Introduction
Breast cancer has recently overtaken lung cancer as the most commonly diagnosed cancer worldwide with >680 000 deaths reported in 2020 (Ref. Reference Sung1). Metastatic spread to other organs is the main cause of death from breast cancer and around 70–80% of metastases will develop in bone. Bone metastases generally form in bones with a high marrow content such as the ribs, spine or the long bones. This condition has a long latency, and is not usually detected until several years after the patient has completed treatment for their primary disease. The first indication of tumour relapse in bone is often the result of a patient presenting in the clinic with tumour-associated skeletal-related events including pathological fractures and spinal cord compression accompanied by severe pain and hypercalcaemia (Ref. Reference Yang2). At this stage, the disease is currently considered incurable, therapeutic strategy switches to, predominantly, palliative care to reduce pain and increase the quality of life and survival drops to ~2–3 years after initial diagnosis of bone involvement (Ref. Reference Coleman3). Therefore, there is an urgent need to identify biomarkers and targetable drivers of breast cancer bone metastasis. This would enable identification of patients whose tumours are likely to relapse in bone and allow earlier treatment delivery and development of more effective therapeutic strategies.
Interleukin-1β (IL-1β) as a biomarker and potential target for inhibiting/treating breast cancer bone metastasis
High levels of IL-1 in tumours are a predictor of poor prognosis in several cancer types including lung, breast, melanoma and metastatic prostate (Refs Reference Pérez-Ramírez4–Reference Tengesdal7). The IL-1 family is an 11-member group of endogenous cytokines involved in inflammation and immune response, including cytokine activators: IL-1 alpha (IL-1α), IL-1 beta (IL-1β) and the cytokine antagonist, IL-1 receptor antagonist (IL-1Ra). Through binding the type 1 receptor (IL-1R1), IL-1α or IL-1β induces downstream signalling cascades and the transcription of downstream genes (Fig. 1) (Ref. Reference Gelfo8). Both IL-1α and its precursor (pro-IL-1α) are biologically active IL-1R1 ligands, but only mature IL-1β has biological activity after its precursor (pro-IL-1β) is cleaved by caspase-1 (Refs Reference Di Paolo and Shayakhmetov9, Reference Rébé and Ghiringhelli10). In primary human breast cancers, in addition to IL-1β produced by tumour cells, the main cellular sources of IL-1β are myeloid cells, especially monocytes and dendritic cells (DCs), as well as endothelial cells. When tumours are growing in the bone microenvironment, osteoblasts and bone marrow cells are also stimulated to secrete IL-1β. Several of these cell types, including tumour cells, do not just secrete IL-1β they also express IL-1R1 allowing both, autocrine and paracrine IL-1 signalling to exert pro/anti-tumour effects dependent on the makeup of the local environment (Refs Reference Kiss11, Reference Wu12). Through interactions with different cell types, IL-1β participates in several physiological and pathological processes including proliferation, migration, invasion and apoptosis and appears to have organ-specific effects on tumour immune responses to promote pro- or anti-tumour responses (Refs Reference Tulotta5, Reference Tulotta13).

Fig. 1. IL-1 pathway. IL-1β is produced as inactive proform in response to pro-inflammatory signals. Activation of pro-IL-1β is initiated through cleavage by caspase-1 to 17 kD active IL-1β. IL-1β signals by assembling a trimeric receptor complex with IL-1R1 and IL-1RacP. This trimeric complex rapidly recruits, assembles and further modifies Mdy88, IRAKs and TRAF6 which initiates downstream NF-κB-mediated transcription, subsequent cytokine production and pathways that regulate tumourigenesis.
IL-1β expression in primary breast cancers has been shown to correlate with breast cancer stage: ex vivo analysis of primary samples in the laboratory demonstrated that secretion of IL-1α, IL-1β and IL-1Ra was significantly higher in tumour tissues compared with non-cancerous tissue and IL-1β was significantly higher in stage II or stages III–IV breast cancer compared with stages 0–I (Ref. Reference Wu12). In addition, TCGA and METABRIC datasets both show that IL-1B gene expression levels are significantly higher in triple negative breast cancer compared with other subtypes of breast cancer (Ref. Reference Lappano14). Moreover, a 10-year follow-up analysis of IL-1B expression in primary tumour samples taken from 1189 patients with stage II/III enrolled onto the phase III AZURE trial (ISRCTN79831382) before randomisation and commencement of treatment (randomised (1:1) to standard adjuvant therapy alone (control) or with zoledronic acid (zoledronate)) showed a strong correlation between IL-1β in tumour cells and distant recurrence at any site (P = 0.0016) (Ref. Reference Tulotta5). Furthermore, patients with higher IL-1β in their primary tumours were more likely to develop bone metastasis (P = 0.02 and P < 0.0001 from independent studies) (Refs Reference Tulotta5, Reference Nutter15). Interestingly, data from patient samples from the AZURE study showed no correlation between IL-1β expression and ER/HER2 (oestrogen/human epidermal growth factor receptor 2) status, suggesting that IL-1β may be an indicator of metastatic potential independent of breast cancer type. Interestingly, in a separate study focused on ER −ve breast cancer, release of high levels of IL-1α and IL-1β in serum released by circulating tumour cells (CTCs) was associated with an increased risk of death (Ref. Reference Vilsmaier16). Taken together, these data indicate that tumour-derived IL-1β is directly correlated with the breast cancer stage and is an indicator of future metastasis.
Direct evidence that tumour-derived IL-1β can promote tumour cell spread to bone and drive metastatic outgrowth in this environment has come from mouse models: human triple negative MDA-MB-231 breast cancer cells that have been manipulated to specifically home to the bone microenvironment following intra-venous injection in BALB/c nude mice, express high levels of IL-1B (Ref. Reference Nutter15). Furthermore, in humanised mouse models, expression of this cytokine is upregulated by 192 ± 82% in MDA-MB-231 and 500 ± 253% in ER +ve MCF7 cells that spontaneously metastasise from the mammary fat pad to human bone implants compared with cells that did not metastasise (Ref. Reference Lefley17). Importantly, overexpression of IL-1B in breast cancer cells by genetic manipulation significantly increases the number of mice that develop bone metastases following intra-venous injection from 12% in mice injected with MDA-MB-231 control cells to 75% in mice injected with MDA-MB-231-IL-1β+ cells. Overall, these data suggest that IL-1β produced by the tumour cells plays a pivotal role in the homing of these cells to the bone environment (Ref. Reference Tulotta5). Although IL-1β produced by tumour cells appears to promote breast cancer relocation to bone, once in bone the process of metastatic outgrowth appears to be driven by a combination of IL-1β produced by tumour cells and cells from the local environment: arrival of tumour cells in bone leads to an increase in IL-1β secretion by tumour cells, osteoblasts, endothelial cells and components of the bone marrow which, in turn, stimulates IL-1β driven Wnt signalling in cancer stem cells (CSCs) and subsequent development of overt metastases (Refs Reference Tulotta5, Reference Eyre18). The importance of IL-1β from the bone microenvironment on metastatic outgrowth is evidenced in mouse models that are globally deficient in IL-1β (IL-1β−/−). Intra-venous injection of syngeneic E0771 cells into IL-1β−/− mice impaired the development of bone metastasis to 22.2% in IL-1β-deficient (IL-1β−/−) animals compared with 66.7% in control IL-1βfl/fl mice (Ref. Reference Tulotta13). Furthermore, pharmacological inhibition of IL-1 by the IL-1Ra, anakinra, or the human-specific anti-IL-1β antibody, canakinumab, has been shown to almost eliminate the development of bone metastases in various types of mouse models including, syngeneic (anakinra only), ER +ve and ER −ve human tumour-specific, as well as ER +ve and ER −ve models of spontaneous metastasis of human-derived breast cancer cell lines to human bone implants (Refs Reference Tulotta5, Reference Lefley17–Reference Holen19).
Despite being an indicator of poor prognosis in breast cancer and promoting metastatic outgrowth in bone, IL-1β appears to have opposing effects on the primary tumour. Conflicting studies show that IL-1β either promotes or reduces growth of primary tumours and/or metastatic outgrowth of tumours in the lung dependent on the mouse's immunological background and the tumour stage when anti-IL-1 therapies were administered (Refs Reference Tulotta5, Reference Tulotta13, Reference Holen19–Reference Guo21). The majority of studies indicate that IL-1β from the microenvironment is responsible for suppressing tumour growth at the primary site, through stimulating an anti-tumour immune response (Refs Reference Tulotta13, Reference Van Den Eeckhout22), whereas IL-1β produced by tumour cells causes these cells to undergo epithelial to mesenchymal transition (EMT) and spread to other organs (Refs Reference Tulotta5, Reference Li23, Reference Castaño24). Therefore, IL-1β in soft tissues has both pro- and anti-tumour effects and inhibition of this cytokine decreases EMT preventing tumour cells from metastasising, but supports an epithelial phenotype to favour tumour proliferation while also reducing infiltration of innate immune cells, potentially, preventing anti-tumour immunological responses.
In this review, we discuss the effects of IL-1β on primary breast cancer and bone metastasis to assess its potential as therapeutic target to prevent the onset and growth of bone metastases.
IL-1β in the metastatic cascade of breast cancer
Breast cancer bone metastasis is a complex multi-step process: tumour cells escape from mammary gland to the blood, survive in the circulation, home to the bones and eventually form metastatic tumours in the bones (Fig. 2). These tumour cells entering the circulation or entering distant sites are called circulating tumour cells (CTCs) or disseminated tumour cells (DTCs), respectively (Refs Reference Riggio25, Reference Hüsemann26).
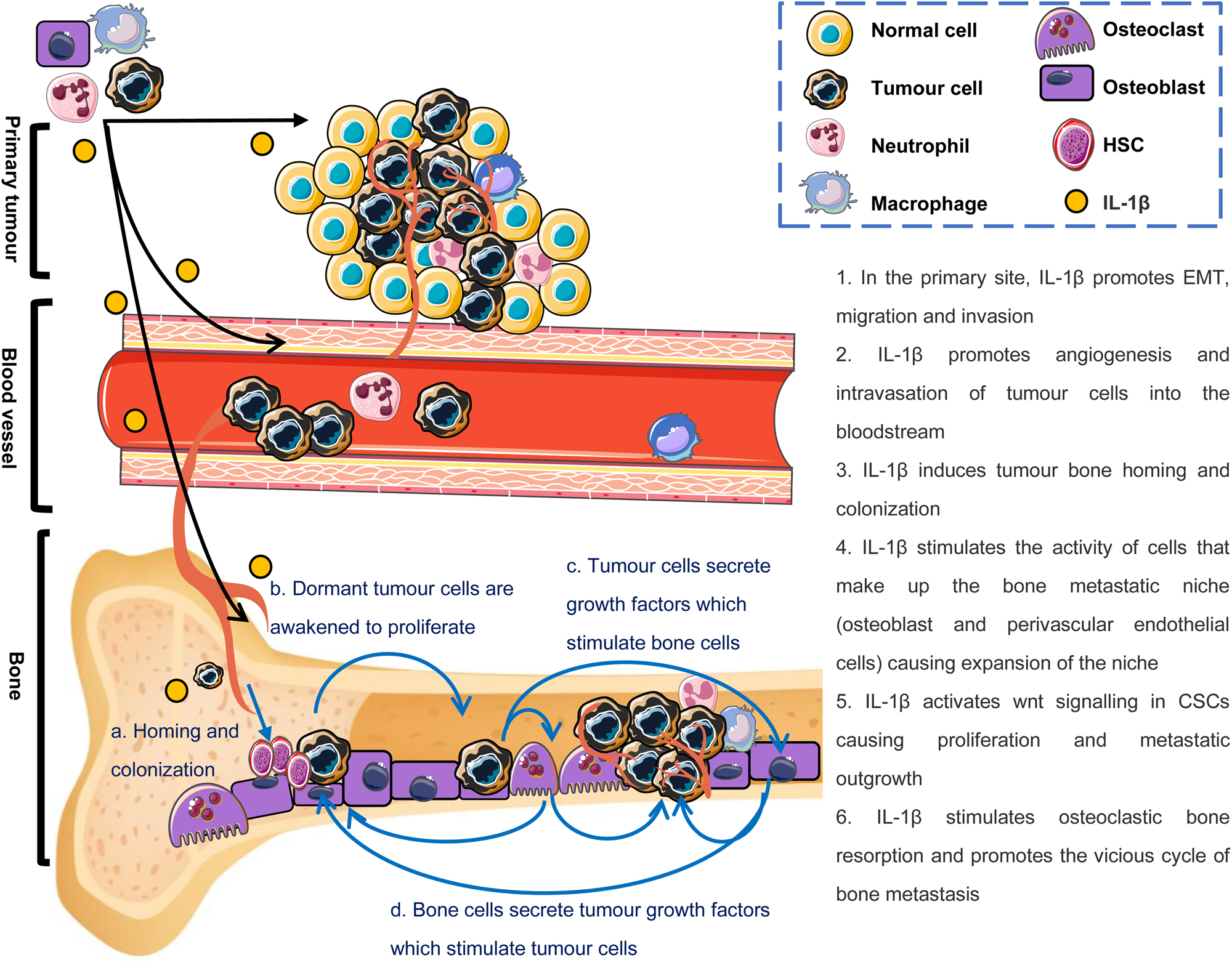
Fig. 2. Effects of IL-1β on the breast cancer bone metastatic cascade and ‘vicious cycle of bone metastases’. Black arrows indicate the breast cancer bone metastatic cascade: tumour cells escape from mammary gland to the blood, survive in the circulation, home to the bones and eventually form metastatic tumours in the bones. Blue arrows indicate how IL-1β in the bone microenvironment drives the ‘vicious cycle of bone metastases’.
Genes associated with IL-1B alteration in breast cancer bone metastasis
The initial stage of the metastatic cascade requires tumour cells to escape from the primary site (Ref. Reference Sosa27). In order to do this, primary tumour cells acquire specific genetic and epigenetic alterations that enable them to invade surrounding tissue, degrade the basement membrane of the vasculature and detach from the primary tumour (Ref. Reference Sosa27). Using humanised mouse models of human breast cancer metastasis to human bone implants, Tulotta et al. identified a significant increase in IL-1B gene expression in CTCs and metastatic tumours in the bone compared with primary tumours in the fat pads (Ref. Reference Tulotta5). In addition, various genes associated with EMT (E-cadherin, N-cadherin and JUP) were also significantly changed in these metastases compared with primary tumours (Ref. Reference Tulotta5). This association with upregulated IL-1B and genes associated with EMT and development of breast cancer metastasis, is not unique to the humanised model, and it has also been reported in spontaneous tumour development and metastasis (MMTV-Py8119 model), as well as in bone homing human MDA-MB-231 cells (Refs Reference Nutter15, Reference Guo21).
IL-1β and EMT, migration and invasion in breast cancer
EMT enables tumour cells to acquire a more invasive phenotype (Ref. Reference Sosa27). Tumour cells undergoing EMT lose their gap junctions and apical-basal polarity and increase their motility to enable enhanced migratory and invasive phenotype (Ref. Reference Lamouille28). The majority of evidence that this process can be driven by IL-1β has come from in vitro experiments.
Genetic manipulation of ER +ve and ER −ve breast cancer cell lines to overexpress IL-1β (MDA-MB-231-IL-1β+, T47D-IL-1β+ and MCF7-IL-1β+) results in both genotypic and phenotypic alterations associated with EMT. IL-1β overexpressing cells expressed significantly less E-cadherin and γ-catenin and more N-cadherin, at both gene and protein levels, compared with the relevant wild-type controls (Ref. Reference Tulotta5). Meanwhile, IL-1β overexpressing cells from this as well as other studies have demonstrated more aggressive invasion and migration abilities in scratch and transwell invasion experiments, in vitro, compared with wild-type control cells (Refs Reference Tulotta5, Reference Tulotta13, Reference Najafi29). Additionally, studies have indicated that IL-1β induced triple-negative breast cancer cells to produce IL-6, and increase tumour proliferation, EMT and drug resistance through the IL-6/JAK2/STAT3 pathway (Ref. Reference Oh30). Moreover, EMT and stem cell-like phenotypes of tumour cells had been attenuated by administration of anti-IL-6 or anti-IL-1β antibodies (Refs Reference Tulotta5, Reference Oh30).
Matrix metalloproteinases (MMPs) are a group of enzymes that degrade the extracellular matrix (ECM) (Ref. Reference Najafi29). A recent study has showed that administration of exogenous IL-1β promoted MMP-9 production in non-metastatic MCF-7 breast cancer cells leading to acquisition of invasive ability by activating focal adhesion kinase and proto-oncogene tyrosine-protein kinase Src (Src) (Ref. Reference Mon31). Moreover, exogenous IL-1β has been shown to induce MMP-2 to degrade the ECM or hard mineralised matrix of bones by increasing β-catenin translocation (Ref. Reference Perez-Yepez32). In addition to effects of exogenous IL-1β, IL-1β produced by MDA-MB-231 breast cancer cells under hypoxia also promoted an invasive phenotype in cancer-associated fibroblasts (CAFs) by paracrine feed-forward signalling (Ref. Reference Lappano14). This cross-talk between CAFs with cancer cells and tumour microenvironment (TME) are also pivotal for the degradation of the ECM (Ref. Reference Najafi29). Importantly, these invasive cancer phenotypes triggered by IL-1β/IL-1R signalling were reversed after IL-1 inhibition with the IL-1R1 antagonist anakinra (Refs Reference Tulotta5, Reference Eyre18, Reference Voloshin20). These data suggest that EMT induced by IL-1β is one of the main reasons that IL-1β drives the early stages of breast cancer metastasis.
IL-1β and angiogenesis in breast cancer
Angiogenesis is a fundamental marker of malignant tumours and metastatic tumours. Tumour cells and cells that make up the TME, including endothelial cells, mesenchymal cells and immune cells, can secrete a variety of cytokines that promote angiogenesis. IL-1β/IL-1R signalling has been shown to mediate angiogenesis by indirectly inducing vascular endothelial growth factor (VEGF) expression and secretion in various types of cells in a multitude of tumours including breast cancer (Ref. Reference Fahey and Doyle33) and it has been hypothesised that IL-1β synergises with VEGF in the early angiogenic response (Ref. Reference Voronov34). Administration of anakinra significantly decreased the percentage of Ki67 positive cells and the number of CD31, CD34 positive micro-vessels in subcutaneous breast cancer tumours, but had no effect on the percentage of caspase-3 positive cells (Refs Reference Holen19, Reference Voloshin20). This suggests that inhibition of IL-1β/IL-1R1 signalling can also show an anti-tumour effect by inhibiting angiogenesis.
IL-1β and breast cancer bone homing and colonisation
The next step of the metastatic cascade is accumulation of DTCs in secondary metastatic organs and lodging of tumour cells in the pre-metastatic niches that support the dormancy and/or outgrowth of DTCs (Ref. Reference Sosa27). Several breast cancer cells have a high affinity for bone in particular areas that make up the metastatic niche(s): the endosteal niche (consisting of osteoblasts and haematopoietic stem cells) and the peri-vascular niche (Refs Reference Eyre18, Reference Brown35, Reference Ottewell36). Nutter et al. have established breast cancer bone homing cells (MDA-IV) by repeatedly passing MDA-MB-231 cells, in vivo, and found that IL1B gene expression and IL-1β secretion were significantly increased in MDA-IV compared with parental MDA-MB-231 cells (Ref. Reference Nutter15). Furthermore, MDA-IV cells have been shown to home to the peri-vascular, endosteal and haematopoietic stem cell niches in mouse bone (Ref. Reference Allocca37) which suggests that IL-1β is associated with breast cancer bone homing (Ref. Reference Nutter15). In support of this hypothesis, injecting MDA-MB-231 breast cancer cells that over express IL-1β into the tail vein of BALB/c nude mice significantly increases the number of mice that develop bone metastasis (as discussed previously) (Refs Reference Tulotta5, Reference Holen19). It is widely accepted that the CXCL12–CXCR4 axis plays a pivotal role in the progression and metastasis of breast cancer: stromal cell-derived CXCL12 is expressed in secondary metastatic sites including by osteoblasts, haematopoietic stem cells and endothelial cell that make up the bone metastatic niche. It is believed that CXCL12 attracts metastatic tumour cells that with upregulated CXCR4 receptor (Ref. Reference Wang38). Experiments performed by Valdivia-Silva et al. indicated that IL-1β stimulation increases the expression of CXCR4 on MCF-7 cells which, in turn, displayed a higher migration index to CXCL12 (Ref. Reference Valdivia-Silva39). Moreover, MDA-MB-231-derived IL-1β was shown to activate mesenchymal stem cells and induce various chemokines including CXCL12 to facilitate trans-endothelial migration of breast cancer cells to the bone marrow (Ref. Reference Escobar40). These data suggest that IL-1β promotes interaction between CXCR4 and CXCL12 and thus induce breast cancer bone homing.
Dissemination of breast cancer cells into the bone marrow is an early event, likely to occur before clinical detection of the primary disease (Ref. Reference Hüsemann26). Studies have shown that 30–40% of breast cancer patients have DTCs in their bone marrow, and for most patients these cells will remain dormant for long periods (several months to years) prior to proliferating into overt metastases (Refs Reference Klein41–Reference Braun43). Therefore, the long-term survival of DTCs, in bone, is a risk factor for recurrence of breast cancer several years after the removal of the primary breast tumour (Refs Reference Riggio25, Reference Tjensvoll44). After a phase of dormancy, components of the metastatic niche can re-activate DTCs. Although the whole mechanism of how this happens is yet to be identified, strong evidence suggests that bone-derived transforming growth factor-beta (TGF-β1) and IL-1β are able to reactivate dormant DTCs stimulating metastatic outgrowth (Refs Reference Jahanban-Esfahlan45, Reference Tulotta and Ottewell46). There is evidence that when these activated DTCs undergo EMT, they acquire stem cell-like properties, thus becoming CSCs to finally enable the formation of overt metastases (Refs Reference Valdivia-Silva39, Reference Lawson47, Reference Balic48). Eyre et al. found that bone marrow-derived IL-1β enables breast CSCs to form metastatic colonies in the bone via activation of intracellular NF-κB/CREB signalling and Wnt ligand production by cancer cells, and neutralisation of IL-1β inhibited the induction of colony formation (Ref. Reference Eyre18). In addition, Sarmiento-Castro et al. found that IL-1β/IL-1R1 signalling is likely to be important for CSCs to drive ALDH+ activity of anti-oestrogen-resistant in breast cancer (Ref. Reference Sarmiento-Castro49). These data suggest that targeting IL-1β signalling can prevent breast cancer bone metastasis by impairing both bone homing of CSCs and colony formation in the bone environment.
IL-1β and vicious cycle of breast cancer bone metastasis
As previously described, bone cell-derived IL-1β enables reactivation of dormant DTCs (Refs Reference Jahanban-Esfahlan45, Reference Tulotta and Ottewell46), whereas tumour-derived IL-1β promotes expansion of the bone metastatic niche resulting in tumour cell proliferation (Ref. Reference Tulotta5). Newly awakened and actively proliferating DTCs secrete a variety of growth factors, such as parathyroid hormone-related protein (PTHrP) and IL-1β, that stimulate the activity of osteoblasts and osteoclasts through the RANKL/RANK/OPG pathway leading to increased bone remodelling (reviewed in Refs Reference Tulotta and Ottewell46, Reference Rao50). Increased bone resorption leads to a positive feedback loop in which matrix-derived growth factors, such as TGF-β are released into the metastatic microenvironment further stimulating proliferation of metastatic tumour cells which, in turn, produce more PTHrP and IL-1β (Refs Reference Ottewell36, Reference Tulotta and Ottewell46, Reference Rao50). Moreover, IL-1β has been shown to promote expansion of the bone metastatic niche promoting additional tumour cells into the environment that can subsequently proliferate and increase metastatic burden. Taken together, these data suggest that IL-1β plays a vital role in this ‘vicious cycle’ of osteolytic metastasis (Fig. 2). The importance of IL-1β from both tumour cells and the microenvironment in this ‘vicious cycle’ have been demonstrated in mouse models: injecting BALB/c nude mice intravenously with MDA-MB-231-IL-1β+ resulted in mice developing significantly larger osteolytic lesions compared with mice injected with MDA-MB-231 wild-type cells (Ref. Reference Tulotta5). Similarly, IL-1β overexpressing MDA-MB-231 cells also lead to increased lytic lesions in BALB/c nude mice compared with wild-type cells following intra-cardiac injection. Although tumour cell-derived IL-1β clearly has effects on tumour growth and lesion development in bone, evidence suggests that either overall concentrations of IL-1β in the local environment or IL-1β secreted from other cell types within the tumour environment may dominate the tumourigenic effects of this cytokine as IL-1β−/− mice develop far fewer and much smaller bone metastases compared with, control, IL-1βfl/fl mice following intra-cardiac injection of E0771 cells (Ref. Reference Tulotta13). Furthermore, inhibiting IL-1β signalling in mouse bone by administration of anakinra or canakinumab significantly increases bone volume and inhibits both osteoclast and osteoblast activity compared with bones from control mice in several breast cancer models in vivo (Refs Reference Tulotta5, Reference Eyre18, Reference Holen19) and IL-1β has also been shown to have direct stimulatory effects on osteoblast and osteoclast proliferation/differentiation in vitro (Refs Reference Tulotta5, Reference He51). It is, therefore, likely that inhibition of IL-1β signalling prevents/reduces growth of breast cancer cells in bone through both inhibiting activation of dormant CSCs and through disrupting the vicious cycle.
IL-1β in tumour immune response
There are strong and clear evidence that IL-1β promotes breast cancer bone metastases and that IL-1β-targeted therapies are able to inhibit these pro-metastatic effects (Fig. 3). However, the effects of inhibiting IL-1β on primary breast cancers are still far from clear. Treatment of primary 4T1, E0771 and MDA-MB-231 cells with anakinra has led to contrasting results with most researchers showing increased growth of tumours in the fat pad (Refs Reference Tulotta5, Reference Voigt52). However, other studies have shown reduced primary tumour growth of 4T1 and MDA-MB-231 cells following administration of the same dose of anakinra (Refs Reference Holen19, Reference Voloshin20). Treatment with canakinumab seems to always lead to increased tumour growth and it is tempting to think that this may be because of differences in targeting signalling via the IL-1R with anakinra and neutralisation of IL-1β with canakinumab. However, data from IL-1β knockout mice suggest that the answer is not that simple: Tulotta et al. showed that E0771 cells grew more rapidly in IL-1β−/− C57BL/6 mice compared with control mice (IL-1βfl/fl) mice following orthotopic injection (Ref. Reference Tulotta13). Similarly, other researchers have shown that 4T1 tumours initially grow following injection into IL-1β−/− mice, but these quickly regress resulting in much smaller tumours in IL-1β−/− mice compared with control (Ref. Reference Kaplanov53). In contrast, Kiss et al. demonstrated delayed tumour growth in IL-1β−/− C57BL/6 mice following orthotopic injection with E0771 cells compared with control mice (IL-1βfl/fl) (Ref. Reference Kiss11). Although these data are contradictory, experiments have been performed in mice from a variety of backgrounds, with various immunological impairments and/or anti-IL-1 treatments have commenced at different stages of tumour growth. Effects of IL-1β in the microenvironment fluctuates between anti-tumour and pro-tumour through driving the infiltration of innate anti-tumour immune cells as well as establishing an immunosuppressive microenvironment by increasing the infiltration of myeloid-derived suppressor cells (MDSCs), therefore it is not surprising that IL-1β may have differing effects dependent on tumour stage and the immunological background of the host. Therefore, understanding the role of IL-1β in tumour immune response in breast cancer is crucial to the study of anti-IL-1β therapies in the treatment of breast cancer bone metastases.
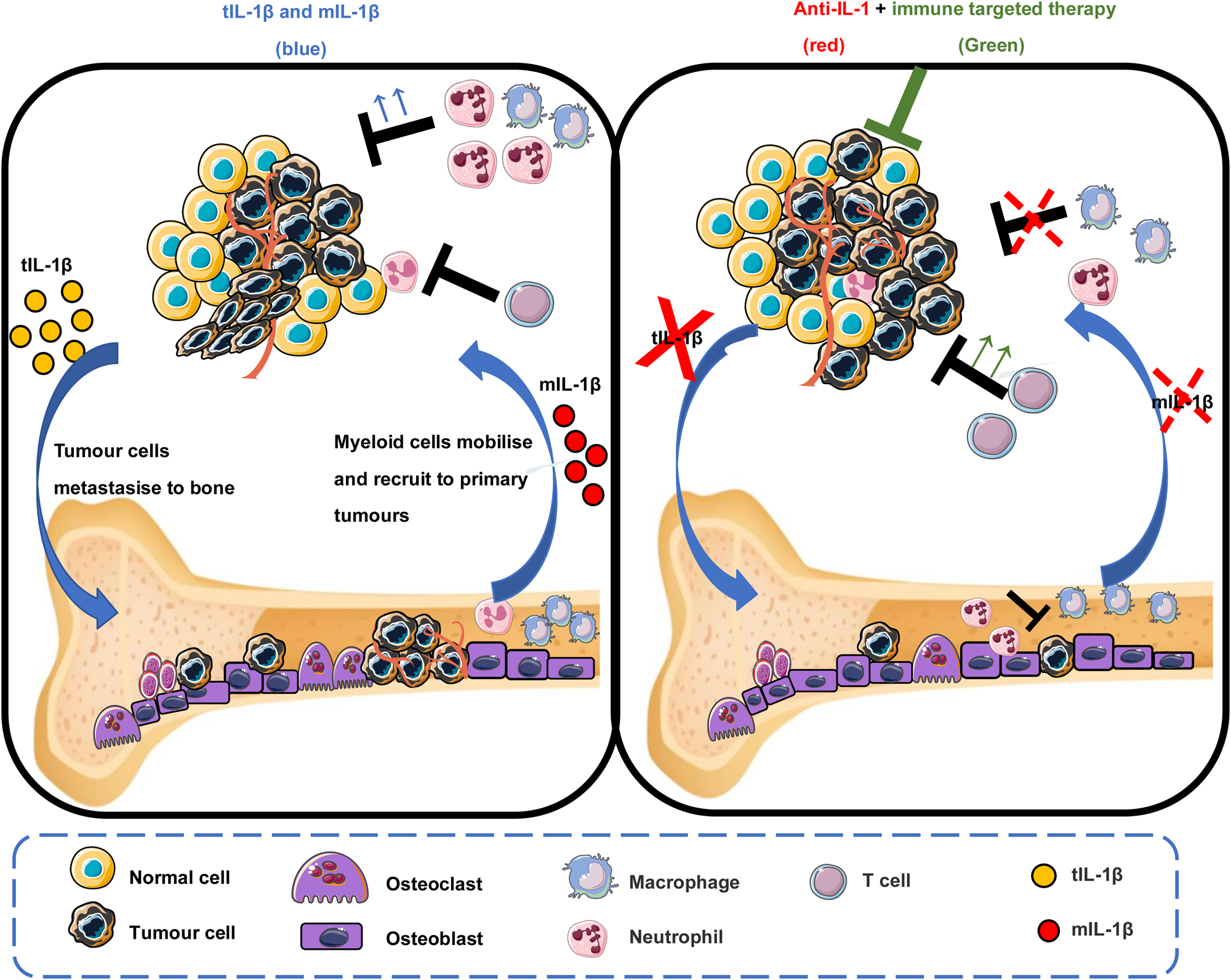
Fig. 3. Tumour and microenvironment-derived IL-1β in primary breast tumour and bone metastasis. In the primary tumour, microenvironment-derived IL-1β drives the recruitment of myeloid cells such as neutrophils that may act as a brake of tumour proliferation in situ. However, tumour-derived IL-1β induces EMT, promoting the initiation of the metastatic cascade, leading to cancer cell spreading to bone. In bone, IL-1β supports niche expansion and enables disseminated CSCs to form metastatic colonies. Inhibition of tumour- or microenvironment-derived IL-1β enhances primary tumour growth, however impairs bone metastasis, suggesting niche-dependent functions of IL-1β. Disabling both tumour and microenvironment-derived IL-1β by inhibiting IL-1β cognate receptor IL-1R1, in combination with therapies that stimulate immunogenic cell death, impairs both primary tumour growth and bone metastasis.
Neutrophils
Neutrophils display phenotypic and functional heterogeneity (Ref. Reference Silvestre-Roig54) and, in cancer, anti-tumourigenic N1-like or pro-tumourigenic N2-like phenotypes have been described (Ref. Reference Ohms55). Several cytokines, such as IL-1β produced by tumour cells, regulate neutrophil recruitment (Ref. Reference Chen56). In addition, neutrophils expressing high level of IL-1β also directly interact with CTCs to support cell cycle progression in circulation and to facilitate metastatic seeding (Ref. Reference Szczerba57). Although experiments performed by Tulotta et al. and Kiss et al. showed IL-1β−/− mice have different breast cancer outcomes, in terms of primary tumour growth, both studies showed reduced numbers of tumour infiltrating neutrophils in the primary site. Additionally, Kiss et al. reported reduced systemic mobilisation and numbers of neutrophils in the circulation in IL-1β−/− mice compared with control. Interestingly, Tulotta et al. found that the lack of microenvironment-derived IL-1β resulted in decreased infiltration of Myeloperoxidase (MPO)+ neutrophils which is consistent with anti-tumour effects observed in breast cancer patients where infiltration of MPO+ neutrophils have been associated with a significantly better overall survival (P < 0.001) (Refs Reference Tulotta13, Reference Zeindler58). Moreover, although Kiss et al. found that loss of microenvironment-derived IL-1β abrogated expansion of circulating CD45 + CD11b + Ly6G+ neutrophils (Ref. Reference Kiss11) increased CD45 + CD11b + Ly6G+ neutrophils have been found in the bone marrow of IL-1β−/− mice following intracardiac-injection of E0771 cells suggesting that IL-1β may be required to mobilise these neutrophils from the bone environment to soft tissue (Ref. Reference Tulotta13).
IL-1β has also been shown to regulate both pro- and anti-metastatic effects of neutrophils on the metastatic outgrowth of breast tumours in the lung. Xiao et al. demonstrated that production of cathepsin C by breast cancer cells in the lung activated IL-1β in local neutrophils. This, in turn leads to the recruitment of more neutrophils from the circulation, increases production of reactive oxygen species, finally leading to the formation of neutrophil extracellular traps (NETs). The release of these NETs in the lung causes degradation of thrombospondin 1, promoting metastatic outgrowth of tumour cells disseminated in this organ (Ref. Reference Xiao59). In contrast, Zhao et al. reported that IL-1β could induce the recruitment of anti-tumour neutrophils to the lung and this resulted in blocking lung metastasis (Ref. Reference Zhao60).
In a slight contradiction to the aforementioned studies, Comes et al. observed that inhibiting IL-1 by IL-1R antagonist had no significant effect on the numbers of neutrophils that infiltrated tumours, but, instead, reduced the polarisation of neutrophils to the N2-like phenotype (Ref. Reference Gomes61). Therefore, IL-1β may be able to exert dual effects, both recruiting neutrophils to the TME and stimulating their polarisation to a tumour-killing or tumour-promoting phenotype. The difference in recruitment or phenotype of neutrophils induced by IL-1β in primary tumours or soft tissue metastasis may lead to contradictory results. However, inhibition of IL-1β consistently impairs bone metastasis and whether this is regulated by neutrophils will require further studies.
Macrophages
Similarly to neutrophils, there are two populations of macrophages in the TME: M1-like and M2-like (Ref. Reference Chen62). M1-like macrophages are anti-tumour macrophages that can mediate cytotoxicity and antibody-dependent cell-mediated cytotoxicity to kill tumour cells; whereas M2-like macrophages have pro-tumour effects through the promotion of immunosuppression by inhibiting T cells (Ref. Reference Chen62). Macrophages are one of the main sources of IL-1β, and tumour cells can stimulate macrophages to produce IL-1β. IL-1β can also promote the infiltration and differentiation of monocytes into macrophages within the primary tumour: there is an auto-amplification loop between macrophages and breast cancer, resulting in further increased expression of IL-1β by both macrophages and breast cancer cells to promote the development of cancer (Ref. Reference Hou63). This hypothesis has been further confirmed in studies showing that macrophage depletion significantly decreases serum IL-1β and delays breast cancer progression in a mouse model (Ref. Reference Jang64). IL-1β-deficient mice, in which primary tumour growth is significantly delayed, have far fewer macrophages compared with their control counterparts (Ref. Reference Kaplanov53). Moreover, in the absence of IL-1β, macrophages have been confirmed to be important for the accumulation and activation of CD8+ cells in breast cancer (Ref. Reference Kiss11).
Macrophage number is not the only factor that influences the effects of this immune cell type on tumourigenesis. Several studies show that polarisation of macrophages from an M1-like to an M2-like phenotype is a more important driver of this process (reviewed in Ref. Reference Hao65), and in addition to promoting anti-tumour effects, IL-1β can also induce pro-tumour effects through altering macrophage polarisation and location: mouse model studies comparing tumours isolated from IL-1β−/− and IL-1βfl/fl mice demonstrated that in the presence of IL-1β in the microenvironment the majority of macrophages recruited to the tumour were inducible nitric oxide synthase (iNOS+) M1-like macrophages and no differences in infiltration of CD163+ M2-like macrophages were observed. Moreover, IL-1β appeared to be important for determining the location of these cells. In primary tumours isolated from control (IL-1βfl/fl) mice, iNOS+ cells have been shown to be located in viable areas of tumours whereas CD163+ macrophages localise to areas of necrosis. This locational bias is lost in IL-1β−/− mice (Ref. Reference Tulotta13) suggesting that IL-1β attracts tumour-killing M1-like macrophages away from the areas of necrosis and into healthy tumour tissue where they can exert maximum anti-tumour effects. These data further explain how microenvironment-derived IL-1β can reduce primary tumour by recruitment and infiltration of innate immune cells.
Although IL-1β appears to exert significant anti-tumour effects on primary tumours through the recruitment of macrophages, the same does not seem to happen in the bone microenvironment. The presence of CD45 + CD11b+ myeloid cells is significantly lower in bone marrow compared with primary tumours (Ref. Reference Kiss11) furthermore no significant differences in the accumulation of CD45 + CD11b + F4/80+ macrophages have been observed in the bone microenvironment between IL-1β−/− and IL-1βfl/fl mice following intra-cardiac injection of E0771 cells (Ref. Reference Tulotta13). It, therefore, appears that IL-1β may exert different effects on anti-tumour immunity in soft tissues compared with bone.
Myeloid-derived suppressor cells
MDSCs (Gr + CD11b+) are a heterogeneous population of cells of myeloid origin composed of immature macrophages, immature neutrophils and DCs. Therefore, the recruitment and mobilisation methods and functions of MDSC are similar to immunosuppressive neutrophils and macrophages (Ref. Reference Welte66). MDSCs can induce apoptosis of cytotoxic T lymphocytes and natural killer cells to participate in the formation of an immune suppressed, pre-metastatic niche and subsequent development, progression and metastasis of breast cancer (Ref. Reference Welte66). Tumour cells induce accumulation of MDSCs and tumour-derived IL-1β has been shown to facilitate an earlier and faster accumulation of MDSCs, in vivo (Ref. Reference Bunt67). IL-1β also stimulates MDSC-induced suppression of both CD4+ and CD8+ T cells via an arginase-dependent mechanism (Ref. Reference Bunt67). Moreover, pharmacological inhibition of IL-1R can reduce MDSC accumulation and inhibit tumour growth and metastasis by reducing the expression of G-CSF in mouse breast cancer models (Ref. Reference Gomes61).
T lymphocytes
T lymphocytes (CD4+ and CD8+) are an essential part of the immune system that regulate the adaptive immune response to cancer. CD8+ T cells kill tumour cells whereas CD4+ T cells assist B cells in regulating the immune response to a particular antigen. IL-1β has well-defined roles in facilitating adaptive T cell-mediated immunity and supporting expansion and maturation of CD4+ and CD8+ T cells in early tumour development (Ref. Reference Van Den Eeckhout22). Primary tumours isolated from IL-1β-deficient mice have increased CD8+ cytotoxic T cells and secrete high-concentrations of IFN-γ, TNF-α, granzyme B and IL-12 but have low levels of IL-10, which co-reduce immunosuppression (Ref. Reference Kaplanov53). However, as described, IL-1β can also mediate the recruitment and mobilisation of MDSCs to suppress T cell-mediated immunity (Ref. Reference Kiss11). In addition, it has been found that IL-1β can activate γδT cells to express IL-17, which in turn polarises and expands granulocyte colony stimulating factor-dependent neutrophils, thereby suppressing the production of CD8+ T cells and promoting breast cancer metastasis (Ref. Reference Coffelt68). There is currently no evidence to show whether the level of T cell immunity stimulated by IL-1β is different between primary tumours and the bone metastatic niche, and further studies are required to understand the role of IL-1β in immune cell regulation of bone metastasis.
Discussion and concluding remarks
Inhibiting IL-1R1 activation (with the anti-IL-1β antibody, canakinumab or the IL-1R antagonist, anakinra) has shown significant and beneficial effects, almost eliminating bone metastasis in a number of pre-clinical models of breast cancer (Refs Reference Tulotta5, Reference Tulotta13, Reference Eyre18, Reference Holen19). Similarly, canakinumab has been shown to have potent anti-cancer effects in the worldwide canakinumab Anti-inflammatory Thrombosis Outcomes Study (Ref. Reference Ridker69). However, it cannot be ignored that blocking IL-1R1 activity also increased the risk of primary tumour growth and outgrowth of disseminated mammary tumour cells in soft tissues in mice. Current data suggest that in the bone IL-1β stimulates tumour growth through expansion of the bone metastatic niche (Ref. Reference Tulotta5) activation of previously dormant breast CSCs (Ref. Reference Eyre18) stimulation of the vicious cycle of bone metastasis (Ref. Reference Tulotta5) and suppression of anti-cancer immune cells (Refs Reference Tulotta13, Reference Guo21, Reference Kaplanov53). In contrast, the role of IL-1β in the growth of primary tumours appears to be driven by immune cell regulation, but remains controversial. Therefore, in order to use anti-IL-1 therapies for the treatment of bone metastasis, it is likely that these would need to be combined with therapies that stimulate anti-tumour immunity (Fig. 3). Initial experiments combining anakinra with drugs that stimulate immunogenic tumour cell death (doxorubicin) or through stimulation of an anti-tumour T cell response (anti-PDL-1 antibodies) look extremely promising: combining anakinra with the standard of care for bone metastasis zoledronic acid and doxorubicin significantly reduced primary tumour growth and metastatic outgrowth in bone and soft tissues in 4T1 and E0771 mouse models (Ref. Reference Tulotta13). Furthermore, treating IL-1β−/− mice with an anti-PD-1 antibody reversed IL-1β-induced 4T1 primary tumour growth and sequential combination with anti-IL-1 treatment followed by PDL-1 antibody completely abrogated tumour progression (Ref. Reference Kaplanov53). The positive outcomes of these preclinical studies provide strong rationale for the commencement of clinical trials combining IL-1-targeted treatments with anti-tumour immune stimulatory drugs for primary breast cancer and metastatic disease.
Acknowledgements
Work associated with regulation of IL-1B in bone metastasis was funded by the Medical Research Council, UK, Ref. MR/P000096/1 and Weston Park Cancer Charity, UK, Ref. CA142 awarded to Penelope Ottewell and Claudia Tulotta is recipient of a Humboldt Postdoctoral Fellowship.