1. Introduction
Cretaceous oceanic anoxic events (OAEs) have received considerable attention during the last couple of decades and a wealth of information has been obtained on the age and duration, palaeoceanography, biogeochemistry and trigger mechanisms of these episodes (e.g. Jenkyns, Reference Jenkyns2010). The so-called OAE 1a (locally known as Livello Selli, Niveau Goguel, Fisch-schiefer) took place during early Aptian time (126.3–123.0 Ma; Gradstein et al. Reference Gradstein, Ogg, Schmitz and Ogg2012) and spanned an interval of approximately 1.0–1.3 Ma (Li et al. Reference Li, Bralower, Montanez, Osleger, Arthur, Bice, Herbert, Erba and Silva2008) or 1.11 ± 0.11 Ma (Malinverno, Erba & Herbert, Reference Malinverno, Erba and Herbert2010). In pelagic and hemipelagic settings, OAE 1a is typically expressed as a stratigraphic sequence of finely laminated, organic carbon-rich deposits formed under anoxic (Quijano et al. Reference Quijano, Castro, Pancost, de Gea, Najarro, Aguado, Rosales and Martin-Chivelet2012) or even euxinic conditions (Pancost et al. Reference Pancost, Crawford, Magness, Turner, Jenkyns and Maxwell2004). In the neritic realm, the time-equivalent deposits are characterized either by a phase of non-deposition or erosion (Wissler, Funk & Weissert, Reference Wissler, Funk and Weissert2003; Föllmi et al. Reference Föllmi, Godet, Bodin and Linder2006) or by the growth of a micro-encrusting microbial and/or algal community (Immenhauser, Hillgärtner & Van Betum, Reference Immenhauser, Hillgärtner and Van Betum2005; Bover-Arnal et al. Reference Bover-Arnal, Salas, Martin-Closas, Schlagintweit and Moreno-Bedmar2011; Huck, Heimhofer & Immenhauser, Reference Huck, Heimhofer and Immenhauser2012). The carbon isotope pattern across the OAE 1a is characterized by a rapid, negative δ13C excursion followed by a long-lasting positive shift and provides a valuable geochemical signature for high-resolution correlation of marine strata (Menegatti et al. Reference Menegatti, Weissert, Brown, Tyson, Farrimond, Strasser and Caron1998; Herrle et al. Reference Herrle, Kössler, Friedrich, Erlenkeuser and Hemleben2004; Robinson et al. Reference Robinson, Clarke, Nederbragt and Wood2008; Millán et al. Reference Millán, Weissert, Fernandez-Mendiola and Garcia-Mondejar2009; Vahrenkamp, Reference Vahrenkamp, Van Buchem, Al-Husseini, Maurer and Droste2010; Moreno-Bedmar et al. Reference Moreno-Bedmar, Company, Sandoval, Tavera, Bover-Arnal, Salas, Delanoy, Maurrasse and Martinez2012; Gaona-Narvaez, Maurrasse & Moreno-Bedmar, Reference Gaona-Narvaez, Maurrasse and Moreno-Bedmar2013; Yamamoto et al. Reference Yamamoto, Ishibashi, Takayanagi, Asahara, Sato, Nishi and Iryu2013).
The widespread accumulation of high amounts of sedimentary organic matter (OM) during this event has been linked to peculiar oceanographic and climatic conditions. Whereas the OAE 1a itself has been proposed to reflect an episode of exceptional warmth triggered by volcanic activity (Larson & Erba, Reference Larson and Erba1999; Méhay et al. Reference Méhay, Keller, Bernasconi, Weissert, Erba, Bottini and Hochuli2009; Bottini et al. Reference Bottini, Cohen, Erba, Jenkyns and Coe2012), enhanced organic carbon sequestration in marine sediments has been interpreted to result in CO2 drawdown and subsequent cooling (Hochuli et al. Reference Hochuli, Menegatti, Weissert, Riva, Erba and Premoli Silva1999; Bellanca et al. Reference Bellanca, Erba, Neri, Premoli, Sprovieri, Tremolada and Verga2002; Ando et al. Reference Ando, Kaiho, Kawahata and Kakegawa2008; Jenkyns, Reference Jenkyns2010). Episodes of exceptional climatic warmth (haline euxinic acidic thermal transgression or HEATT episodes of Kidder & Worsley, Reference Kidder and Worsley2010) are expected to have had a pronounced effect on the association and distribution of terrestrial vegetation. Prominent changes in floral compositions associated with major carbon-cycle perturbations have been reported for the Palaeocene–Eocene thermal maximum (Wing et al. Reference Wing, Harrington, Smith, Bloch, Boyer and Freeman2005; Jaramillo et al. Reference Jaramillo, Ochoa, Contreras, Pagani, Carvajal-Ortiz, Pratt, Krishnan, Cardona, Romero, Quiroz, Rodriguez, Rueda, de la Parra, Moron, Green, Bayona, Montes, Quintero, Ramirez, Mora, Schouten, Bermudez, Navarrete, Parra, Alvaran, Osorno, Crowley, Valencia and Vervoort2010), the Triassic–Jurassic transition (McElwain, Beerling & Woodward, Reference McElwain, Beerling and Woodward1999; Bonis, Kürschner & Krystyn, Reference Bonis, Kürschner and Krystyn2009; van de Schootbrugge et al. Reference van de Schootbrugge, Quan, Lindstrom, Puettmann, Heunisch, Pross, Fiebig, Petschick, Roehling, Richoz, Rosenthal and Falkowski2009) and the Permian–Triassic warming event (Looy et al. Reference Looy, Brugman, Dilcher and Visscher1999; Hochuli et al. Reference Hochuli, Os Vigran, Hermann and Bucher2010; Hermann et al. Reference Hermann, Hochuli, Bucher, Brühwiler, Hautmann, Ware and Roohi2011). Given the proposed climatic impact of Cretaceous OAEs on the entire ocean–atmosphere system, a better understanding of the response of continental vegetation composition and dynamics is highly relevant. The high sea-surface temperatures (SSTs) proposed for tropical and subtropical sites for the early Aptian OAE 1a (Schouten et al. Reference Schouten, Hopmans, Forster, van Breugel, Kuypers and Damsté2003; Dumitrescu et al. Reference Dumitrescu, Brassel, Schouten, Hopmans and Sinninghe Damsté2006; Ando et al. Reference Ando, Kaiho, Kawahata and Kakegawa2008) would imply even higher (surface air) temperatures for continental settings during this event. Temperature extremes during late Early Cretaceous time may have exceeded the heat tolerance of low-latitude terrestrial ecosystems at times, resulting in the apparent sparseness of land plant vegetation in the tropical-equatorial hot arid belt (Chumakov et al. Reference Chumakov, Zharkov, Herman, Doludenko, Kalandadze, Lebedev, Ponomarenko and Rautian1995; Hay & Floegel, Reference Hay and Floegel2012).
In contrast to the marine record of the OAE 1a, information on the response of terrestrial ecosystems to this exceptional event is relatively scarce. This is mainly due to the lack of suitable and well-dated continental archives covering the early Aptian time span. In marine settings, the overwhelming occurrence of algal and/or bacterial-derived amorphous OM within the black shale horizons often hampers accurate palynological analysis and a quantitative assessment of spore-pollen assemblages. In addition, due to their distance to the coast, pelagic deposits often contain only a small portion of continent-derived spores and pollen, with the assemblages being potentially biased due to long transport distances (Heusser & Balsam, Reference Heusser and Balsam1977; Tyson, Reference Tyson1995; Traverse, Reference Traverse2007). Only a few records exist at this stage, which are essentially based on spore-pollen data from stratigraphically well-constrained marine sections located along the northern margin of the western Tethys (Hochuli et al. Reference Hochuli, Menegatti, Weissert, Riva, Erba and Premoli Silva1999; Heimhofer et al. Reference Heimhofer, Hochuli, Herrle, Andersen and Weissert2004, Reference Heimhofer, Hochuli, Herrle and Weissert2006; Keller et al. Reference Keller, Hochuli, Weissert, Bernasconi, Giorgioni and Garcia2011; Najarro et al. Reference Najarro, Rosales, Moreno-Bedmar, de Gea, Barron, Company and Delanoy2011). Whereas most of the latter studies focus essentially on the OAE 1a interval itself and on pre-OAE strata, only Hochuli et al. (Reference Hochuli, Menegatti, Weissert, Riva, Erba and Premoli Silva1999) provide a palynological record covering the entire event and its aftermath.
In this study, we present a spore-pollen record covering the entire early Aptian OAE 1a interval and its aftermath. Palynofacies analysis provides information on sea-level changes and any potential bias of the spore-pollen dataset due to hydrodynamic sorting. The studied succession deposited in the Maestrat Basin of eastern Spain is bio- and chemostratigraphically well constrained, which allows a comparison of changes in the palynoflora with existing spore-pollen records across OAE 1a, as well as with palaeoclimatic proxy data from the continental and marine realms.
2. Geological setting
Lower Cretaceous marine and continental sediments are well exposed in the Galve sub-basin of the Maestrat Basin in the eastern Iberian Chain of Spain. During Late Jurassic and Early Cretaceous times, extension of the Iberian plate due to the opening of the Central and North Atlantic domains resulted in rifting and in the formation of several intraplate basins, including the Maestrat Basin and its sub-basins (Fig. 1; Salas et al. Reference Salas, Guimera, Mas, Martin-Closas, Melendez, Alonso, Ziegler, Cavazza, Robertson and CrasquinSoleau2001). Here, deposition of terrigenous siliciclastics and epeiric carbonates took place under differential subsidence in a complex tectonic setting, resulting in extraordinary sedimentary thicknesses (Bover-Arnal et al. Reference Bover-Arnal, Moreno-Bedmar, Salas, Skelton, Bitzer and Gili2010). Tectonic inversion during the Alpine Orogeny caused faulting, uplift and the formation of the Iberian Chain (Salas et al. Reference Salas, Guimera, Mas, Martin-Closas, Melendez, Alonso, Ziegler, Cavazza, Robertson and CrasquinSoleau2001).

Figure 1. Palaeogeographic reconstruction of the western Tethys region during late Early Cretaceous time (modified after Ziegler, Reference Ziegler1990). Distribution of major climate belts after Chumakov et al. (Reference Chumakov, Zharkov, Herman, Doludenko, Kalandadze, Lebedev, Ponomarenko and Rautian1995). Study site is marked with an asterisk. AM – Armorica Massif; IBM – Iberian Massif; MC – Massif Central; MM – Morocco Meseta; RM – Rhenish Massif.
The studied Barranco de las Calzadas (BdlC) section is located in the province of Teruel, about 5 km NE of the village Villarroya de los Pinares. The succession is accessible in outcrop on the eastern limb of the NNW–SSE-striking Miravete anticline. Here, about 450 m of early Aptian (Bedoulian) deposits are subdivided into the Morella, Xert, Forcall and Villarroya de los Pinares formations (Canérot et al. Reference Canérot, Cugny, Pardo, Salas, Villena and García1982). The investigated section covers 150 m of the Forcall Formation and is essentially composed of epeiric marine marls with intercalations of orbitolinid-rich, nodular and sand-rich limestone beds. The succession represents part of a large-scale transgressive–regressive sequence (Bover-Arnal et al. Reference Bover-Arnal, Moreno-Bedmar, Salas, Skelton, Bitzer and Gili2010; Embry et al. Reference Embry, Vennin, Buchem, Schroeder, Pierre, Aurell, Van Buchem, Gerdes and Esteban2010). In the lower part of the Forcall Formation (305–311 m), a conspicuous carbonate horizon composed of coral rubble encrusted by Lithocodium aggregatum is noteworthy (Bover-Arnal et al. Reference Bover-Arnal, Salas, Martin-Closas, Schlagintweit and Moreno-Bedmar2011).
The stratigraphic assignment of the lower Aptian succession in the eastern Iberian Chain is well constrained based on magnetostratigraphy (Salas et al. Reference Salas, Martín-Closas, Delclòs, Guimerà, Caja and Mas2005), carbon isotope stratigraphy based on bulk carbonate (Bover-Arnal et al. Reference Bover-Arnal, Moreno-Bedmar, Salas, Skelton, Bitzer and Gili2010; Embry et al. Reference Embry, Vennin, Buchem, Schroeder, Pierre, Aurell, Van Buchem, Gerdes and Esteban2010) and ammonite biostratigraphy (Moreno-Bedmar et al. Reference Moreno-Bedmar, Company, Bover-Arnal, Salas and Delanoy2009, Reference Moreno-Bedmar, Company, Bover-Arnal, Salas, Delanoy, Maurrasse, Grauges and Martinez2010). The Forcall Formation is dated by ammonites as early Aptian (Bedoulian) and comprises the complete Deshayesites forbesi and Deshayesites deshayesi zones and the lower part of the Dufrenoyia furcata biozone (Moreno-Bedmar et al. Reference Moreno-Bedmar, Company, Bover-Arnal, Salas, Delanoy, Maurrasse, Grauges and Martinez2010). Additional stratigraphic refinement is obtained from carbon isotope data, which show a characteristic early Aptian trend with stratigraphic height (Moreno-Bedmar et al. Reference Moreno-Bedmar, Company, Bover-Arnal, Salas and Delanoy2009; Bover-Arnal et al. Reference Bover-Arnal, Moreno-Bedmar, Salas, Skelton, Bitzer and Gili2010;). However, despite the excellent match of the existing carbon isotope curve with published reference records (Menegatti et al. Reference Menegatti, Weissert, Brown, Tyson, Farrimond, Strasser and Caron1998; Gaona-Narvaez, Maurrasse & Moreno-Bedmar, Reference Gaona-Narvaez, Maurrasse and Moreno-Bedmar2013), the stratigraphic significance of the abrupt negative shift (C3) in the record of Bover-Arnal et al. (Reference Bover-Arnal, Moreno-Bedmar, Salas, Skelton, Bitzer and Gili2010) remains questionable. Given the distinct lithological changes associated with this short-lived negative shift, a diagenetic bias of the signature cannot be ruled out.
3. Palaeogeography and palaeophytogeography
During late Early Cretaceous time, the progressive fragmentation of Laurasia in combination with high sea level resulted in the formation of an archipelago composed of isolated emergent landmasses along the northwest margin of the Alpine Tethys Ocean (Hay et al. Reference Hay, DeConto, Wold, Wilson, Voigt, Schulz, Wold, Dullo, Ronov, Balukhovsky, Soeding, Barrera and Johnson1999). The Iberian Massif was situated at the southwest tip of this archipelago at an approximate palaeolatitude of 30–35° N (Sewall et al. Reference Sewall, de Wal, van der Zwan, van Oosterhout, Dijkstra and Scotese2007). During Aptian time, the Iberian Massif formed an isolated, low-relief island surrounded by intracratonic basins (including the Maestrat Basin) covered by shallow epeiric seas. Towards the southeast, these epicontinental seas passed into the deep oceanic realm of the Alpine Tethys Ocean. The Iberian landmass was probably the main source for all types of erosional products as well as for land plant-derived debris and sporomorphs deposited in the Maestrat Basin.
Climatic conditions during Aptian time were characterized by global warmth as indicated by low meridional thermal gradients, reduced seasonality, high atmospheric CO2 levels and the absence of large continental ice sheets (Huber, Hodell & Hamilton, Reference Huber, Hodell and Hamilton1995; Price, Reference Price1999; Herman & Spicer, Reference Herman and Spicer2010; Jenkyns et al. Reference Jenkyns, Schouten-Huibers, Schouten and Sinninghe Damsté2012). The Iberian Massif was situated in a transitional position between two major climatic zones: the tropical-equatorial hot arid belt in the south and the mid-latitude warm humid belt in the north (Chumakov et al. Reference Chumakov, Zharkov, Herman, Doludenko, Kalandadze, Lebedev, Ponomarenko and Rautian1995; Skelton et al. Reference Skelton, Spicer, Kelley and Gilmour2003). This resulted in a subtropical, seasonally dry (semi-arid to arid) climate in most parts of Iberia during late Early Cretaceous time (Ruffell & Batten, Reference Ruffell and Batten1990; Heimhofer et al. Reference Heimhofer, Adatte, Hochuli, Burla and Weissert2008; Diéguez, Peyrot & Barrón, Reference Diéguez, Peyrot and Barrón2010). Consequently, the vegetation of this winterwet biome was dominated by xerophytic forests composed of microphyllous conifers and diverse fern communities forming the understory. Additional floral elements are seed ferns, ginkgoales, horsetails, lycopsids and, of subordinate importance, early representatives of the angiosperms (Solé de Porta et al. Reference Solé de Porta, Querol, Cabanes and Salas1994; Diéguez, Peyrot & Barrón, Reference Diéguez, Peyrot and Barrón2010; Friis, Pedersen & Crane, Reference Friis, Pedersen and Crane2010).
4. Materials and methods
A total of 50 samples were collected at regular levels throughout the BdlC section, Maestrat Basin, eastern Spain, resulting in a sample spacing of 2.5 m. In order to minimize contamination by modern plant debris and/or near-surface weathering processes, the uppermost 15–20 cm of sediment were removed before sampling. Following collection, the sample material was dried at 40 °C in a laboratory oven before further processing.
4.a. Carbon and oxygen isotopes
Measurement of stable carbon and oxygen isotopes of sedimentary carbonates was carried out on powdered bulk sample material (c. 0.5 mg) on a total of 50 samples. An additional 18 samples were taken from a hand-specimen collected from a Lithocodium-rich limestone unit (305–311 m) in order to investigate the significance of the negative shift, which marks the onset of the OAE 1a. Stable isotope analysis was conducted using a Thermo Fisher Scientific Gasbench II carbonate device connected to a Thermo Fisher Scientific Delta 5 Advantage isotope ratio mass spectrometer, available at the Leibniz University, Hannover, Germany. The gas bench uses viscous water-free (98 g mol−1) orthophosphoric acid at 72 °C to release CO2 of the calcite from the sample material 1 h before the start of the measurement. Repeated analyses of certified carbonate standards (CO-1, NBS-18, NBS-19) show an external reproducibility ± 0.1 ‰ for δ18O and ± 0.08 ‰ for δ13Ccarb. Values are expressed in conventional delta notation relative to the Vienna-Pee Dee Formation belemnite (VPDB) international standard, in parts per mil (‰). A total of nine duplicate measurements shows that the measured values are representative and indicate that the samples are quite homogenous (maximum deviation for duplicate measurements is ± 0.2 for δ18O and ± 0.08 for δ13C).
4.b. Clay minerals
A total of 37 samples from the BdlC section were analysed for their clay mineralogy. Clay mineral analyses were based on methods described by Kübler (Reference Kübler1987). Ground chips were mixed with deionized water (pH 7–8) and agitated. The carbonate fraction was removed by addition of 10 % HCl at room temperature for 20 min or longer until all the carbonate was dissolved. Ultrasonic disaggregation was accomplished during 3 min intervals. The insoluble residue was washed and centrifuged (5–6 times) until a neutral suspension was obtained (pH 7–8). Separation of two different grain size fractions (<2 μm and 2–16 μm) was obtained by the timed settling method based on Stokes’ law. The selected fraction was then pipetted onto a glass plate and air dried at room temperature. X-ray diffraction (XRD) analysis of oriented clay samples were carried out after air drying at room temperature and under ethylene-glycol solvated conditions. The intensities of selected XRD peaks characterizing each clay mineral present in the size fraction (e.g. chlorite, mica, kaolinite and illite-smectite mixed layers) were measured for a semi-quantitative estimate of the proportion of clay minerals present in two size fractions (<2 μm and 2–16 μm). Determination of the illite-smectite mixed layers and their content in smectitic expandable layers is based on the method of Moore & Reynolds (Reference Moore and Reynolds1997).
4.c. Palynology
A total of 38 rock samples from the BdlC section were prepared for palynological analysis by the Geological Survey of North Rhine-Westphalia in Krefeld, Germany. Cleaned, crushed and weighed samples (20–50 g) were treated with HCl and HF following standard palynological preparation techniques (e.g. Traverse, Reference Traverse2007). All 38 productive samples were studied for their particle content (palynofacies) and sporomorph assemblage (spores and pollen). For analysis of the palynofacies, a minimum of 300 particles was counted from strew mounts. For the spore-pollen assemblage, a minimum of 200 grains were determined per slide. Variations in the spore-pollen assemblage represent normalized frequencies and are reported as a percentage (%) of the total assemblage. Light photomicrographs were taken using an Olympus BX 53F light microscope (LM) equipped with an integrated 5 megapixel Olympus XC50 digital camera. The preservation of the studied palynomorphs varies from moderate to very good. Thermally unaltered preservation of organic matter is indicated by the virtually unchanged colouring of the palynomorphs and shows a thermal alteration index (TAI) <2 according to Staplin (Reference Staplin and Staplin1982).
5. Results
5.a. Stable isotope geochemistry
Carbon isotope values vary between −0.3 ‰ and +5.5 ‰ and show a distinct negative–positive trend with stratigraphic height (Fig. 2). The lowermost part of the section is characterized by δ13C values ranging between +0.2 ‰ and +1.0 ‰ (277–285 m; segment C2). This interval is followed by a gradual negative shift to minimum values of −0.3 ‰ (285–296 m; segment C3) and a subsequent positive trend reaching +2.6 ‰ (296–312 m; segment C4). For the following 10 m of section, values remain comparatively stable varying between +2.6 ‰ and +3.6 ‰ (312–322 m; segment C5), followed by an additional small-step increase (322–325 m; segment C6). The overlying stratigraphic interval (325–389 m; segment C7) is characterized by an increase in δ13C up to peak values of +5.5 ‰. The remaining part of the succession shows a gradual trend towards less positive δ13C values. The general pattern of the carbon isotope record matches well with the curve published previously by Bover-Arnal et al. (Reference Bover-Arnal, Moreno-Bedmar, Salas, Skelton, Bitzer and Gili2010) for the same section. Integration of the new carbon isotope data with the existing δ13C dataset results in an improved high-resolution record for the BdlC section. The new measurements confirm the general stratigraphic trend of the existing δ13C record and its principal chemostratigraphic interpretation (Moreno-Bedmar et al. Reference Moreno-Bedmar, Company, Bover-Arnal, Salas and Delanoy2009; Bover-Arnal et al. Reference Bover-Arnal, Moreno-Bedmar, Salas, Skelton, Bitzer and Gili2010).
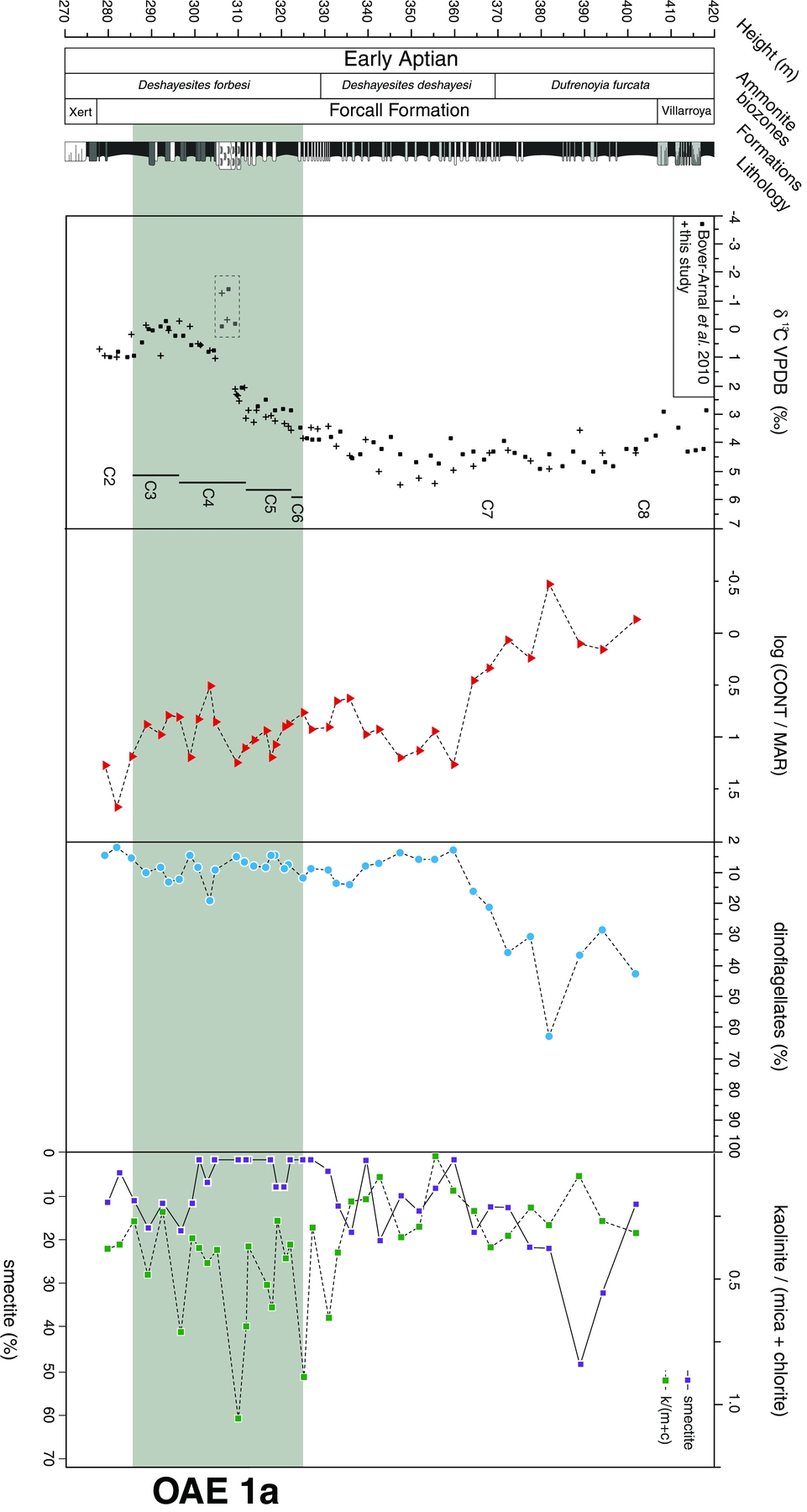
Figure 2. Carbon isotope curve based on bulk carbonate, log CONT/MAR ratio, dinoflagellate cyst abundance, smectite content and kaolinite/(mica + chlorite) ratio plotted against a lithostratigraphic log and biostratigraphic information of the BdlC section, Maestrat Basin, eastern Spain. Litho- and biostratigraphy after Moreno-Bedmar et al. (Reference Moreno-Bedmar, Company, Bover-Arnal, Salas and Delanoy2009). Carbon isotope data represent a composite curve based on Bover-Arnal et al. (Reference Bover-Arnal, Moreno-Bedmar, Salas, Skelton, Bitzer and Gili2010) and this study. Negative carbon isotope values framed by stippled line represent measurements from the Lithocodium-rich limestone unit and are excluded from chemostratigraphic interpretation.
5.b. Palynofacies
In the studied samples, the particulate organic matter is dominated by terrestrial phytoclasts (average 56.9 %), which account for 14.2–84.1 %. Both opaque and translucent phytoclast particles occur in roughly equal abundances. Plant-derived gymnosperm pollen (with angiosperm pollen being of only subordinate importance) vary between 3.5 and 31.3 % (average 12.5 %), spores are less abundant and reach only 0.3–5.5 % (average 2.3 %). Particles of marine origin are dominated by dinoflagellate cysts in the range 1.9–62.8 % (average 13.5 %). Acritarchs and foraminifera test linings account for only a small fraction. Amorphous organic matter is composed of orange-brown to grey flakes and represents a consistent fraction of the particulate organic matter (average 6.2 %).
Based on the quantitative palynofacies analysis, two parameters are used to provide information on the stratigraphic evolution of particulate organic matter sedimentation in the BdlC section. The ratio of continent-derived to marine-derived particles (log CONT/MAR) is a proxy for distinguishing transgressive–regressive trends (Steffen & Gorin, Reference Steffen and Gorin1993; Pellaton & Gorin, Reference Pellaton and Gorin2005). In addition, the relative abundance of dinoflagellate cysts is used as an indication for increasing marine influence (Tyson, Reference Tyson1995; Pellaton & Gorin, Reference Pellaton and Gorin2005). The CONT/MAR ratio shows a distinct decrease versus stratigraphic height with a strong dominance of terrestrial particles (CONT/MAR ratio up to 1.7) in the lowermost part of the succession (277–285 m; Figs 2, 3a). The middle part (285–360 m) displays lower CONT/MAR ratios of 0.5–1.3, whereas the uppermost marl-rich part of the section (360–402 m) is characterized by a decrease towards low ratios ranging between 0.5 and −0.5. As expected, the relative percentage of dinoflagellate cysts shows an opposing pattern with low abundances (1.9–14.1 %; average 8.0 %) in the lower and middle part of the section. The upper part (360–402 m) is characterized by a strong increase in dinoflagellate cyst abundance (average 32.1 %) reaching peak values of 62.8 % at 382 m (Figs 2, 3b). All palynofacies data are available in the form of online Supplementary Material at http://journals.cambridge.org/geo.

Figure 3. Photomicrographs of two selected palynofacies samples illustrating changes in particulate organic matter composition. (a) Sample MA-4 (285.0 m) showing a composition strongly dominated by translucent and opaque phytoclasts resulting in a CONT/MAR ratio of 15.5. (b) Sample CA-21 (381.7 m) displaying high abundances of intact and broken dinoflagellate cysts with a CONT/MAR ratio of 0.3. Scale bar is 50 μm.
5.c. Clay mineralogy
In the BdlC section, clay mineral assemblages are mostly composed of smectite, kaolinite, mica (illite) with minor chlorite and illite-smectite mixed layer (IS) components (Fig. 2). The lowest part of the section (C2) is dominated by mica (60–70 %) and kaolinite (20–25 %) with lower abundances of chlorite (5–7 %); smectite and illite-smectite mixed layers (IS) range between 2 and 12 %. The C3 interval is characterized by increased smectite (up to 25 %) and IS contents to the detriment of mica and, to a lesser extent, kaolinite and chlorite. From C4 upwards, a notable decrease in smectite coincides with higher chlorite contents reaching up to 15 % in the upper part of C4. Kaolinite and chlorite show an increase in the C5 and C6 intervals (up to 40 % and 25 %, respectively), while smectite is rarely present within this interval. The lower part of C7 interval is dominated by mica (50–70 %) and shows a distinct decrease in kaolinite, which reaches minimum values (0–20 %), whereas smectite remains low with fluctuating contents of <25 %. The upper part of C7 is marked by a gradual increase in smectite (up to 50 %) paralleled by a decline in mica, whereas kaolinite and IS remain low and constant (5–25 %). The overall evolution of the clay mineral assemblage is well expressed in the kaolinite to mica plus chlorite ratio (K/(M + C)). All clay mineral data are available in the form of online Supplementary Material at http://journals.cambridge.org/geo.
5.d. Palynology
The sedimentary deposits of the BdlC section contain a rich and diverse terrestrial palynoflora composed of bisaccate and non-saccate gymnosperm pollen, pteridophyte spores and very few angiosperm pollen. Most spores and pollen were determined on the generic level. In the studied record, 17 genera of gymnosperm pollen (including 3 species), 17 genera of spores and two types of angiosperm pollen can be differentiated (Fig. 4). The assemblage is strongly dominated by non-saccate conifer pollen, which account for 88.6 % of the total assemblage on average. Bisaccate pollen grains (including conifer-derived Alisporites spp., Parvisaccites spp. and Podocarpidites spp. as well as the pteridosperm pollen Vitreisporites pallidus) account for less than 2.0 % and show only minor variations with stratigraphic height. Despite a relatively high diversity, spores produced by bryophytes (Stereisporites spp.), lycopsids (Leptolepidites spp., Retitriletes spp.) and ferns make up less than 9.0 % on average. Among the fern spores, the most abundant taxa include Cicatricosisporites spp. (average 2.8 %), Cyathidites spp. (average 1.0 %) and Deltoidospora spp. (average 0.8 %). The low and stable total spore content is well reflected in a high and constant pollen-spore ratio, which characterizes the entire succession (Fig. 4). The assemblage of non-saccate conifer pollen is dominated by Classopollis pollen, accounting for an overwhelming 63.6 % on average. Other quantitatively important forms produced by conifers include Inaperturopollenites spp. (up to 31.6 %; average 11.6 %), Araucariacites spp (up to 20.1 %; average 6.5 %) and Callialasporites spp (including C. damperi and C. trilobatus; up to 5.7 %; average 2.4 %). Additional gymnosperm pollen of bennettitalean affinity such as Exesipollenites spp. (up to 4.7 %) or ginkoalean/cycadalean affinity such as Cycadopites spp. (up to 6.0 %) are only of subordinate importance (average 1.2 % and 1.5 %, respectively). Similarly, pollen produced by gnetalean plants (Ephedripites spp, Steevesipollenites spp.) account for less than 0.5 % of the total sporomorph assemblage.
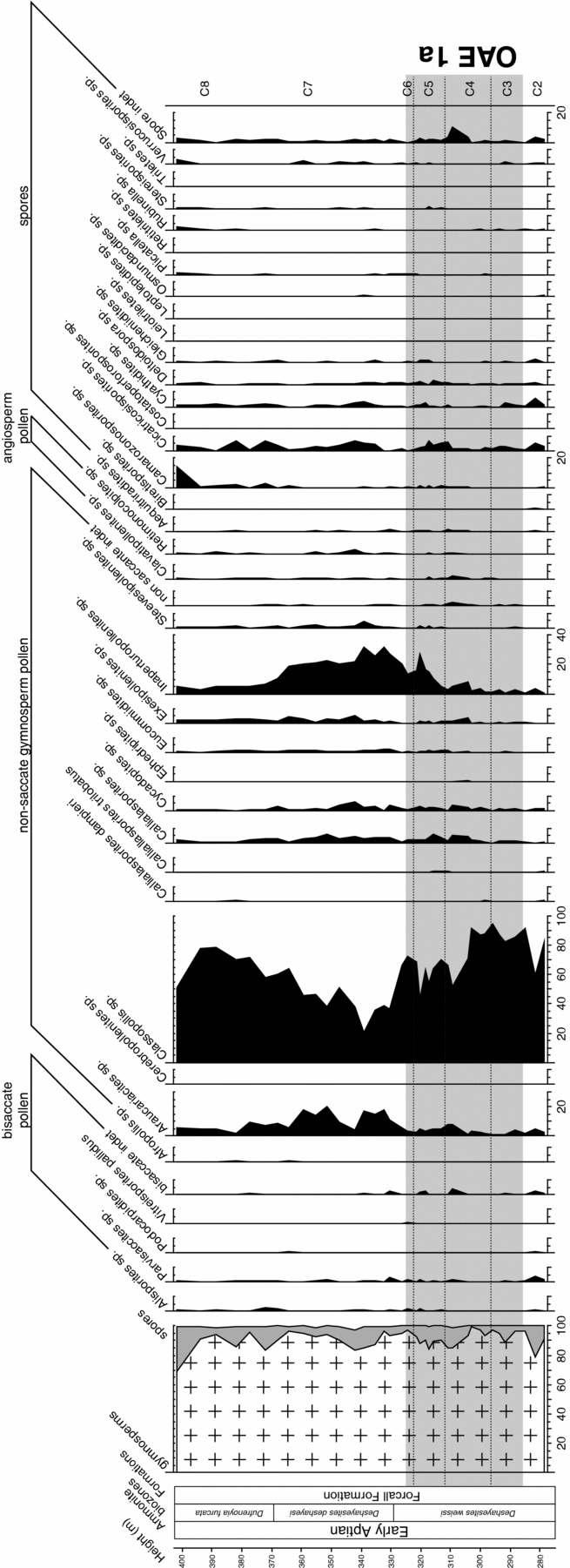
Figure 4. Relative abundances (%) of spores and pollen in the BdlC section, Maestrat Basin, eastern Spain. Litho- and biostratigraphy after Moreno-Bedmar et al. (Reference Moreno-Bedmar, Company, Bover-Arnal, Salas and Delanoy2009). Grey shaded bar corresponds to the OAE 1a interval.
Together, the three conifer pollen taxa Classopollis spp., Inaperturopollenites spp. and Araucariacites spp. make up 61.1–96.6 % of the total sporomorph assemblage. Interestingly, these three groups show significant variations in normalized frequency distribution with stratigraphic height. In the lowermost part of the succession (278.8–303.0 m), Classopollis dominates the assemblages reaching up to 94.5 % (average 84.8 %), whereas Araucariacites spp. and Inaperturopollenites spp. remain below 4.9 % and 3.8 %, respectively. In the subsequent interval (304.3–324.6 m), a relative decline in Classopollis spp. (43.6–72.1 %, average 62.4 %) is accompanied by a gradual increase in Inaperturopollenites spp. up to 27.0 % (average 12.0 %) and, less pronounced, in Araucariacites spp. (average 4.5 %). This is followed by a distinct decrease in the relative proportion of Classopollis (as low as 20.5 %) in the middle part (326.5–339.1 m), followed by a gradual increase (up to 78.5 %) within the upper part of the succession (342.1–401.8 m). Corresponding to the Classopollis minimum at 339.1 m, normalized frequencies of Inaperturopollenites spp. and Araucariacites spp. show an opposing pattern with high abundances (up to 31.6 % and 17.7 %, respectively) and display a stepwise decline towards the top of the stratigraphic succession.
6. Discussion
6.a. Chemostratigraphic significance
The overall trend observed in the new composite δ13C record for the BdlC section is in good accordance with existing early Aptian carbon isotope curves and enables identification of the characteristic early Aptian chemostratigraphic segments (Menegatti et al. Reference Menegatti, Weissert, Brown, Tyson, Farrimond, Strasser and Caron1998; Millán et al. Reference Millán, Weissert, Fernandez-Mendiola and Garcia-Mondejar2009; Kuhnt, Holbourn & Moullade, Reference Kuhnt, Holbourn and Moullade2011; Hu et al. Reference Hu, Zhao, Yilmaz and Li2012; Moreno-Bedmar et al. Reference Moreno-Bedmar, Company, Sandoval, Tavera, Bover-Arnal, Salas, Delanoy, Maurrasse and Martinez2012; Gaona-Narvaez, Maurrasse & Moreno-Bedmar, Reference Gaona-Narvaez, Maurrasse and Moreno-Bedmar2013). However, uncertainties exist with regard to the position of the negative carbon isotope peak (segment C3) and the exact position of the OAE 1a interval. In a previous interpretation of the carbon isotope record, a number of negative δ13C values (ranging from −1.4 to −0.1 ‰) have been interpreted to reflect the C3 negative peak located at the base of the OAE 1a (Moreno-Bedmar et al. Reference Moreno-Bedmar, Company, Bover-Arnal, Salas and Delanoy2009; Bover-Arnal et al. Reference Bover-Arnal, Moreno-Bedmar, Salas, Skelton, Bitzer and Gili2010). These comparatively negative carbon isotope values represent samples taken from an interval composed of an indurated Lithocodium-rich limestone unit (305–311 m), whereas the remainder of the carbon isotope dataset is based on marly lithologies. In order to investigate the isotopic composition and variability of this conspicuous limestone unit, petrographic inspection and geochemical analysis of selected components from the Lithocodium-rich limestone unit has been conducted (Fig. 5). Differentiation between various types of matrices, bioclastic components and cements shows a wide range of δ13C signatures with comparatively positive values (up to +3.4 ‰) for biological precipitates (Lithocodium-crust, shell material) to strongly depleted values (as low as −4.2 ‰) for diagenetic cements within a single hand specimen. Analysed bulk limestone samples from this horizon show values between packstone matrix and sparite cement composition, indicating substantial diagenetic alteration of the bulk rock signature. Given the strong heterogeneity of the Lithocodium-rich limestone unit with regard to its stable isotope signature and the indication for diagenetic alteration of its bulk rock signal, we decide to exclude it from the dataset used for our stratigraphic purposes.
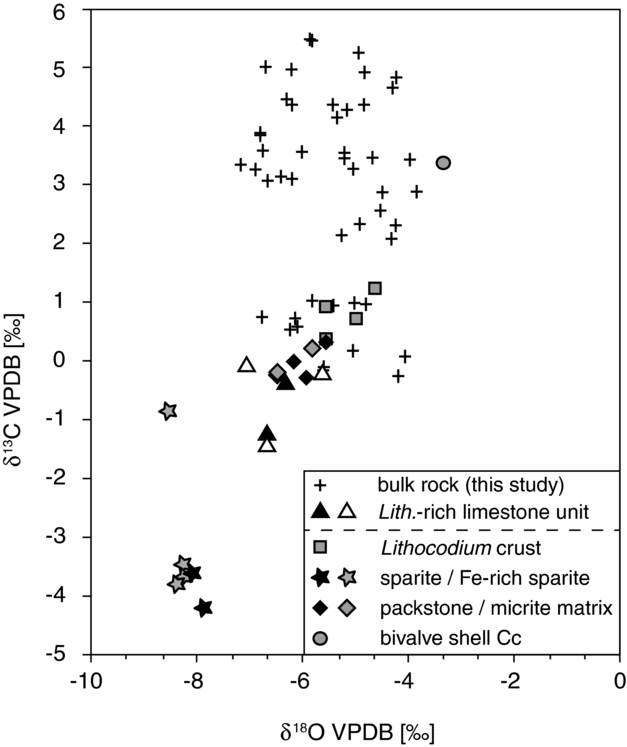
Figure 5. Diagram showing δ13C and δ18O values from the BdlC section plotted against each other. Black crosses represent stable isotope data derived from marly lithologies; triangles correspond to bulk rock measurements from the Lithocodium-rich limestone unit in this study (white) and by Bover-Arnal et al. (Reference Bover-Arnal, Moreno-Bedmar, Salas, Skelton, Bitzer and Gili2010) (black), respectively. Other black and grey symbols correspond to values of different types of carbonate phases from a single hand specimen from the Lithocodium-rich limestone unit.
Based on these observations, some modifications in the previous chemostratigraphic interpretation of the BdlC carbon isotope record are proposed. In the revised curve (excluding the negative values from the Lithocodium-rich limestone unit), the C3 segment is represented by a gradual trend towards minimum values of −0.3 ‰ spanning the interval between 285 m and 296 m (Fig. 2). A stratigraphically more extended C3 peak with less negative values is in good accordance with published δ13C records from the eastern Iberian Chain (Cap de Vinyet section; Moreno-Bedmar et al. Reference Moreno-Bedmar, Company, Bover-Arnal, Salas and Delanoy2009) and the Prebetic domain of Spain (Cau section; Moreno-Bedmar et al. Reference Moreno-Bedmar, Company, Sandoval, Tavera, Bover-Arnal, Salas, Delanoy, Maurrasse and Martinez2012). Furthermore, the revised curve fits well with records from France (La Bedoule section; Moullade et al. Reference Moullade, Kuhnt, Bergen, Masse and Tronchetti1998; Kuhnt, Holbourn & Moullade, Reference Kuhnt, Holbourn and Moullade2011) and Switzerland (Roter Sattel section; Menegatti et al. Reference Menegatti, Weissert, Brown, Tyson, Farrimond, Strasser and Caron1998) as well as with recently published records from Oman (Vahrenkamp, Reference Vahrenkamp, Van Buchem, Al-Husseini, Maurer and Droste2010; Yamamoto et al. Reference Yamamoto, Ishibashi, Takayanagi, Asahara, Sato, Nishi and Iryu2013) and Tunisia (Heldt, Bachmann & Lehmann, Reference Heldt, Bachmann and Lehmann2008). As a consequence of the vertical adjustment of the C3 peak, the subsequent C4 segment now spans an extended interval between 296 and 312 m. The existing chemostratigraphic assignment of the overlying segments C5 to C8 is not modified.
The proposed revision of the chemostratigraphic interpretation of the C3 segment has consequences for the position of the OAE 1a interval in the BdlC section. Based on reference records from Italy and Switzerland (Menegatti et al. Reference Menegatti, Weissert, Brown, Tyson, Farrimond, Strasser and Caron1998; Li et al. Reference Li, Bralower, Montanez, Osleger, Arthur, Bice, Herbert, Erba and Silva2008), the organic carbon-rich OAE 1a horizon is bracketed by the chemostratigraphic segments C3–C6. By applying the similar stratigraphic subdivision, the OAE 1a is represented by the interval between 285 m (base of C3) and 325 m (top of C6) in the BdlC section. Compared to the previous interpretation, the OAE 1a is now significantly expanded downwards into the lower part of the Forcall Formation spanning a total thickness of c. 40 m. Estimates for the duration of the OAE 1a based on orbital chronologies range between 1.0 and 1.3 Ma (Li et al. Reference Li, Bralower, Montanez, Osleger, Arthur, Bice, Herbert, Erba and Silva2008; Malinverno, Erba & Herbert, Reference Malinverno, Erba and Herbert2010) and result in average sedimentation rates of the order 3.1–4.0 cm ka−1 for the OAE 1a episode in the BdlC section.
6.b. Palynofacies and sea level
In order to investigate the potential effect of changing depositional environment and/or sea-level fluctuations on the composition of the spore-pollen assemblage, the particulate sedimentary organic matter has been studied as a whole. Qualitative and quantitative analysis of the palynofacies can provide useful information on changing patterns of organic matter sedimentation, and certain parameters can be used to distinguish transgressive–regressive trends in sedimentary successions (Gorin & Steffen, Reference Gorin and Steffen1991; Rameil, Götz & Feist-Burkhardt, Reference Rameil, Götz and Feist-Burkhardt2000; Schiøler, Crampton & Laird, Reference Schiøler, Crampton and Laird2002; Pellaton & Gorin, Reference Pellaton and Gorin2005). For the Galve sub-basin, two independent and detailed sequence-stratigraphic interpretations based on an analysis of facies and depositional environments exist (Vennin & Aurell, Reference Vennin and Aurell2001; Bover-Arnal et al. Reference Bover-Arnal, Moreno-Bedmar, Salas, Skelton, Bitzer and Gili2010; Embry et al. Reference Embry, Vennin, Buchem, Schroeder, Pierre, Aurell, Van Buchem, Gerdes and Esteban2010). These studies agree in interpreting the Forcall and lower Villarroya de los Pinares formations to represent the second of two major transgressive–regressive sequences which characterize early Aptian time. According to these studies, the transgression is marked by the transition from platform carbonates with mud- and wackestone textures towards basinal deposits, including mainly orbitolinid-rich packstones and marls. The establishment of a highstand carbonate platform with rudist bivalves and colonial corals characterizes the regressive stage (Bover-Arnal et al. Reference Bover-Arnal, Salas, Moreno-Bedmar and Bitzer2009). The general deepening trend proposed for the Forcall Formation is well displayed in the stratigraphic distribution of the particulate organic matter. Despite certain scatter, the trend visible in the CONT/MAR ratio and the dinoflagellate cyst abundance is in good agreement with the existing sequence-stratigraphic interpretations (Vennin & Aurell, Reference Vennin and Aurell2001; Bover-Arnal et al. Reference Bover-Arnal, Moreno-Bedmar, Salas, Skelton, Bitzer and Gili2010; Embry et al. Reference Embry, Vennin, Buchem, Schroeder, Pierre, Aurell, Van Buchem, Gerdes and Esteban2010). Whereas the lower part of the sequence is characterized by a stepwise decrease in continent-derived organic input, the upper part is marked by the strong dominance of marine dinoflagellate cysts and sparse terrestrial debris (Fig. 2).
The pronounced deepening trend visible in the different fractions of the particulate organic matter has apparently no strong effect on the distribution of the sporomorph assemblage. None of the investigated groups shows a pattern that directly correlates with the CONT/MAR ratio or the dinoflagellate percentage. Even sporomorph types with a documented tendency towards taphonomic enrichment during transport and/or deposition, such as bisaccate pollen or thick-walled ornamented spores (Heusser & Balsam, Reference Heusser and Balsam1977; Tyson, Reference Tyson1995; Traverse, Reference Traverse2007), show no distribution pattern that would correspond to the inferred transgressive–regressive trend. Furthermore, the very similar distribution pattern observed in sporomorph groups with very different size and exine thickness (Araucariacites and Inaperturopollenites) is incompatible with strong sorting effects. Hydrodynamic sorting processes associated with sea-level change are therefore considered to be of minor importance in controlling the distribution of spores and pollen in the studied hemipelagic depositional setting. This conclusion is in agreement with the general view that spore-pollen assemblages obtained from open marine settings predominantly reflect changes in vegetation patterns on the adjacent hinterland rather than taphonomic sorting processes during transport and deposition (e.g. Abbink, Reference Abbink1998; van der Kaars, Reference van der Kaars2001; Moss, Kershaw & Grindrod, Reference Moss, Kershaw and Grindrod2005; Traverse, Reference Traverse2007; Schrank, Reference Schrank2010; Dupont, Reference Dupont2011).
6.c. Changes in terrestrial vegetation
The distribution patterns of the palynoflora show distinct, stepwise changes within the stratigraphic succession (Fig. 4), interpreted to reflect changes in the terrestrial ecosystems of the nearby Iberian Massif and surrounding islands. Before and during the onset of the OAE 1a (upper C2 and C3 segments) the vegetation is strongly dominated by drought-resistant (xerophytic) Cheirolepidiaceae, most probably thriving under hot and semi-arid to arid conditions (Doyle, Jardiné & Doerenkamp, Reference Doyle, Jardiné and Doerenkamp1982; Vakhrameyev, Reference Vakhrameyev1982, Reference Vakhrameyev1991; Francis, Reference Francis1983; Heimhofer et al. Reference Heimhofer, Adatte, Hochuli, Burla and Weissert2008; Schrank, Reference Schrank2010; Bonis & Kürschner, Reference Bonis and Kürschner2012; Riding et al. Reference Riding, Leng, Kender, Hesselbo and Feist-Burkhardt2013). A first drop in the abundance of cheirolepideacean conifers during the C4 interval is paralleled by increasing abundances of Araucariaceae-derived pollen types, which are interpreted to reflect a shift of the forest ecosystem towards warm, mesic habitats with a moderate supply of moisture (Doyle, Jardiné & Doerenkamp, Reference Doyle, Jardiné and Doerenkamp1982; Abbink, Van Konijnenburg – Van Cittert & Visscher, Reference Abbink, Van Konijnenburg – Van Cittert and Visscher2004; Schrank, Reference Schrank2010). A similar change from semi-arid to more humid conditions at the onset of the C4 interval is indicated by a relative increase in kaolinite to the detriment of smectite, mica and chlorite contents. A second, even more pronounced decline in Cheirolepidiaceae begins with the end of segment C6, the horizon, which corresponds to the end of the OAE 1a interval. Cheirolepidiaceans show an abrupt reduction here, paralleled by a distinct increase in the relative abundance of Araucariaceae-type conifers. Given the varying ecological requirements of the different conifer types, the observed turnover in the plant ecosystem was most probably driven by a change in climate towards less arid and cooler conditions. A similar shift in the K/(M + C) ratio can be interpreted to reflect a change in weathering patterns towards more cooler conditions. Interestingly, trilete spores, which are predominantly produced by leptosporangiate ferns, show no significant changes with stratigraphic height in either terms of frequency abundance or diversity. Given the generally hygrophilous character of many late Mesozoic ferns (e.g. van Konijnenburg – van Cittert, Reference van Konijnenburg – van Cittert2002), the stable distribution patterns and low abundances indicate that conditions remained comparatively dry even during the interval characterized by high Araucariaceae abundances. Within segment C7, the re-establishment of cheirolepidiacean forests is indicated by a successive increase in Classopollis pollen and declining abundances in Araucariacites and Inaperturopollenites. This interpretation is supported by increasing smectite contents paralleled by low kaolinite and decreasing mica contents, indicating a return to more semi-arid climate conditions. However, this latter shift coincides with a distinct increase in dinoflagellate cyst abundance, interpreted to reflect a sea-level rise. Since smectite is usually more abundant in open marine settings, the high smectite ratios may also reflect (at least in partly) a sea-level rise (Adatte, Keller & Stinnesbeck, Reference Adatte, Keller and Stinnesbeck2002).
Comparison with existing terrestrial spore-pollen records from time-equivalent deposits in other Tethyan basins provides further insights into vegetation dynamics during and after OAE 1a (Fig. 6). The strong dominance of thermophilous cheirolepidiacean conifers in the initial phase of the OAE 1a is not restricted to the Maestrat Basin record, but can also be observed at other localities. High Classopollis abundances (36.4–84.9 %) have been reported by Najarro et al. (Reference Najarro, Rosales, Moreno-Bedmar, de Gea, Barron, Company and Delanoy2011) from the C3 segment of a succession located in the North Cantabrian Basin (Spain). In the study of Keller et al. (Reference Keller, Hochuli, Weissert, Bernasconi, Giorgioni and Garcia2011) based on marine records from the Lombardian and Belluno basins (Italy), the C3 interval (sensu Menegatti et al. Reference Menegatti, Weissert, Brown, Tyson, Farrimond, Strasser and Caron1998) is characterized by a gradual increase in Classopollis with peak values reaching up to 79.3 % within the lower segment C4. The upper part of C4 as well as segments C5 and C6 are marked by moderately high but fluctuating Classopollis contents, followed by a significant decline subsequent to the termination of the black shale episode (Fig. 6). In the Belluno Basin, the decrease in Cheirolepidiaceaen pollen is paralleled by a dramatic post-OAE rise in bisaccate conifer pollen interpreted to reflect a major cooling episode (Hochuli et al. Reference Hochuli, Menegatti, Weissert, Riva, Erba and Premoli Silva1999). High bisaccate abundances are also reported by Najarro et al. (Reference Najarro, Rosales, Moreno-Bedmar, de Gea, Barron, Company and Delanoy2011) from a single sample attributed to segment C8 (upper Aptian). The spore-pollen distribution patterns observed in the Belluno and Maestrat basins differ markedly from the rather stable Classopollis and Araucariacites pollen contents observed by Heimhofer et al. (Reference Heimhofer, Hochuli, Herrle, Andersen and Weissert2004) in the Vocontian Basin of SE France. Here, a record covering the upper part of the OAE 1a interval has been studied. The absence of distinct variations in the spore-pollen assemblages led to the conclusion that the OAE 1a itself had no major impact on the vegetation composition surrounding the Vocontian Basin (Heimhofer et al. Reference Heimhofer, Hochuli, Herrle, Andersen and Weissert2004). However, given the uncertainties in chemostratigraphic correlations across different basins, the Vocontian dataset may actually cover only the C5–C6 segments. Hence, neither the prominent fluctuations in palynoflora at the onset of the OAE 1a (segments C3–C4) nor the major turnover following its termination (C7) seem to be represented in that record.

Figure 6. Comparison of relative abundances (%) of selected gymnosperm pollen types (including Classopollis, Araucariacites and bisaccate pollen) from various Tethyan sites during the early Aptian OAE 1a. Pollen data from the Lombardian Basin are derived from Keller et al. (Reference Keller, Hochuli, Weissert, Bernasconi, Giorgioni and Garcia2011), from the Belluno Basin from Hochuli et al. (Reference Hochuli, Menegatti, Weissert, Riva, Erba and Premoli Silva1999) and from the Vocontian Basin from Heimhofer et al. (Reference Heimhofer, Hochuli, Herrle and Weissert2006).
Regarding the distribution pattern of Classopollis pollen, the records from Italy (Belluno and Lombardian basins) and Spain (Maestrat Basin) show very similar distribution patterns across the OAE 1a interval with high Classopollis contents prevailing within the C3 and lower C4 segments, followed by a moderate stepwise decline during segments C5–C6. Lowest Classopollis contents are observed in the aftermath of the OAE 1a within segment C7. The main floral turnover is observed at this stratigraphic level, which is characterized by a significant increase in bisaccate conifer pollen in the Belluno Basin (Hochuli et al. Reference Hochuli, Menegatti, Weissert, Riva, Erba and Premoli Silva1999) and by a rise in non-saccate pollen types of auraucarian affinity (including Araucariacites, Inaperturopollenites) in the Maestrat Basin. These differences in palynofloral composition are best explained by latitudinal effects due to differing positions of the respective sites within the mid-latitude warm humid belt.
6.d. Terrestrial ecosystem change in response to OAE 1a
Increased volcanogenic carbon dioxide and other greenhouse gas emissions, in concert with intermittent methane injections from submarine hydrate dissociation, have been proposed to have altered the chemistry of the early Aptian atmosphere and oceans (Jahren et al. Reference Jahren, Arens, Sarmiento, Guerrero and Amundson2001; Weissert & Erba, Reference Weissert and Erba2004; Méhay et al. Reference Méhay, Keller, Bernasconi, Weissert, Erba, Bottini and Hochuli2009; Erba et al. Reference Erba, Bottini, Weissert and Keller2010; Bottini et al. Reference Bottini, Cohen, Erba, Jenkyns and Coe2012). Excess carbon dioxide levels resulted in exceptional climate warming as indicated by different types of sea-surface temperature (SST) reconstructions (Schouten et al. Reference Schouten, Hopmans, Forster, van Breugel, Kuypers and Damsté2003; Dumitrescu et al. Reference Dumitrescu, Brassel, Schouten, Hopmans and Sinninghe Damsté2006; Ando et al. Reference Ando, Kaiho, Kawahata and Kakegawa2008; Jenkyns et al. Reference Jenkyns, Schouten-Huibers, Schouten and Sinninghe Damsté2012).
A phase of warmth at the onset of the OAE 1a is in line with the palynological evidence from the Maestrat and Belluno basins. The high abundances of Classopollis pollen at both sites during the early phase of the event supports a scenario with very warm to hot temperatures triggered by rapid volcanogenic CO2 injection, probably in conjunction with methane hydrate dissociation (Méhay et al. Reference Méhay, Keller, Bernasconi, Weissert, Erba, Bottini and Hochuli2009). These unusual climatic conditions may have caused the proliferation of draught-adapted Cheirolepidiacean forests in the studied lower mid-latitude settings. Given the transitional position of the Iberian Massif between two climatic zones, the northwards expansion of the so-called tropical-equatorial hot-arid belt could explain the spread of this thermophile plant type during an exceptional hothouse episode at the onset of OAE 1a (Keller et al. Reference Keller, Hochuli, Weissert, Bernasconi, Giorgioni and Garcia2011).
The initial phase of extraordinary warmth was replaced by a phase of relative climatic cooling, which has been associated with the effect of CO2 drawdown due to enhanced organic carbon burial (Dumitrescu et al. Reference Dumitrescu, Brassel, Schouten, Hopmans and Sinninghe Damsté2006; Ando et al. Reference Ando, Kaiho, Kawahata and Kakegawa2008; Kuhnt, Holbourn & Moullade, Reference Kuhnt, Holbourn and Moullade2011) and/or reduced volcanogenic activity (Keller et al. Reference Keller, Hochuli, Weissert, Bernasconi, Giorgioni and Garcia2011). Based on TEX86 data from the tropical Pacific, Dumitrescu et al. (Reference Dumitrescu, Brassel, Schouten, Hopmans and Sinninghe Damsté2006) reported instabilities in SSTs with two episodes of transient cooling (up to 4 °C) during the OAE 1a black shale interval. Similar transient phases of SST decline have been reported from the Cenomanian–Turonian OAE 2 and linked to the effect of excess carbon sequestration (Sinninghe Damste et al. Reference Sinninghe Damste, van Bentum, Reichart, Pross and Schouten2010). For the OAE 1a, the intermittent effect of carbon burial (as illustrated in the widespread deposition of organic carbon-rich facies) is considered to have caused short-lasting fluctuations in temperature and humidity in the NW Tethyan realm. This is indicated by the fluctuating, but overall declining abundance trend observed in Cheirolepidiaceans during segments C5 and C6 in the Belluno and Maestrat basins.
With the end of segment C6 (which corresponds to the second positive C-isotope shift), marine black shale deposition ceased worldwide (Menegatti et al. Reference Menegatti, Weissert, Brown, Tyson, Farrimond, Strasser and Caron1998; Bellanca et al. Reference Bellanca, Erba, Neri, Premoli, Sprovieri, Tremolada and Verga2002; Price, Reference Price2003; Herrle et al. Reference Herrle, Kössler, Friedrich, Erlenkeuser and Hemleben2004; Robinson et al. Reference Robinson, Clarke, Nederbragt and Wood2008; Gaona-Narvaez, Maurrasse & Moreno-Bedmar, Reference Gaona-Narvaez, Maurrasse and Moreno-Bedmar2013). In contrast, the changes affecting terrestrial ecosystems proceed in the aftermath of the OAE 1a. In the Maestrat Basin, Classopollis pollen reaches its low point (which corresponds to a maximum in Araucaria-derived pollen) about 14.5 m above the end of segment C6. Similarly, a pronounced decline in Classopollis occurs just above the uppermost organic-rich layer in the Belluno Basin and is paralleled by a major increase in bisaccates (Hochuli et al. Reference Hochuli, Menegatti, Weissert, Riva, Erba and Premoli Silva1999). Thus, significant changes in terrestrial vegetation post-date the black shale episode and therefore remain difficult to explain with climatic cooling triggered exclusively by enhanced marine organic carbon accumulation. As noted already by Bralower et al. (Reference Bralower, Arthur, Leckie, Sliter, Allard and Schlanger1994), the major positive carbon isotope excursion occurs during the late stages of the OAE 1a. In order to explain the temporal lag between marine black shale formation and the subsequent positive carbon isotope shift, burial of organic carbon in marginal marine or continental settings has been suggested as one possibility (Bralower et al. Reference Bralower, Arthur, Leckie, Sliter, Allard and Schlanger1994). A similar scenario has recently been proposed to explain the Valanginian positive carbon isotope anomaly, which also lacks time-equivalent marine black shale deposits (Westermann et al. Reference Westermann, Föllmi, Adatte, Matera, Schnyder, Fleitmann, Fiet, Ploch and Duchamp-Alphonse2010; Kujau et al. Reference Kujau, Heimhofer, Ostertag-Henning, Greselle and Mutterlose2012;). For the early Aptian period, a change from predominantly marine to terrestrial organic carbon burial would be in line with the existing palynological evidence. Climatic cooling triggered initially by marine black shale formation may have resulted in an equator-wards expansion of the mid- and high-latitude warm and temperate humid belts, thus expanding the area of coal formation during Early Cretaceous time (McCabe & Parrish, Reference McCabe, Parrish, McCabe and Parrish1992). Coals and lignites dated as Aptian have been described from various localities including Artic Canada and the Canadian Rocky Mountains (Bustin & Smith, Reference Bustin and Smith1993; Basset & Kleinspehn, Reference Basset and Kleinspehn1997), NE China (Sha et al. Reference Sha, Hiromichi, Yao and Pan2008), Mongolia (Erdenetsogt et al. Reference Erdenetsogt, Lee, Bat-Erdene and Jargal2009) and SE Russia (Kirillova, Reference Kirillova2003). Similarly, the formation and expansion of lacustrine settings often associated with high organic carbon accumulation rates (e.g. Mello and Maxwell, Reference Mello, Maxwell and Katz1990; Ntamak-Nida et al. Reference Ntamak-Nida, Baudin, Schnyder, Makong, Komguem and Abolo2008; Lirong et al. Reference Lirong, Dingsheng, Zhi, Zhiwei and Jingchun2013) may have resulted from the expansion of humid zones during early Aptian time. A protracted phase of terrestrial carbon burial in the aftermath of the OAE 1a would also explain the long-lasting interval characterized by positive carbon isotope values during late early Aptian time.
7. Conclusions
The normalized frequencies of spore-pollen and clay mineral assemblages derived from the BdlC section, Maestrat Basin, east Spain, show distinct variations with stratigraphic height throughout the early Aptian Forcall Formation, which are paralleled by distinct and well-established changes in the δ13C trend before, during and in the aftermath of the OAE 1a interval. Based on comparison with existing interpretations on the evolution of the depositional system and corroborated by new palynofacies data, hydrodynamic sorting due to sea-level changes is not considered as the first-order control on the distribution patterns of the spore-pollen and clay mineral assemblage. The distribution patterns of climate-sensitive conifer pollen support a phase of warmth during the OAE 1a itself. This warm phase is followed by a subsequent cooling, as indicated by significant changes in the gymnosperm pollen assemblage and weathering products. The cooling episode post-dates the OAE 1a interval but coincides with a major positive δ13C shift, which is interpreted to reflect enhanced OC burial in the marine realm or on the continents.
Acknowledgements
We thank Ramon Salas (University of Barcelona) and Josep Anton Moreno-Bedmar (UNAM) for their support and help with sampling in the western Maestrat Basin. Christiane Wenske (Leibniz University Hannover) is acknowledged for support with the stable isotope measurements. Financial support from the Nordrhein-Westfälische Akademie der Wissenschaften und Künste to UH and by the I + D + i research project CGL2008-04916 to TBA is gratefully acknowledged.
Declaration of interest
None
Supplementary material
To view supplementary material for this article, please visit http://dx.doi.org/10.1017/S0016756814000557