Eurasia in the Eastern Mediterranean during Mesozoic–Cenozoic time (Jacobshagen et al. Reference Jacobshagen, Dürr, Kockel, Kopp, Kowalczyk, Berckhemer, Büttner, Cloos, Roeder and Schmidt1978). It is now located in the Aegean Sea (Fig. 1a), the back-arc of the active Hellenic subduction zone (Le Pichon & Angelier, Reference Le Pichon and Angelier1981). Three main palaeogeographical units are distinguished in the Cyclades (Fig. 1a; Bonneau, Reference Bonneau, Dixon and Robertson1984): a basal unit correlated with the Gavrovo–Tripolitza nappe of the external zones (Avigad et al. Reference Avigad, Garfunkel, Jolivet and Azañón1997; Jolivet et al. Reference Jolivet, Rimmelé, Oberhänsli, Goffé and Candan2004; Ring et al. Reference Ring, Glodny, Will and Thomson2007a , Reference Ring, Will, Glodny, Kumerics, Gessner, Thomson, Gungor, Moie, Okrusch and Drueppel b ); the middle Attic–Cycladic units (the Attic–Cycladic Blueschist unit and the Cycladic Basement); and the uppermost Pelagonian unit (Upper Cycladic Nappe).

Figure 1. (a) Simplified geological map of the central Aegean, modified after Grasemann et al. (Reference Grasemann, Schneider, Stöckli and Iglseder2012) and Jolivet et al. (Reference Jolivet, Faccenna, Huet, Labrousse, Le Pourhiet, Lacombe, Lecomte, Burov, Denèle, Brun, Philippon, Paul, Salaün, Karabulut, Piromallo, Monié, Gueydan, Okay, Oberhänsli, Pourteau, Augier, Gadenne and Driussi2012). The Attic–Cycladic–Menderes unit regroups the Attic–Cylcadic Blueschist unit, the Cycladic basement, the Menderes basement and the Menderes cover. It appears as windows below the upper units: the Pelagonian unit (Olympos, Pelion, Ossa and Cyclades in Greece) and the Bornova flysch and Lycian nappes (Menderes massif in Turkey). It lies over the external units of the Hellenides. The Attic–Cycladic–Menderes unit has been exhumed below sets of low-angle normal faults: the North Cycladic Detachment system (NCDS), the West Cycladic Detachment System (WCDS) and the Naxos-Paros Detachment system (NPDS). The arrows indicate the sense of shear associated with these detachments. (b) Geological map of Andros island, after Mehl et al. (Reference Mehl, Jolivet, Lacombe, Labrousse, Rimmele, Taymaz, Yilmaz and Dilek2007). The contact between the upper unit and the Cycladic Blueschist is a low-angle normal fault belonging to the NCDS (Jolivet et al. Reference Jolivet, Lecomte, Huet, Denele, Lacombe, Labrousse, Pourhiet and Mehl2010). The contact between the Makrotantalon unit and the Cycladic Blueschist is not well defined everywhere (Huyskens & Bröcker, Reference Huyskens and Bröcker2014) and follows serpentinite bodies (Papanikolaou, Reference Papanikolaou1978). The stars indicate preserved HP-LT index minerals (Mehl et al. Reference Mehl, Jolivet, Lacombe, Labrousse, Rimmele, Taymaz, Yilmaz and Dilek2007; Huyskens & Bröcker, Reference Huyskens and Bröcker2014). The investigated samples are also localized on the map.
The Attic–Cycladic units comprise a Permo-Mesozoic continental margin and remnants of an oceanic domain, the Attic–Cycladic Blueschist unit that is correlated with the Pindos Nappe, detached from its pre-alpine basement, the Cycladic Basement (Huet, Labrousse & Jolivet, Reference Huet, Labrousse and Jolivet2009). They were affected by two metamorphic events (Altherr et al. Reference Altherr, Schliestedt, Okrusch, Seidel, Kreuzer, Harre, Lenz, Wendt and Wagner1979, Reference Altherr, Kreuzer, Wendt, Lenz, Wagner, Keller, Harre and Höhndorf1982; Wijbrans & McDougall, Reference Wijbrans and McDougall1986, Reference Ernst1988; Bröcker et al. Reference Bröcker, Kreuzer, Matthews and Okrusch1993; Putlitz, Cosca & Schumacher, Reference Putlitz, Cosca and Schumacher2005; Lagos et al. Reference Lagos, Scherer, Tomaschek, Münker, Keiter, Berndt and Ballhaus2007; Grasemann et al. Reference Grasemann, Schneider, Stöckli and Iglseder2012): a blueschist and eclogite facies episode during Eocene time (55–35 Ma) followed by retrogression in the greenschist and amphibolite facies during Oligo-Miocene time (30–15 Ma).
The Pelagonian unit consists of ophiolitic material obducted over a pre-alpine continental basement and its Palaeo-Mesozoic cover. In the Cyclades, it is characterized by LP-HT metamorphism yielding Late Cretaceous ages (Patzak, Okrusch & Kreuzer, Reference Patzak, Okrusch and Kreuzer1994). Miocene greenschist-facies metamorphism locally affected the Pelagonian unit (Bröcker & Franz, Reference Bröcker and Franz1998; Zeffren et al. Reference Zeffren, Avigad, Heimann and Gvirtzman2005). Although Early and Late Cretaceous blueschists occur in the Pelagonian unit of Evia Island (Maluski et al. Reference Maluski, Vergely, Bavay, Bavay and Katsikatsos1981), Olympos (Schermer, Lux & Burchfiel, Reference Schermer, Lux and Burchfiel1990) and Ossa regions (Lips, White & Wijbrans, Reference Lips, White and Wijbrans1998), no high-pressure rock has yet been recognized in the Pelagonian unit from the Cyclades realm.
The contact separating the Attic–Cycladic units and the Pelagonian unit played a major role in the history of the Cyclades. As the boundary between the upper and lower plates of the Hellenic subduction zone, it acted during Eocene time as a thrust and then as a normal fault, leading successively to burial and syn-orogenic exhumation of the Attic–Cycladic units (Trotet, Jolivet & Vidal, Reference Trotet, Jolivet and Vidal2001). After the Hellenic slab began to retreat 30–35 Ma ago (Jolivet & Faccenna, Reference Jolivet and Faccenna2000), the Aegean lithosphere started to stretch, the HP-LT rocks then re-equilibrated in LP-HT conditions and were exhumed below several sets of detachments that partly reactivated the Pelagonian unit basal contact. Correlations between detachments lead to the identification of three major detachment systems (Fig. 1a): the North Cycladic Detachment System (NCDS, Jolivet et al. Reference Jolivet, Lecomte, Huet, Denele, Lacombe, Labrousse, Pourhiet and Mehl2010); the Western Cycladic Detachment System (WCDS, Grasemann et al. Reference Grasemann, Schneider, Stöckli and Iglseder2012); and the Naxos–Paros Detachment System (NPDS, Gautier, Brun & Jolivet, Reference Gautier, Brun and Jolivet1993). When exposed, the Pelagonian unit systematically appears as the upper unit of all these detachments.
2.b. Geology of Andros
Three units are distinguished on Andros Island: the Attic–Cycladic Blueschist unit; the Makrotantalon unit; and an upper unit (Papanikolaou, Reference Papanikolaou1978; Mehl et al. Reference Mehl, Jolivet, Lacombe, Labrousse, Rimmele, Taymaz, Yilmaz and Dilek2007). The Attic–Cycladic Blueschist unit is a metasedimentary sequence (schist, marble and interlayered metavolcanite) that contains bodies of ultramafic and metamorphosed basic and felsic magmatic rocks. U–Pb ages on detrital and magmatic zircons constrain the deposition age of the sedimentary sequence to Triassic–Jurassic time (Bröcker & Pidgeon, Reference Bröcker and Pidgeon2007; unpublished data cited in Huyskens & Bröcker, Reference Huyskens and Bröcker2014). HP-LT rocks preserved from retrogression are mostly found along the SW coast (Papanikolaou, Reference Papanikolaou1978; Mehl et al. Reference Mehl, Jolivet, Lacombe, Labrousse, Rimmele, Taymaz, Yilmaz and Dilek2007). They formed at 450–500°C and a minimum pressure of 10 kbar (Reinecke, Reference Reinecke1986) and were first exhumed in the glaucophane stability field in constrictive NE–SW stretching (Ziv et al. Reference Ziv, Katzir, Avigad and Garfunkel2010). The Rb–Sr phengite ages in the range 40–50 Ma are considered to date this episode (Bröcker & Franz, Reference Bröcker and Franz2006; Huyskens & Bröcker, Reference Huyskens and Bröcker2014). Widespread retrogression in the greenschist facies is associated with brittle–ductile NE–SW stretching and top-to-the-NE shear (Mehl et al. Reference Mehl, Jolivet, Lacombe, Labrousse, Rimmele, Taymaz, Yilmaz and Dilek2007). Greenschist facies overprint occurred at conditions in the range 350–520°C and 5–9 kbar (Bröcker & Franz, Reference Bröcker and Franz2006) and is dated at 21–24 Ma by Rb–Sr phengite ages (Bröcker & Franz, Reference Bröcker and Franz2006; Huyskens & Bröcker, Reference Huyskens and Bröcker2014).
The upper unit, correlated with the Pelagonian unit, comprises intensely foliated greenschists and serpentinites lying over a breccia. It is separated from the Attic–Cycladic Blueschist unit by a detachment (Mehl et al. Reference Mehl, Jolivet, Lacombe, Labrousse, Rimmele, Taymaz, Yilmaz and Dilek2007) that is part of the NCDS (Jolivet et al. Reference Jolivet, Lecomte, Huet, Denele, Lacombe, Labrousse, Pourhiet and Mehl2010). Rocks close to the contact yielded Rb–Sr phengite ages between 24 and 28 Ma (Huyskens & Bröcker, Reference Huyskens and Bröcker2014). This is considered to date the activity of the NCDS on Andros.
The Makrotantalon unit is a metasedimentary sequence with characteristics distinct from the Attic–Cycladic Blueschist unit. It contains dolomitic marbles that yielded Permian fossils (Papanikolaou, Reference Papanikolaou1978). U–Pb ages on detrital zircons are older than in the Attic–Cycladic Blueschist unit (c. 260 Ma, unpublished data cited in Huyskens & Bröcker, Reference Huyskens and Bröcker2014). Phengite Rb–Sr ages clustering around 80 Ma indicate a Late Cretaceous metamorphic event (Bröcker & Franz, Reference Bröcker and Franz2006; Huyskens & Bröcker, Reference Huyskens and Bröcker2014). Older ages (c. 105 Ma) have also been found. Index minerals for the HP-LT metamorphism described in mafic rocks (Mehl et al. Reference Mehl, Jolivet, Lacombe, Labrousse, Rimmele, Taymaz, Yilmaz and Dilek2007; Huyskens & Bröcker, Reference Huyskens and Bröcker2014) show that HP-LT rocks are more widespread in the Makrotantalon unit than in the Attic–Cycladic Blueschist unit (Fig. 1b). However, the Makrotantalon and Attic–Cycladic Blueschist units have apparently shared the same LP-HT history. Greenschist facies deformation is continuous across the contact between the two units (Mehl et al. Reference Mehl, Jolivet, Lacombe, Labrousse, Rimmele, Taymaz, Yilmaz and Dilek2007). Similar Rb–Sr phengite ages and P-T conditions have been found in greenschist facies rocks of both (Bröcker & Franz, Reference Bröcker and Franz2006; Huyskens & Bröcker, Reference Huyskens and Bröcker2014). Finally, the contact between the Makrotantalon unit and the Attic–Cycladic Blueschist unit is loosely defined by a series of serpentinite bodies (Papanikolaou, Reference Papanikolaou1978) and its nature has been debated (see Bröcker & Franz, Reference Bröcker and Franz2006; Mehl et al. Reference Mehl, Jolivet, Lacombe, Labrousse, Rimmele, Taymaz, Yilmaz and Dilek2007; Huyskens & Bröcker, Reference Huyskens and Bröcker2014). According to the most recent interpretation, the contact was a thrust which was active at 40–45 Ma as constrained by Rb–Sr phengite ages (Huyskens & Bröcker, Reference Huyskens and Bröcker2014).
3. Coupling thermobarometry and geochronology
Four representative samples selected from a collection of over 40 samples have been investigated (Fig. 1b, Table 1). Andros1 is a garnet-glaucophane schist from Makrotantalon unit. Andros2, Andros3 and Andros4 are micaschists sampled at different structural levels of the lower unit. Pressure–temperature conditions were estimated for all samples and phengite 40Ar–39Ar geochronology was performed on samples Andros1 and Andros2.
Table 1. Samples location

We used an electron microprobe to analyse the minerals belonging to the metamorphic assemblages in thin-sections (service Camparis, Université Pierre et Marie Curie-Paris 6, France; see Yamato et al. Reference Yamato, Agard, Goffé, De Andrade, Vidal and Jolivet2007). Representative electron microprobe analyses are presented in Table 2. Thermodynamic modelling of metamorphic assemblages and mineral compositions was carried out using Theriak-Domino software (de Capitani & Petrakakis, Reference De Capitani and Petrakakis2010) using the updated database of Holland & Powell (Reference Holland and Powell1998) available on the Theriak-Domino website (http://titan.minpet.unibas.ch/minpet/theriak/prog120103/). We used a chemical composition calculated with modal counting and microprobe analyses in order to avoid secondary and retrograde phases (Meyre et al. Reference Meyre, de Capitani, Zack and Frey1999). In the micaschists, the equilibrium conditions of phengite-chlorite pairs were calculated with TWEEQU (thermobarometry with estimation of equilibration state) multi-equilibrium thermobarometry (Berman, Reference Berman1991; Vidal & Parra, Reference Vidal and Parra2000), according to the method described in Yamato et al. (Reference Yamato, Agard, Goffé, De Andrade, Vidal and Jolivet2007). This method was successful for determining the P-T path of the neighbouring island of Tinos (Fig. 1a; Parra, Vidal & Jolivet, Reference Parra, Vidal and Jolivet2002).
Table 2. Representative electron microprobe analyses of garnet, glaucophane, phengite and chlorite from Makrotantalon and lower units

n.a. not analysed; Fe3+ is not recalculated for phyllosilicates
40Ar–39Ar geochronology was performed at Géosciences Montpellier (Université Montpellier 2, France). Two different dating techniques were used. Five single grains of phengite in the fraction 0.5–1 mm were dated by laser-probe step heating (see procedure in Monié & Agard, Reference Monié and Agard2009). Leftover single grains were mounted with double-stick conductive carbon tape and analysed with the electron microprobe (8 grains per sample, core and rim analyses). Additionally, one phengite concentrate from Andros2 was dated by 40Ar–39Ar furnace step heating (grain size 0.25–0.5 mm; see procedure in Arnaud et al. Reference Arnaud, Tapponnier, Roger, Brunel, Scharer, Wen and Zhiqin2003). The 40Ar–39Ar phengite isotopic data are presented in Tables 3 and 4.
Table 3. 40Ar/39Ar phengite isotopic data for the dated single grains from Makrotantalon and lower units
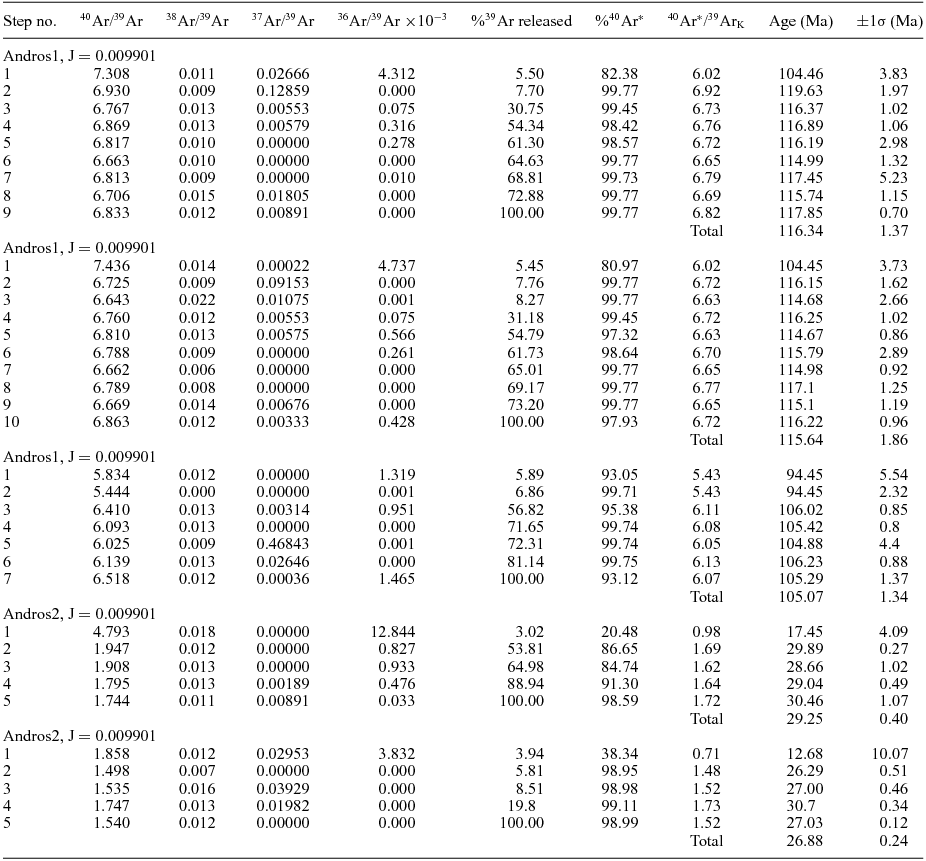
Table 4. 40Ar/39Ar phengite isotopic data for the concentrate from the lower unit

Phengite has been considered for both thermobarometry and geochronology; because this mineral recrystallizes easily, it is stable over a wide range of P-T conditions and its composition strongly depends on the P-T conditions (Parra, Vidal & Agard, Reference Parra, Vidal and Agard2002; Vidal et al. Reference Vidal, De Andrade, Lewin, Munoz, Parra and Pascarelli2006). Chemical analysis of isolated grains allowed the correlation of pressure–temperature–strain evolution provided by in situ petrological and microstructural analysis of thin-sections, as well as ages provided by extracted phengite.
4. P-T-t characterization of Makrotantalon unit
4.a. Description of the sample
The glaucophane-garnet schist Andros1 was collected within serpentinite at the base of the Makrotantalon unit (Fig. 1b). The main assemblage contains garnet, phengite, blue amphibole, quartz and rutile (Fig. 2a). The schistosity defined by phengite, blue amphibole and quartz is folded. Garnet has a mosaic texture and contains inclusion of quartz, rutile and phengite. Chloritized biotite is found as a retrogression product after garnet and phengite. Garnet has a homogeneous composition and is very poor in manganese (Fig. 3a, XAlm = 0.62–0.67, XGrs = 0.16–0.24, XPrp = 0.10–0.16, XSps = 0.01–0.03). The blue amphibole is glaucophane of homogeneous and intermediate composition (XMg ~ 0.5, Fig. 3b, c). Two composition clusters are found in both isolated and in situ phengite (Fig. 3d): Si-high phengite (XMg ~ 0.6, Si ~ 3.4) and Si-low phengite (XMg ~ 0.65, Si ~ 3.2). In thin-section, Si-high phengite is found both as inclusions and in the matrix and Si-low phengite is found in the matrix. Two of the analysed isolated grains are zoned with a Si-rich core and a Si-poor rim (Fig. 3d). The Si-high phengite is therefore older than the Si-poor phengite.

Figure 2. Photomicrographs of the samples. (a) Andros1: the HP-LT equilibrium assemblage contains garnet, glaucophane, phengite and rutile. (b) Andros2: the schistosity is defined by alternating albite-calcite-quartz and phengite-chlorite-quartz layers. (c) Andros 3: the quartz-phengite-epidote-albite-chlorite matrix is cross-cut by a top-to-the-NE shear band containing very-fine-grained phengite, quartz and chlorite. (d) Andros 4: quartz-albite-phengite-chlorite microlithons are separated by a top-to-the-NE extensional crenulation cleavage composed of fine-grained phengite, chlorite and quartz. The thin-sections have been cut in the XZ plane of finite deformation. NE is on the left side of the photos. The scale bars are 500 μm. Mineral abbreviations after Whitney & Evans (Reference Whitney and Evans2010), except Chl-Bt: chloritized biotite.

Figure 3. Chemical composition of minerals belonging to the HP-LT paragenesis in (a–d) glaucophane-garnet schist Andros1 and (d, e) phyllosilicates in micaschists Andros2, Andros3 and Andros4. Circles indicate compositions determined with electron microprobe and stars indicate mineral composition modelled at 18.5 kbar and 550°C. (a) Garnet composition in an Almandine-Grossular-Pyrope ternary diagram. (b, c) Blue amphibole composition in amphibole classification diagrams (Leake et al. Reference Leake, Woolley, Birch, Burke, Ferraris, Grice, Hawthorne, Kisch, Krivovichev, Schumacher, Stephenson and Whittaker2007). (d) Si-XMg diagram of isolated phengite (left panel) and in situ phengite (right panel). (e) Si-XMg diagram of isolated phengite (Andros2, left panel) and in situ phengite (Andros2, Andros3, Andros4, right panel). (f) Chlorite composition in a Si-XMg diagram. End-member abbreviations after Whitney & Evans (Reference Whitney and Evans2010).
The compositions of garnet, glaucophane and phengite (Table 1) are similar to those measured in blueschists from the Cyclades (Schliestedt, Reference Schliestedt1986; Bröcker, Reference Bröcker1990; Trotet, Vidal & Jolivet, Reference Trotet, Vidal and Jolivet2001; Parra, Vidal & Jolivet, Reference Parra, Vidal and Jolivet2002). The Si content in phengite is controlled by the Tschermak substitution and increases with increasing pressure at constant bulk rock chemistry (Massonne & Schreyer, Reference Massonne and Schreyer1987). We therefore suggest that the Si-high phengite together with garnet, glaucophane and rutile represent the equilibrium HP-LT assemblage in sample Andros1. This assemblage is characteristic of the transition between blueschist and eclogite facies conditions in Ca-poor rocks (Evans, Reference Evans1990). Si-low phengite and the chloritized biotite are the products of the partial retrogression of this primary assemblage at lower pressure.
4.b. P-T estimates
An equilibrium assemblage diagram was calculated (chemical composition in wt%: 0.34 TiO2, 45.63 Al2O3, 29.87 FeO, 6.33 MgO, 5.47 CaO, 2.37 Na2O and 9.99 K2O with excess SiO2 and H2O). The observed HP-LT equilibrium assemblage is stable within a large field (520–600°C, 18–22 kbar, Fig. 4a). Comparison between the analysed and modelled chemical compositions of garnet, glaucophane and phengite precisely constrain the P-T conditions of the observed assemblage at 550°C and 18.5 kbar (stars in Figs 3a–d, 4a). At these conditions the modelled compositions match the analyses within natural scatter, except for the XMg in glaucophane (Fig. 3c).

Figure 4. (a) Equilibrium assemblage diagram calculated for glaucophane-garnet schist Andros1 as a function of pressure and temperature. The dotted and dashed lines are isopleths of Si and XMg in the phengite, respectively. Garnet, phengite, rutile, quartz and water being stable at all the conditions covered by the diagram, they are not indicated in the assemblage labelling the different fields. The star indicates the pressure–temperature conditions of peak metamorphism (18.5 kbar and 550°C). Mineral abbreviations after Whitney & Evans (Reference Whitney and Evans2010). (b) Pressure–temperature estimates and uncertainties calculated for phengite-chlorite pairs in micaschists Andros2, Andros3 and Andros4.
These conditions fall within the field corresponding to the observed HP-LT assemblage and are considered the peak conditions associated with the HP-LT event recorded in Makrotantalon unit. The observation of chloritized biotite is consistent with an exhumation path first crossing fields where biotite alone is stable and then fields where chlorite replaces it progressively (Fig. 4a).
4.c. 40Ar–39Ar ages
The three dated isolated phengite grains extracted from Andros1 produced age spectra showing no evidence of a major disturbance in the K–Ar system (Fig. 5a–c). The first step or first two steps (less than 7% of the released 39Ar) have a large uncertainty which is associated with a large atmospheric argon component loosely bound to low retentivity sites, such as grain surface. Two grains yielded 2σ-plateau ages for 95% of the released 39Ar that are identical within the uncertainty: 117.2±1.8 Ma and 115.6±1.9 Ma (Fig. 5a, b). A third grain produced a younger 2σ-plateau age (105.9±1.7 Ma, Fig. 5c). This age overlaps with two phengite Rb–Sr ages at 104.6±3.8 Ma and 103.9±1.3 Ma from an albite schist of the Makrotantalon unit (Fig. 6; Bröcker & Franz, Reference Bröcker and Franz2006; Huyskens & Bröcker, Reference Huyskens and Bröcker2014).

Figure 5. The phengite Ar age spectra (a–c) from glaucophane-garnet schist Andros1 indicate Early Cretaceous ages and (d–f) from micaschist Andros2 indicate Oligo-Miocene ages. The plateau ages, the considered steps for their calculations and the total fusion ages (TFA) are indicated. Steps and calculated ages are provided with a 1σ uncertainty.

Figure 6. Space and time distribution of the radiochronologic ages from Andros on (a) a map and (b) a time scale.
4.d. Interpretation of the 40Ar–39Ar ages
These phengite 40Ar–39Ar ages are either reproducible (c. 116 Ma) or confirmed by geochronology with the Rb–Sr system (c. 105 Ma) and the spectra do not show evidence of major disturbance. The concordance of ages determined with the 40Ar–39Ar and another system probably indicates that excess argon is not a major problem in the dated sample, as it has been demonstrated in other HP belts such as the d’Entrecasteaux belt of Papua New Guinea (Baldwin et al. Reference Baldwin, Lister, Hill, Foster and McDougall1993, Reference Baldwin, Monteleone, Webb, Fitzgerald, Grove and Hill2004; Baldwin, Webb & Monteleone, Reference Baldwin, Webb and Monteleone2008). In general, excess argon is limited to polyorogenic belts where old protoliths are poorly deformed, partially recrystallized and with limited fluid circulation (Schneider et al. Reference Schneider, Bosch, Monié and Bruguier2007). Solid diffusion modelling (Warren, Hanke & Kelley, Reference Warren, Hanke and Kelley2012) using recent diffusion parameters (Harrison et al. Reference Harrison, Célérier, Aikman, Hermann and Heizler2009) suggests that the K–Ar system in white mica has a higher closure temperature (500–550°C) than generally accepted, especially at high pressure, as suggested by previous studies (Lister & Baldwin, Reference Lister and Baldwin1996; Villa, Reference Villa1998). Finally, phengite 40Ar–39Ar geochronology using in situ methods suggests that argon diffusion can be negligible even at relatively high temperatures (Di Vincenzo et al. Reference Di Vincenzo, Ghiribelli, Giorgetti and Palmeri2001; Agard et al. Reference Agard, Monié, Jolivet and Goffé2002; Maurel et al. Reference Maurel, Monié, Respaut, Leyreloup and Maluski2003; Mulch & Cosca, Reference Mulch and Cosca2004; Augier et al. Reference Augier, Agard, Monié, Jolivet, Robin and Booth-Rea2005). The ages determined in sample Andros1 are therefore considered as geologically relevant crystallization ages because of the similarity between the recorded peak temperature in sample Andros1 and the closure temperature for Ar in white mica.
In sample Andros1, two different phengite chemistries have been recognized. Si-high phengite crystallized at peak conditions and Si-low phengite crystallized later during retrogression. Two different ages (c. 116 and 105 Ma) have been determined in this sample, interpreted as crystallization ages. We cannot provide proof of direct correspondence between the phengite chemistry and the ages, and therefore assume that each phengite growth step is associated with one age. We therefore suggest that the 116 Ma age dates the peak conditions or the onset of exhumation right after maximum burial, whereas the 105 Ma age corresponds to a step along the retrogression path. This indicates a short residence time at temperatures higher than 500°C, consistent with negligible Ar loss in the investigated large grains (Warren, Hanke & Kelley, Reference Warren, Hanke and Kelley2012).
5. P-T-t characterization of the Attic–Cycladic Blueschist unit
5.a. Description of samples
The micaschists Andros2, Andros3 and Andros4 have been sampled at different structural levels and in different structures of the Attic–Cycladic Blueschist unit (Fig. 1b). Andros2 has been sampled in a boudin preserved from late deformation. Its microstructure is dominated by a single schistosity (Fig. 2b). In contrast, Andros3 and Andros4 have been sampled in zones of intense top-to-the-NE ductile deformation at a higher structural level closer to the detachment (Fig. 1b). They exhibit shear-bands deflecting the schistosity (Fig. 2c, d). All contain phengite, chlorite, albite and quartz. Additional minerals are calcite and rutile in Andros2 and epidote in Andros3.
In all samples, phengite and chlorite show wide ranges of Si-content (Phg:Si = 3.15–3.52, Chl:Si = 2.6–2.9) and clustered XMg values (Fig. 3e, f; Table 1). Similar variations have been observed in the phyllosilicates of the neighbouring island of Tinos (Parra, Vidal & Jolivet, Reference Parra, Vidal and Jolivet2002). Sample Andros2 shows a slightly larger dispersion in analysis performed on isolated grains compared to in situ measurements (Fig. 3e).
For a constant bulk-rock chemistry, Si variations in phengite indicate variable crystallization pressures (Massonne & Schreyer, Reference Massonne and Schreyer1987). Similarly, the Si variations in chlorite are correlated with variation of crystallization temperature (Vidal, Parra & Trotet, Reference Vidal, Parra and Trotet2001). The observed chemical variations within each sample reflect crystallization at different conditions. The range of Si in the phengite is narrower in Andros2 than in the other micaschists, most probably reflecting a narrower range of crystallization pressure conditions recorded in this sample.
5.b. P-T estimates
The distribution of the P-T estimates determined by multi-equilibrium thermobarometry in the micaschists is complex (Fig. 4b). Different P-T conditions are determined in the same sample and the P-T record is heterogeneous. Sample Andros4 yielded two types of P-T estimates. One P-T estimate is situated at 320°C and 12 kbar, whereas the seven others have a similar pressure (c. 7 kbar) but different temperatures covering a wide range (260–430°C). The latter plot a plateau at constant pressure. Pressure–temperature estimates determined in sample Andros3 confirm either the HP-LT record or the plateau at 7 kbar. These two samples from the same structural level share a common P-T history. Further, two other P-T estimates from sample Andros3 are situated at the maximum temperature of the plateau and lower pressure. Sample Andros2 yielded one cluster of P-T conditions located at the high-temperature part of the plateau. This record is consistent with the deeper structural position of the sample, its monophase structure and the rather homogeneous composition of phyllosilicates.
Even though there is clear evidence for deformation within the Attic–Cycladic Blueschist unit, there is no major internal shear zone (Mehl et al. Reference Mehl, Jolivet, Lacombe, Labrousse, Rimmele, Taymaz, Yilmaz and Dilek2007). This unit is apparently coherent and it is legitimate to define a single composite P-T path rather than multiple paths (insets of Fig. 4b). Three P-T domains are distinguished. Domain 1 corresponds to estimates determined in samples Andros3 and Andros4 with pressure above 10 kbar and temperature in the range 300–400°C. The uncertainty is important, which might reflect partial re-equilibration of the initial mineral chemistry in the following stages of the P-T path. Domain 1 constrains the P-T path in HP-LT conditions. Domain 2 corresponds to estimates determined in the three micaschists with temperature in the range 260–430°C for c. 7 kbar. This domain indicates heating at constant pressure. Domain 3 corresponds to estimates determined in the three micaschists with pressure below 7 kbar for c. 400°C. Domain 3 constrains the P-T path in the greenschist facies. Notice that the P-T estimates yielded by sample Andros2 are in both domains 2 and 3. The P-T conditions in domains 2 and 3 are consistent with most of those determined in mafic greenschists of the Attic–Cycladic Blueschist unit (Fig. 7a; Bröcker & Franz, Reference Bröcker and Franz2006).

Figure 7. Pressure–temperature–time evolution of the (a) Attic–Cycladic Blueschist unit and (b) Makrotantalon unit on Andros. P-T conditions estimated in greenschists are taken from Bröcker & Franz (Reference Bröcker and Franz2006). The range of peak conditions in Tinos (Bröcker, Reference Bröcker1990; Parra, Vidal & Jolivet, Reference Parra, Vidal and Jolivet2002) is taken as a proxy for the peak conditions in the Attic–Cycladic Blueschist unit. (c–e) Three possible P-T-t histories for the Makrotantalon unit.
5.c. 40Ar–39Ar ages
One isolated grain of sample Andros2 yielded a 2σ-plateau age at 29.8±0.6 Ma determined for 97% of the released 39Ar (Fig. 5d). The first step has a large uncertainty, which is associated with a large atmospheric argon component trapped in lesser retentive sites such as the grain surface. No other major disturbance in the K–Ar system can be inferred from this spectrum. Another single grain produced a discordant spectrum for which no plateau can be defined (Fig. 5e), since c. 80% of 39Ar was released in a single step. A concentrate produced a perturbed spectrum (Fig. 5f). The global U-shaped pattern reveals Ar excess degassed at low and high experimental temperatures. The convex-downwards central part is consistent with mixing of two phengite populations (Wijbrans & McDougall, Reference Wijbrans and McDougall1986). The scatter in phengite composition supports this interpretation (Fig. 3e). A younger bound for the age of the older population would be 28 Ma and an older bound for the age of the younger population would be 23 Ma (Fig. 5f). The older bound is consistent with the single-grain plateau age of 29.8±0.6 Ma and an Rb–Sr phengite age of 29.1±2.7 Ma (Fig. 6; Huyskens and Bröcker, Reference Huyskens and Bröcker2014), and the younger bound is consistent with seven Rb–Sr phengite ages in the range 21–24 Ma (Fig. 6; Bröcker & Franz, Reference Bröcker and Franz2006).
5.d. Interpretation of the 40Ar–39Ar ages
These 40Ar–39Ar ages have been determined in phengite that apparently crystallized at 400–430°C and c. 7 kbar (Fig. 4b). The P-T estimates of domain 3 show no evidence of further heating. The plateau age at 29.8±0.6 Ma, for which no mixing and no perturbation of the argon system are recognized, is therefore considered as a crystallization age. This age overlaps with a phengite Rb–Sr age at 29.1±2.7 Ma (Fig. 6; Huyskens & Bröcker, Reference Huyskens and Bröcker2014). It dates the P-T conditions recorded in sample Andros2, which correspond to the end of the isobaric heating and the onset of exhumation in the greenschist facies.
6. Discussion
We here combine our pressure–temperature and time constraints with data from the literature in order to build the P-T-t paths of both the Attic–Cycladic Blueschist and Makrotantalon units and discuss their implications.
6.a. The P-T-t path of the Attic–Cycladic Blueschist unit on Andros
Since more information about the P-T-t evolution of the Attic–Cycladic Blueschist unit is available, it is discussed first. The available peak P-T conditions for this unit are 450–500°C and minimum pressure of 10 kbar (Reinecke, Reference Reinecke1986). Following Bröcker & Franz (Reference Bröcker and Franz2006), we use peak data from Tinos island – 500–520°C and 16–18 kbar (Parra, Vidal & Jolivet, Reference Parra, Vidal and Jolivet2002) – which is compatible with the wider peak range of 450–550°C and 12–20 kbar (Fig. 7a) determined by Bröcker (Reference Bröcker1990). The end of the exhumation under HP-LT conditions is constrained by the P-T estimates of group 1 (Fig. 7a; see Section 5.b), stopping at c. 300°C and 7 kbar. By comparison with the well-documented areas of the Attic–Cycladic Blueschist, this HP-LT exhumation can be bracketed between 35 Ma and 55 Ma (Jolivet & Brun, Reference Jolivet and Brun2010). The peak is then younger than 55 Ma and conditions of 300°C and 7 kbar are reached before 35 Ma.
Exhumation under HP-LT conditions is then followed by heating at constant 7 kbar pressure up to 420°C, as shown by P-T estimates of group 2 (Fig. 7a). P-T estimates of group 3 indicate nearly isothermal decompression at 400–420°C between 7 and 2 kbar. The onset of this second period of exhumation is constrained by our phengite 40Ar–39Ar plateau age of c. 30 Ma. The almost continuous geochronologic record by the phengite Rb–Sr method (Fig. 6) shows that it occurred between 30 Ma and 21 Ma. This is consistent with the discordant phengite 40Ar–39Ar spectra obtained in this study (Fig. 5f), which is interpreted as the effect of a phengite mixture between a population older than 28 Ma and a population younger than 23 Ma. The youngest phengite Rb–Sr age at 21 Ma is likely to date the end of ductile deformation at 350–400°C.
6.b. Possible P-T-t paths for the Makrotantalon unit
Our results constrain the HP-LT peak conditions in the Makrotantalon unit at 550°C, 18.5 kbar and 116 Ma (Fig. 7b). We suggest that this correlates with the Early Cretaceous blueschist metamorphism described in the Pelagonian region of continental Greece. The later evolution of the Makrotantalon unit is constrained by phengite Rb–Sr and 40Ar–39Ar ages and petrologic observations that are not tightly linked together.
-
1. The P-T conditions related to the 105 Ma re-equilibration are not known, but are most likely at lower grade than the peak conditions.
-
2. The phengite Rb–Sr ages between 70 and 90 Ma (Fig. 6; Bröcker & Franz, Reference Bröcker and Franz2006; Huyskens & Bröcker, Reference Huyskens and Bröcker2014) are the record of a metamorphic event that is contemporaneous with a widespread metamorphism and plutonism in Pelagonian unit (Patzak, Okrusch & Kreuzer, Reference Patzak, Okrusch and Kreuzer1994). This supports a Pelagonian origin for the Makrotantalon unit (Bröcker & Franz, Reference Bröcker and Franz2006; Huyskens & Bröcker, Reference Huyskens and Bröcker2014), but additional investigations are required to characterize the degree of thermal overprint related to this episode.
-
3. The phengite Rb–Sr ages in the contact area between the Makrotantalon and the Attic–Cycladic Blueschist units cluster at 40–45 Ma, which could date the stacking between the two units (Fig. 6; Huyskens & Bröcker, Reference Huyskens and Bröcker2014). According to these ages, the Makrotantalon unit was stacked on top of the Attic–Cycladic Blueschist unit during Eocene time while the latter was still experiencing HP-LT conditions and exhuming in the subduction zone below the Pelagonian unit that represented the upper plate (Trotet, Jolivet & Vidal, Reference Trotet, Jolivet and Vidal2001; Jolivet et al. Reference Jolivet, Faccenna, Huet, Labrousse, Le Pourhiet, Lacombe, Lecomte, Burov, Denèle, Brun, Philippon, Paul, Salaün, Karabulut, Piromallo, Monié, Gueydan, Okay, Oberhänsli, Pourteau, Augier, Gadenne and Driussi2012; Philippon, Brun & Gueydan, Reference Philippon, Brun and Gueydan2012). The Makrotantalon unit can therefore be considered as part of the upper plate of the subduction zone until 40–45 Ma, and its basal contact was not a thrust (Huyskens & Bröcker, Reference Huyskens and Bröcker2014) but a normal fault. The P-T conditions at which the two units were finally stacked together are of T>300°C and P>7 kbar. The Makrotantalon unit therefore followed the same P-T-t path as the Attic–Cycladic Blueschist unit after 40–45 Ma (Fig. 7b). This is consistent with two of the three P-T estimates in greenschists (Bröcker & Franz, Reference Bröcker and Franz2006).
-
4. The assemblages containing albite, lawsonite and pumpelleyite without glaucophane (Huyskens & Bröcker, Reference Huyskens and Bröcker2014) indicate that at least part of the Makrotantalon unit equilibrated at the boundary between the pumpelleyite-albite and the lawsonite blueschist facies. Petrological grids constrain this equilibration at c. 300°C and 7 kbar (Fig. 7b; Evans, Reference Evans1990), which corresponds (fortuitous or not) to the final HP-LT exhumation in the Attic–Cycladic Blueschist unit (Fig. 7a).
-
5. A wide range of meaningful Rb–Sr and 40Ar–39Ar ages and P-T conditions is preserved. A first interpretation is that the Makrotantalon unit is a tectonic mélange containing elements with different metamorphic histories. Alternatively, it indicates that both isotopic and petrologic systems have barely been perturbed at high temperature. We consider the first explanation as unlikely, since the distinctive Permian marble layer can be followed over several kilometres (Fig. 1b).
Based on these constraints and assuming that the Makrotantalon unit was coherent, we propose three possible P-T-t paths between 116 Ma and 40–45 Ma (Fig. 7c–e). The first scenario considers rapid post-peak exhumation of the Makrotantalon unit close to the surface (Fig. 7c). Low-pressure re-equilibration at 105 Ma would occur along this exhumation. The unit would then have been buried again and stacked with the Attic–Cycladic Blueschist unit in HP-LT conditions at 40–45 Ma, close to conditions where lawsonite and pumpelleyite are co-stable. There is no direct evidence of shallow exhumation of the Makrotantalon unit. However, Late Cretaceous and Paleocene flysch deposited above the Pelagonian unit contains detrital glaucophane (Faupl, Pavlopoulos & Migiros, Reference Faupl, Pavlopoulos and Migiros1999; Faupl et al. Reference Faupl, Petrakakis, Migiros and Pavlopoulos2002), indicating that blueschist-facies rocks were at the surface and eroded as early as c. 100 Ma (Faupl et al. Reference Faupl, Petrakakis, Migiros and Pavlopoulos2002). The Makrotantalon unit, or one of its lateral equivalent, could be the source of this detrital glaucophane. The second scenario considers rapid post-peak exhumation up to conditions where lawsonite and pumpelleyite are both stable (Fig. 7d). The Makrotantalon would then have been at stable P-T conditions in the hanging wall of the Hellenic subduction zones until partial exhumation of the Attic–Cycladic Blueschist unit over which it stacked at 40–45 Ma. The P-T evolution in the third scenario is similar (Fig. 7e), but the Makrotantalon exhumed slowly until stacking over the Attic–Cycladic Blueschist unit. This scenario implies long residence time at high temperature and is not entirely consistent with limited isotopic resetting.
6.c. Implications for the history of the Hellenic subduction zone
The HP-LT paragenesis described on Makrotantalon belongs, at least partly, to the Eohellenic phase. It is synchronous with the obduction of the Othrys ophiolite (110–130 Ma; Jacobshagen, Reference Jacobshagen1986) and the initiation of blueschist facies metamorphism in the Pelagonian unit (see Fig. 1a) at 100–125 Ma in the Ossa range (Lips, White & Wijbrans, Reference Lips, White and Wijbrans1998) and at 110–120 Ma on Evia island (Maluski et al. Reference Maluski, Vergely, Bavay, Bavay and Katsikatsos1981). The onset of blueschist facies metamorphism in the Olympos range could be younger (at least 100 Ma; Schermer, Reference Schermer1990; Schermer, Lux & Burchfiel, Reference Schermer, Lux and Burchfiel1990). This episode in continental Greece is closer to greenschist facies/blueschist facies transition (Schermer, Reference Schermer1990; Schermer, Lux & Burchfiel, Reference Schermer, Lux and Burchfiel1990; Lips, White & Wijbrans, Reference Lips, White and Wijbrans1998), that is, lower grade than the Makrotantalon unit on Andros. This Cretaceous event is younger than the Late Jurassic obduction of the Vourinos ophiolite and older than the obduction of Late Cretaceous Vardar–Izmir–Ankara ophiolite and the final closure of the Tethys Ocean. It confirms a continuous subduction and accretion in the Vardar–Izmir–Ankara suture zone south of the Rhodope since Early Cretaceous time.
These Early Cretaceous peak conditions in the Makrotantalon unit are similar to the Eocene peak conditions determined in the Attic–Cycladic Blueschist unit (see a review in Philippon, Brun & Gueydan, Reference Philippon, Brun and Gueydan2012). This may imply that the Hellenic subduction zone could have been at thermal steady state during Early Cretaceous – Eocene time. Exhumation of the HP-LT rocks in the subduction zone would have been a short-lived discontinuous phenomenon related to Early Cretaceous obduction and Eocene accretion of continental terrain. Such behaviour is consistent with a worldwide review of the dynamics of oceanic subduction zones (Agard et al. Reference Agard, Yamato, Jolivet and Burov2009).
6.d. Implications for the evolution of the North Cycladic Detachment System
The North Cycladic Detachment System (NCDS) acted as a normal fault exhuming the Attic–Cycladic Blueschist unit in the northern part of this unit (Jolivet et al. Reference Jolivet, Lecomte, Huet, Denele, Lacombe, Labrousse, Pourhiet and Mehl2010). It controlled both syn-orogenic (in the Hellenic subduction zone) and post-orogenic (in the back-arc of the Hellenic subduction zone) exhumation.
Figure 8a depicts the compiled P-T-t paths of the Attic–Cycladic Blueschist in the NCDS footwall: Olympos range (Schermer, Reference Schermer1990; Schermer, Lux & Burchfiel, Reference Schermer, Lux and Burchfiel1990); Ossa/Pelion range (Lips, White & Wijbrans, Reference Lips, White and Wijbrans1998; Lips, Wijbrans & White, Reference Lips, Wijbrans, White, Durand, Jolivet, Horvàth and Séranne1999; Lacassin et al. Reference Lacassin, Arnaud, Leloup, Armijo and Meyer2007); Evia island (Katzir et al. Reference Katzir, Avigad, Matthews, Garfunkel and Evans2000; Ring et al. Reference Ring, Glodny, Will and Thomson2010); Andros island (this study); and Tinos island (Parra, Vidal & Jolivet, Reference Parra, Vidal and Jolivet2002). Along-strike from NW to SE (Olympos, Ossa/Pelion, Evia, Andros, Tinos; Fig. 1a), conditions of Eocene HP-LT metamorphism and the maximum temperature during Oligo-Miocene exhumation both increase below the NCDS. This trend highlights a gradient of exhumation along the NCDS, where the deepest and warmest rocks are exhumed in the centre of the Aegean (Jolivet & Patriat, Reference Jolivet, Patriat, Durand, Jolivet, Horvàth and Séranne1999). Furthermore, two groups of P-T-t evolution can be distinguished. In continental Greece and on Evia Island decompression is accompanied by constant cooling, whereas in the Cyclades there is a break in exhumation accompanied with heating at constant pressure. The timing (before c. 37–30 Ma) and amount of heating (100–150°C) on each island is similar. This corresponds to thermal re-equilibration of the lithosphere between a cold syn-orogenic regime in the subduction zone and a warm post-orogenic regime in the back-arc of the subduction zone (Parra, Vidal & Jolivet, Reference Parra, Vidal and Jolivet2002), and coincides with a change of boundary conditions due to the onset of slab retreat (Jolivet & Faccenna, Reference Jolivet and Faccenna2000). Pressure of this thermal excursion (7 kbar on Andros and 9 kbar on Tinos) indicates that thermal re-equilibration occurred at lower crustal depth.

Figure 8. (a) Pressure–temperature–time path of the NCDS hanging wall in Olympos (Schermer, Reference Schermer1990; Schermer, Lux & Burchfiel, Reference Schermer, Lux and Burchfiel1990), Ossa-Pelion (Lips, White & Wijbrans, Reference Lips, White and Wijbrans1998; Lips, Wijbrans & White, Reference Lips, Wijbrans, White, Durand, Jolivet, Horvàth and Séranne1999; Lacassin et al. Reference Lacassin, Arnaud, Leloup, Armijo and Meyer2007), SE Evia (Katzir et al. Reference Katzir, Avigad, Matthews, Garfunkel and Evans2000; Ring et al. Reference Ring, Glodny, Will and Thomson2010), Andros (this study) and Tinos (Parra, Vidal & Jolivet, Reference Parra, Vidal and Jolivet2002). (b) Schematic cross-section indicating the upwards migration of localized strain in the NCDS.
Four detachments have been identified in the North Cycladic Detachment System (Fig. 8b; Jolivet et al. Reference Jolivet, Lecomte, Huet, Denele, Lacombe, Labrousse, Pourhiet and Mehl2010) as follows. (1) The Vari detachment (observed on Syros and Tinos) is an Eocene ductile low-angle normal fault active in the Hellenic subduction zone under HP-LT conditions. It was partly reactivated at 10 Ma. (2) The Tinos detachment (observed on Andros and Tinos) is an Oligo-Miocene (15–30 Ma) brittle–ductile low-angle normal fault. It localizes at the bottom of the upper unit. (3) The Livada detachment (observed on Tinos and Mykonos) is a low-angle brittle–ductile normal fault (14–10 Ma). It deforms syn-tectonic I-type granitoids which are 14–15 Ma old on Tinos (Altherr et al. Reference Altherr, Kreuzer, Wendt, Lenz, Wagner, Keller, Harre and Höhndorf1982; Avigad & Garfunkel, Reference Avigad and Garfunkel1989; Bröcker & Franz, Reference Bröcker and Franz1994; Brichau et al. Reference Brichau, Ring, Carter, Monie, Bolhar, Stockli and Brunel2007) and 13 Ma old on Mykonos (Altherr et al. Reference Altherr, Kreuzer, Wendt, Lenz, Wagner, Keller, Harre and Höhndorf1982; Brichau et al. Reference Brichau, Ring, Carter, Bolhar, Monie, Stockli and Brunel2008) and localizes within the upper unit above these intrusions. (4) The Mykonos detachment is a low-angle brittle normal fault localizing in the upper unit and at the bottom of Miocene clastic sediments (Lecomte et al. Reference Lecomte, Jolivet, Lacombe, Denèle, Labrousse and Le Pourhiet2010), and was active between 14 and 9 Ma. All these detachment have a top-to-the-N or -NE kinematics.
Based on similar structural position and timing, we correlate the contact at the bottom of the Makrotantalon unit with the Vari detachment. Incorporation of the Makrotantalon unit within the Attic–Cycladic Blueschist at 40–45 Ma suggests that this unit moved from the hanging wall of the NCDS to its footwall during syn-orogenic exhumation. Localized strain in the NCDS jumped structurally higher in the Tinos detachment (at the bottom of the Pelagonian ophiolite), the activity of which was constrained by phengite Rb–Sr ages of 24–28 Ma (Huyskens & Bröcker, Reference Huyskens and Bröcker2014). This jump is coeval with the thermal re-equilibration that occurred before 35–30 Ma. We suggest that this jump is controlled by both uplifting of the brittle–ductile transition (Jolivet et al. Reference Jolivet, Lecomte, Huet, Denele, Lacombe, Labrousse, Pourhiet and Mehl2010) and strong strength contrasts at the bottom of the Pelagonian ophiolite (Huet et al. Reference Huet, Pourhiet, Labrousse, Burov and Jolivet2011a , Reference Huet, Le Pourhiet, Labrousse, Burov and Jolivet b ). Localized strain has again migrated upward within the NCDS: in the Livada detachment and in the Mykonos detachment (Fig. 8b, Jolivet et al. Reference Jolivet, Lecomte, Huet, Denele, Lacombe, Labrousse, Pourhiet and Mehl2010). In both cases, migration is controlled by successive uplifting of the brittle–ductile transition favoured by intrusion of warm material.
Active deformation in the NCDS has therefore always migrated upward and each uplift event can be related to a perturbation of the thermal state in the crust. These brutal migrations of the localized strain are consistent with the predictions of thermomechanical models showing that long-lived detachment zones are made of anastomosed shear zones, which are active at different times of the activity of the detachment (Huet et al. Reference Huet, Pourhiet, Labrousse, Burov and Jolivet2011a , Reference Huet, Le Pourhiet, Labrousse, Burov and Jolivet b ). We therefore suggest that deformation in a long-lived crustal-scale normal fault tends to migrate upwards; this is contrary to a thrust, in which case it migrates downwards.
7. Conclusion
Phengite 40Ar–39Ar geochronology coupled with thermobarometry is a powerful method that allows the tectono-metamorphic evolution of Andros Island to be determined. The Makrotantalon unit experienced HP-LT metamorphism with peak conditions at 550°C and 18.5 kbar at 116 Ma. This correlates with Early Cretaceous blueschist facies metamorphism in the Pelagonian area of continental Greece and favours a Pelagonian origin for the Makrotantalon unit. This unit is now present as a Pelagonian sliver, stacked over to the Attic–Cycladic Blueschist in the Hellenic subduction zone at 40–45 Ma. The P-T evolution of the Attic–Cycladic Blueschist unit is characterized by an exhumation after peak conditions under HP-LT conditions at 55–35 Ma, isobaric heating at 7 kbar until 30 Ma and then isothermal decompression until 21 Ma. These P-T-t evolutions confirm that transition between a stable regime with thermal steady state and a retreating regime in the Hellenic subduction zone occurred between 35 and 30 Ma. It also confirms that the NCDS is a complex structure in which active deformation migrated upwards, favoured by uplifting of the brittle–ductile transition and controlled by thermal perturbations. This study highlights that P-T estimates tightly linked to radiochronology are essential for understanding complex tectono-metamorphic evolutions.
Acknowledgments
This work has been funded by the ANR-EGEO project. The reviewer Roland Oberhänsli is thanked for his comments that allowed us to improve the quality of the manuscript.