Introduction
The implementation of integrated crop–livestock (iCL) and crop–livestock–forest (iCLF) systems is an alternative for pasture intensification, which increase farming sustainability (Balbino et al., Reference Balbino, Cordeiro, Silva, Moraes, Martinez, Alvarenga, Kichel, Fontaneli, Santos, Franchini and Galerani2011; Figueiredo et al., Reference Figueiredo, Jayasundara, Bordonal, Reis, Wagner-Riddle and La Scala2017; Salton et al., Reference Salton, Mercante, Tomazi, Zanatta, Concenço, Silva and Retore2014). Integrated systems improve land use efficiency (Carvalho et al., Reference Carvalho, Raucci, Cerri, Bernoux, Feigl, Wruck and Cerri2010; Garrett et al., Reference Garrett, Nilesb, Gila, Gaudine, Chaplin-Kramerf, Assmanng, Assmannh, Brewere, Carvalhoi, Cortnera, Dynesj, Garbachk, Kebreabl, Muellerm, Petersone, Reisn, Snowj and Valentimo2017; Gil et al., Reference Gil, Siebold and Berger2015). Furthermore, when trees take part in these systems, positive effects on microclimate variables (Benavides et al., Reference Benavides, Douglas and Osoro2009) and an increase in carbon sequestration are observed, which expand the possibilities of using these systems to mitigate climate change effects (Almeida et al., Reference Almeida, Oliveira, Macedo and Pezzopane2011; Amatya et al., Reference Amatya, Chang, Beare and Mead2002; Oliveira et al., Reference Oliveira, Macedo, Venturin and Higashikawa2009).
Systems, such as iCLF, are more complex than monocultures. The presence of more than one vegetal layer or several plant and animal species promotes interactions that vary in time and space (Jose et al., Reference Jose, Gillespie and Pallardy2004; Pezzopane et al., Reference Pezzopane, Bosi, Nicodemo, Santos, Cruz and Parmejiani2015). The magnitude of these interactions, with positive or negative results, is determined by the patterns of resource partitioning (mainly water, solar radiation, and nutrients) and by the age of the system components (Gillespie et al., Reference Gillespie, Jose, Mengel, Hoover, Pope, Seifert, Biehle, Stall and Benjamin2000; Rivest et al., Reference Rivest, Paquette, Moreno and Messier2013).
Characteristics, arrangement, and population density of trees in iCLF systems cause environmental changes, which reflect on plant and animal productivity. Numerous studies on iCLF systems, conducted in many Brazilian regions, concluded that competition between components becomes significant 4 or 5 years after their implementation, depending on the arrangement and population of trees, reducing yield of pastures or crops (Magalhães et al., Reference Magalhães, Pedreira, Tonini and Farias Neto2018; Pedreira et al., Reference Pedreira, Domiciano, Rodrigues, Moraes, Magalhaes, Matos, Zolin and Medeiros2017; Pezzopane et al., Reference Pezzopane, Bernardi, Bosi, Oliveira, Marconato, Pedroso and Esteves2019; Santos et al., Reference Santos, Guimarães Junior, Vilela, Maciel and França2018). Reductions in pasture yield were also identified in regions subjected to severe water deficits, such as the cattle-raising regions of Australia and New Zealand (Benavides et al., Reference Benavides, Douglas and Osoro2009; Pollock et al., Reference Pollock, Mead and McKenzie2009; Rivest et al., Reference Rivest, Paquette, Moreno and Messier2013). In these countries, adverse effects are more significant in regions with annual rainfall lower than 900 mm.
Thinning and pruning of trees may contribute to maintaining a good balance between the yields of the several components of iCLF systems. Pasture and crop yields may be increased by thinning in these systems. Thinning may be performed by removing alternate tree rows (increasing spacing between rows) or alternate trees in the rows (increasing spacing between trees in the rows), which reduces competition for water and solar radiation (Nicodemo et al., Reference Nicodemo, Castiglioni, Pezzopane, Tholon and Carpanezzi2016; Reynolds et al., Reference Reynolds, Simpson, Thevathasan and Andrew2007; Paciullo et al., Reference Paciullo, Carvalho, Aroeira, Morenz, Lopez and Rossiello2007). Another alternative to reduce competition in these systems is the use of deciduous trees, which increase soil water availability for understory plants during the growth season (Pollock et al., Reference Pollock, Mead and McKenzie2009).
The goal of this study was to evaluate the effects of thinning eucalyptus trees on yield and nutritive value of corn for silage and palisadegrass in an iCLF system and to evaluate the aboveground biomass yield in systems with and without trees. These information may contribute to the elucidation of these complex systems, with the goal of filling gaps in knowledge and encouraging the adoption of iCLF by farmers.
Material and Methods
This study was conducted in two integrated systems, an iCL and an iCLF, at Embrapa Pecuária Sudeste, São Carlos, state of São Paulo, Brazil (21°57′S, 47°50′W, 860 m a. s. l.), from October 2016 to April 2018. The climate of this location is Cwa (Köppen), with a dry season (from April to September) with an average temperature of 19.9 °C and total rainfall of 250 mm and a wet season (from October to March) with an average temperature of 23.0 °C and total rainfall of 1100 mm. The local relief is flat to mildly hilly and the soil a Dystrophic Red-Yellow Latossol with sandy loam texture (Calderano Filho et al., Reference Calderano Filho, Santos, Fonseca, Santos, Primavesi and Primavesi1998). Each integrated system was 6 ha, split into two experimental areas with 3 ha each. These areas were divided into six 0.5 ha paddocks and managed as rotational stocking systems. In the iCLF system, eucalyptus trees (Eucalyptus urograndis clone GG100) were planted in April 2011, in single rows, with a near east-west orientation and a 15 × 2 m spacing (15 m between rows and 2 m between trees in the rows), which resulted in a population density of 333 trees ha−1. In July 2016, trees were thinned, which consisted in cutting 50% of the trees in each row and spacing changed to 15 × 4 m (165 trees ha−1) (Supplementary Material Figure S1). During the 2016/2017 season, eucalyptus trees were about 27.2 m high and 24.0 cm in diameter at breast height (DBH) and, in the 2017/2018 season, 28.6 m high and 26.3 cm in DBH.
Pasture renovation was performed in four paddocks per integrated system (Supplementary Material Figure S2). For this, corn (Zea mays L. hybrid AG 8690 PRO 3) was sown first (Supplementary Material Figure S1), and palisadegrass [Urochloa (syn. Brachiaria) brizantha (Hochst ex A. Rich.) Stapf cv. BRS Piatã] was sown after the corn was harvested for ensilage. Initially, the local soil was corrected by applying dolomitic lime to achieve 70% base saturation. Corn was sown mechanically on November 8, 2016, with 0.8 × 0.2 m spacing, with a goal of achieving a population of 62,500 plants ha−1. Corn was fertilized at sowing (40 kg of N, 140 kg of P2O5, and 80 kg of K2O ha−1) and 30 days after emergence (100 kg of N, 25 kg of P2O5, and 100 kg of K2O ha−1) and harvested on February 22, 2017. Palisadegrass was mechanically sown on February 24, 2017, with a spacing of 0.4 m between sowing lines and 10 kg of seeds ha−1 (5 kg of pure viable seeds ha−1). The pasture was kept under free growth conditions until June 13, 2017, when grazing started. Grazing was performed by Nelore and Canchim (3/8 Nelore + 5/8 Charolais) bulls, which were 11 months old and weighed 200 kg on average when put in the systems.
For assessments of corn and pasture variables, five treatments were considered, with the iCL system as a single treatment and the iCLF system split into four treatments, consisting of four positions relative to the row of trees positioned to the north. For this, it was considered that the rows of trees to the north were responsible for shading in the assessed areas during most of the year because of solar declination. These treatments were 1.90 m (iCLF_1), 3.75 m (iCLF_2), 7.50 m (iCLF_3), and 11.25 m (iCLF_4) from the north row (Supplementary Material Figure S3).
Corn for silage was assessed at harvest when dry matter content was approximately 30%. Corn plants were cut at 0.3 m above the ground, in four repetitions and with three sampling sites of 0.48 m2 (three linear meters in two sowing lines) per repetition. Each sample was weighed, and 10 plants were taken for evaluation of plant and ear insertion heights and proportion of grains in the total mass. The remaining plants of the samples were mixed and milled, and a subsample of 500 g per repetition was taken to determine dry matter content by drying in an air forced dry oven (65 °C) until constant weight.
Pasture assessments were conducted only in paddocks in which pasture was renovated with corn (Supplementary Material Figure S2), and in four cycles representing each season of the year: autumn (from February 24 to June 13, 2017), with the pasture under free growth; winter (from August 1 to September 4, 2017); spring (from November 17 to December 21, 2017); and summer (from January 8 to February 9, 2017) cycles under grazing. In the winter, spring, and summer cycles, forage was collected in post and pre-grazing to calculate forage accumulation during each rest period. In the autumn cycle, the pre-grazing forage mass was considered as forage accumulation. Grazing was managed as rotational stocking with cycles having 6 days of occupation and 30 days of rest.
Pasture assessments were performed in four repetitions by cutting all the grass at ground level, in four sampling sites of 0.25 m2 per repetition, with a metallic 0.5 × 0.5 m square frame randomly positioned for each treatment. Pasture height, from the ground to the most recently expanded leaf, was measured at three points within the frame for each sample. The grass samples were individually weighed and mixed with the other samples from the same repetition. Subsamples of 200 g were taken to determine dry matter content by drying in an air forced dry oven (65 °C, 72 h) and to perform plant part composition. From the latter, the leaves were taken to measure leaf area using a leaf area meter, LI-3100 (Lincoln, Nebraska, USA), and to determine leaf area index (LAI) and specific leaf area (SLA).
Corn and grass subsamples used to assess dry matter content were also used to determine crude protein (CP) content and in vitro dry matter digestibility (IVDMD). For this, the subsamples were ground in a Wiley mill with a 0.5 mm sieve. Corn subsamples were analyzed to determine CP (AOAC, 1990) and IVDMD according to Moore and Mott (Reference Moore and Mott1974). Grass subsamples were analyzed by the Fourier transform-near infrared (NIR) technique using a spectrometer model NIRFlex N-500 with polarization interferometer (Büchi, Flawil, Switzerland). These measurements were performed using a calibration model developed and validated by Embrapa Pecuária Sudeste (R2 = 0.944 for CP and R2 = 0.923 for IVDMD) specifically for species and cultivars of Urochloa spp.
Half-yearly, between October 2016 and April 2018, trunk volume and total biomass of the eucalyptus trees were estimated for each experimental paddock of the iCLF system using allometric equations developed for this kind of system (Pezzopane et al., Reference Pezzopane, Bosi, Bernardi, Muller, Oliveira and Laclau2018). These equations require measurements of DBH (at 1.3 m from the ground) and tree height, which were measured with a diametric tape and a Haglof hypsometer, respectively.
The total aboveground biomass yield was determined for each system by summing the data of aboveground biomass of the corn for silage, the palisadegrass, and eucalyptus trees. Three pasture growth cycles were not assessed, since the focus of this study was to evaluate only one cycle per season of the year. In the case of corn, a factor was considered to correct the mass yield calculation, considering the area where it was not possible to sow the crop because of the presence of trees, which was 20.8% of the total area. For the pasture, a correction factor for area was not necessary since pasture was present in the entire area.
Soil moisture, in treatments iCL, iCLF_1, and iCLF_3 (the most contrasting treatments), was measured weekly in the 0–1 m depth layer with a capacitance probe (Diviner 2000®) in four replicates. Photosynthetically active radiation (PAR) was measured continuously with linear quantum sensors CQ311 (Apogee, Logan, Utah, USA) at the four iCLF positions and with a point quantum sensor CS110 (Apogee, Logan, Utah, USA) in the iCL system. These sensors were connected to a datalogger CR1000 (Campbell Scientific, Logan, Utah, USA) programmed to take measurements every 10 s, recording averages every 15 min, and total daily values. The PAR transmission was calculated by dividing the PAR incidence at each position in the iCLF system by the PAR incidence in the iCL system.
Data of pasture, corn variables, and total aboveground biomass yield were statistically analyzed through a complete randomized block design with four repetitions. The treatments for corn and pasture variables were the treatments iCL, iCLF-1, iCLF-2, iCLF-3, and iCLF-4 and for total aboveground biomass yield, the systems iCL, and iCLF. Data were subjected to analysis of variance using the PROC ANOVA of SAS (Schlotzhauer and Littell, Reference Schlotzhauer and Littell1987), and means were compared using the T test at the 5% significance level. For pasture variables, one analysis was performed per season of the year to compare the treatments.
Results
During the corn cycle, average PAR in the iCL system was 9.7 MJ m−2 d−1, and the average PAR transmission in the iCLF system was 65.3%, varying from 55.6% to 73.1% between the four positions (Figure 1). During the pasture growth cycles, PAR in the iCL positions ranged from 6.6 to 9.3 MJ m−2 d−1, with lower values in the autumn and winter cycles. The PAR transmission at the four positions in the iCLF system ranged from approximately 50% in the autumn and winter cycles, with homogeneity between positions, to 60% in the spring and summer cycles, achieving 70% in the iCLF_2 and iCLF_3 positions (Figure 1). Average daily air temperature varied between 12 and 28 °C, averaging 21.4 °C during the experimental period (Figure 2a). Rainfall was normal for the region, with good water supply in spring and summer and reduced amounts in late autumn and winter (Figure 2a). In the winter pasture growth cycle, rainfall was only 20.2 mm. Total rainfall for the corn cycle was 663 mm.
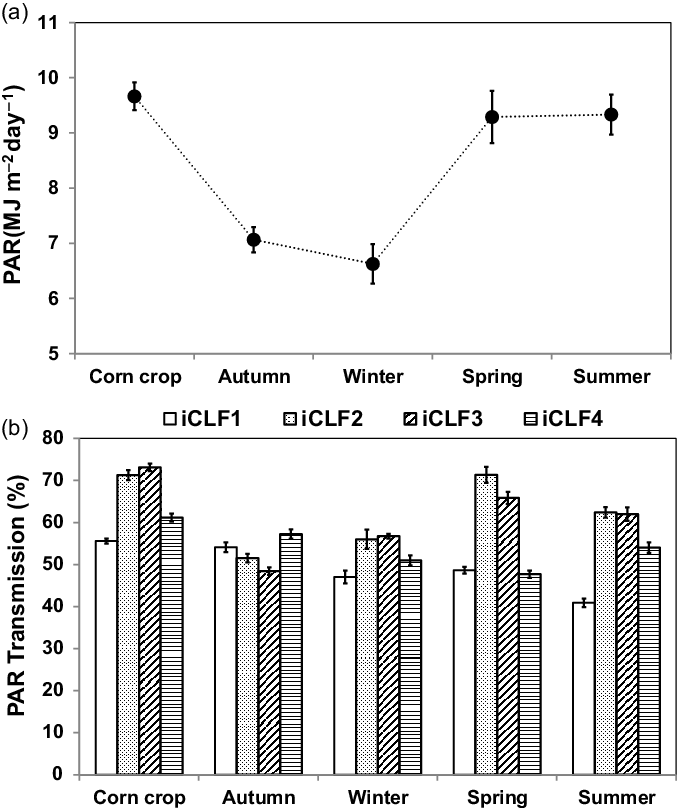
Figure 1. Photosynthetically active radiation (PAR) incidence in the integrated crop–livestock system (a) and PAR transmission (b) in four positions (iCLF_1: 1.9 m, iCLF_2: 3.75 m, iCLF_3: 7.50 m, and iCLF_4: 11.25 m from the north row of trees) within the integrated crop–livestock–forest system during the corn for silage cycle (corn crop) and pasture growth cycles (autumn, winter, spring, and summer). Vertical bars represent standard error of the means.
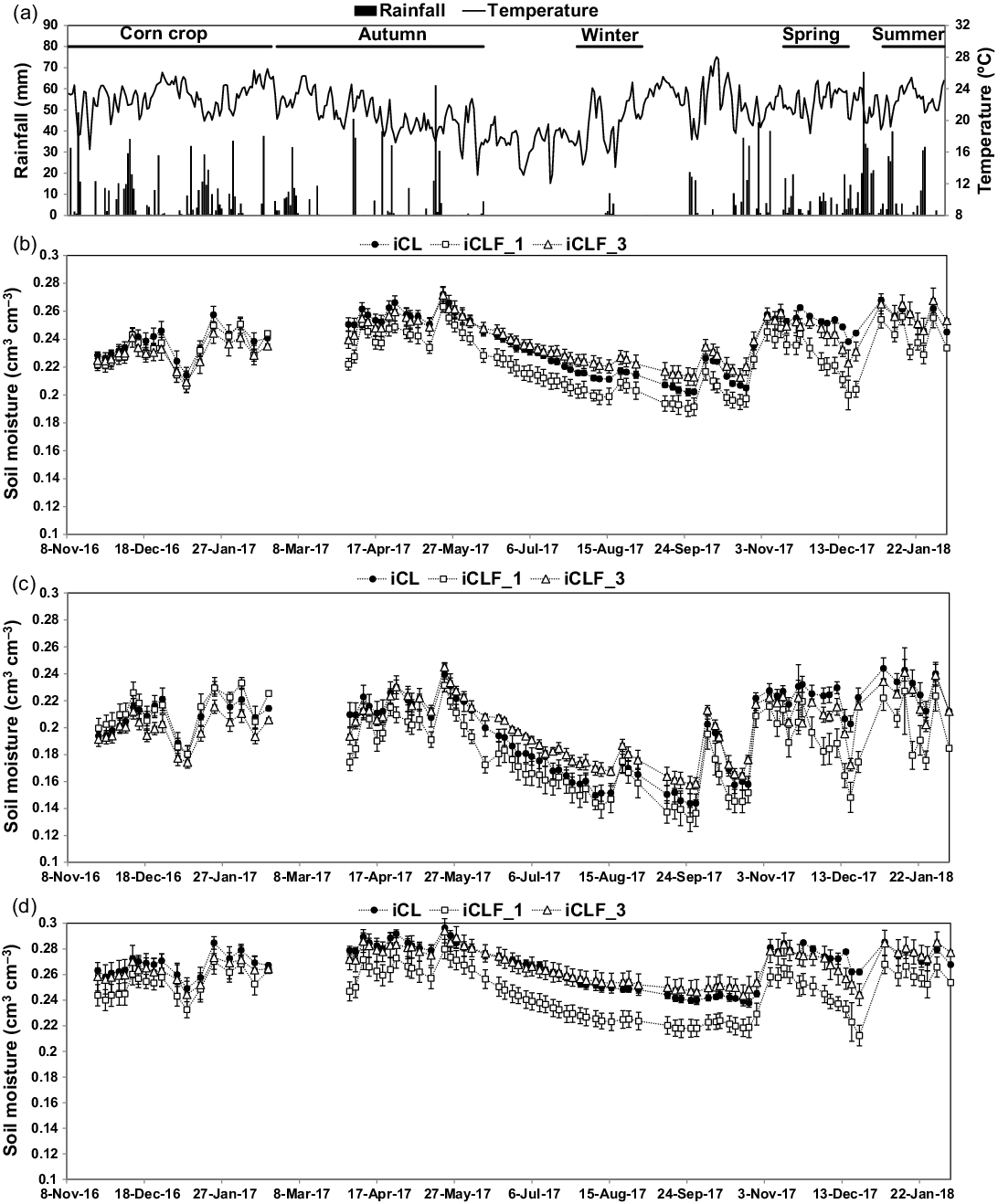
Figure 2. Air temperature (°C) and rainfall (mm) (a), and soil moisture (cm3 cm−3) in the layers 0.0–1.0 m (b), 0.0–0.5 m (c), and 0.5–1.0 m (d) during the corn for silage cycle (corn crop) and pasture growth cycles (autumn, winter, spring, and summer) in the integrated crop–livestock system (iCL) and in two positions (1.9 m, iCLF_1 and 7.5 m, iCLF_3) for the integrated crop–livestock–forest system: Vertical bars represent standard error of the means.
Soil moisture in the 0–1 m layer was between 0.202 and 0.272 cm3 cm−3 in iCL, 0.190 and 0.263 cm3 cm−3 in iCLF_1, and 0.209 and 0.271 cm3 cm−3 in iCLF_3 (Figure 2b). In the 0.0–0.5 m layer, it varied between 0.144 and 0.244 cm3 cm−3, 0.132 and 0.233 cm3 cm−3, and 0.157 and 0.245 cm3 cm−3, for the same positions, respectively (Figure 2c). In the 0.5–1.0 m layer, soil moisture ranged from 0.238 to 0.296 cm3 cm−3, 0.212 to 0.280 cm3 cm−3, and 0.244 to 0.294 cm3 cm−3 for the same positions, respectively (Figure 2d). During the corn cycle, soil moisture was similar between the assessed positions and throughout the soil profile as a result of high rainfall. In the driest period of the experiment (between May and September 2017), soil moisture was lower at iCLF_1, mainly because of the lower values observed in the 0.5–1.0 m layer (Figure 2d). This indicated higher water consumption in this position because of water uptake by the tree roots. In the 0.0–0.5 m layer, iCLF_3 presented higher soil moisture than iCLF_1 and iCL (Figure 2c). This pattern persisted during the soil water recharge at the end of the dry period (between September and December 2017). From December 2017 to April 2018, after high rainfall, soil moisture was again similar between the assessed positions.
The corn variables for plant and ear insertion heights were different between treatments (P = 0.0175 and P = 0.0009, respectively), with higher values at iCLF_2, iCLF_3, and iCLF_4 than in iCL (Table 1). Corn presented higher dry matter content in iCL than in the iCLF positions (P = 0.0152). No differences were observed between treatments for corn biomass yield, which averaged 13.57 Mg ha−1. The proportion of grains in the total biomass was higher in iCLF_2, iCLF_3, and iCLF_4 than in iCL. Corn plants in the treatments of the iCLF system presented higher CP content than in iCL (P < 0.0001), but no differences in IVDMD were observed.
Table 1. Vegetative and productive characteristics of corn for silage in an integrated crop–livestock system (iCL) and four positions (iCLF_1: 1.9 m, iCLF_2: 3.75 m, iCLF_3: 7.50 m, and iCLF_4: 11.25 m from the north row of trees) within an integrated crop–livestock–forest system during the 2016/2017 crop

1 Means followed by the same letter in a column are not different by t test (P < 0.05).
2 IVDMD: In vitro dry matter digestibility.
Considering pasture variables, pasture height was higher in iCLF_2 and iCLF_3 than in the other treatments, during the autumn, higher in iCLF_2 than in iCL, iCLF_3, and iCLF_4, during winter, and no differences were observed for this variable in the spring and summer cycles (Table 2). LAI was not different between the five treatments, averaging 0.9 in the winter cycle and 2.7 in the autumn and summer cycles. SLA was higher at iCLF_3 than at the other treatments (P = 0.0210) in the winter cycle and lower in iCL than at the iCLF_1 and iCLF_4 (P = 0.0109) in the spring cycle. Forage accumulation was not different between treatments (Table 3), with average values ranging from 2262.4 kg ha−1 in the spring cycle to 375 kg ha−1 in the winter cycle. Pre-grazing forage mass was higher in iCL and at iCLF_3 than at iCLF_1 and iCLF_2 in the spring and summer cycles. It varied from 1806 kg ha−1 to 5260 kg ha−1, highlighting the increase in forage mass in the first year after pasture sowing. CP content was higher in the iCLF positions than in iCL, except in the summer cycle, when it was similar among treatments. IVDMD was higher in iCL than in iCLF_1, iCLF_2, and iCLF_4 (P = 0.0431) in the autumn cycle and higher in iCL than at the iCLF positions (P = 0.0246) in the winter cycle. No differences were observed for IVDMD in the spring and summer cycles.
Table 2. Vegetative characteristics of palisadegrass in an integrated crop–livestock system (iCL) and in four positions (iCLF_1: 1.9 m, iCLF_2: 3.75 m, iCLF_3: 7.50 m, and iCLF_4: 11.25 m from the north row of trees) within an integrated crop–livestock–forest system during four growth cycles
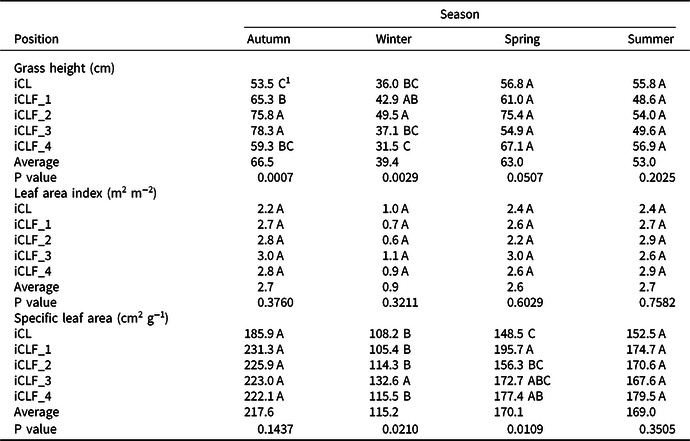
1 Means followed by the same letter in a column are not different by t test (P < 0.05).
Table 3. Productive characteristics of palisadegrass in an integrated crop–livestock system (iCL) and in four positions (iCLF_1: 1.9 m, iCLF_2: 3.75 m, iCLF_3: 7.50 m, and iCLF_4: 11.25 m from the north row of trees) within an integrated crop–livestock–forest system during four growth cycles
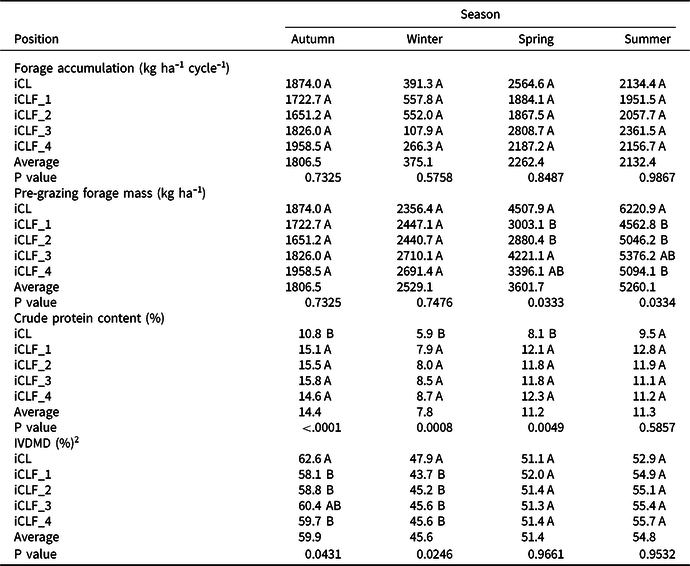
1 Means followed by the same letter in a column are not different by t test (P < 0.05).
2 IVDMD: In vitro dry matter digestibility.
After thinning, the remaining trees presented an increase in trunk volume (85.68–120.07 m3 ha−1; Figure 3a) and in aboveground biomass (41.29–55.41 Mg ha−1; Figure 3b) between October 2016 and April 2018. The total aboveground biomass yield of the corn, the palisadegrass, and eucalyptus together was 20.97 and 30.09 Mg ha−1 in iCL and iCLF systems, respectively, differing statistically (P = 0.0202) (Figure 3c).
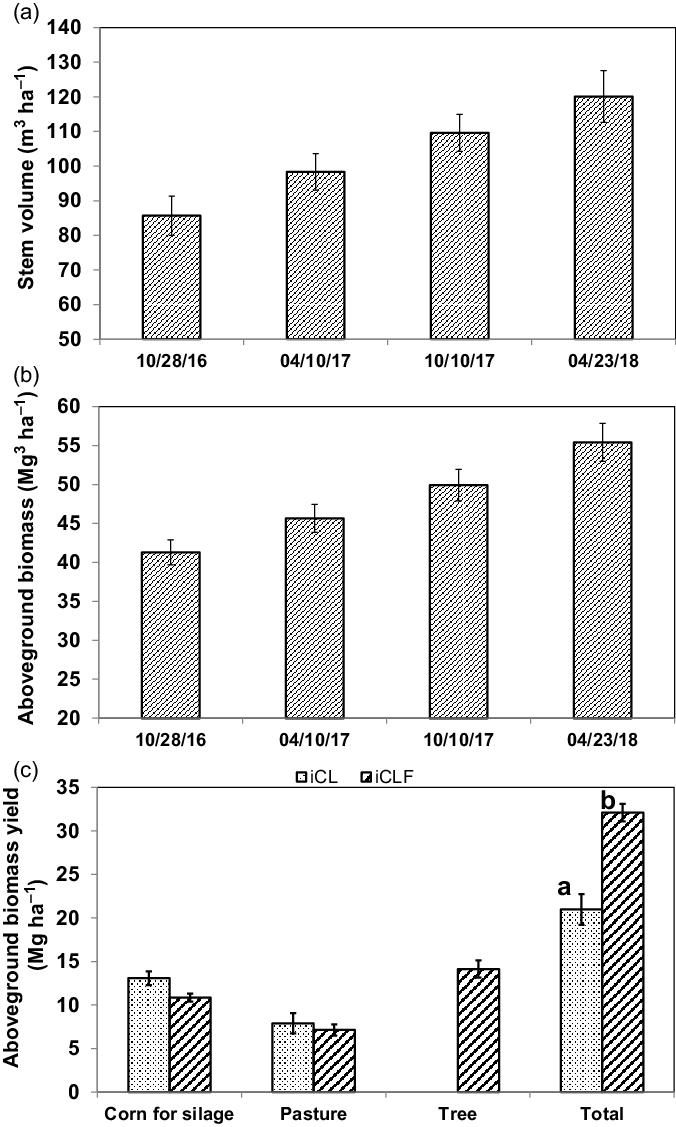
Figure 3. Stem volume (a) and aboveground biomass (b) of eucalyptus trees in an integrated crop–livestock–forest system with a population density of 166 trees ha−1, between October 2016 (78 months after planting) and April 2018 (96 months after planting). Aboveground biomass yield (c) of trees, corn for silage, and palisadegrass, separately and together, in an integrated crop–livestock system (iCL) and in an integrated crop–livestock–forest system (iCLF). Means of total aboveground biomass yield followed by the same letter are not different by t test (P < 0.05).
Discussion
The thinning of the trees, which reduced tree population density from 333 to 165 trees ha−1, increased PAR transmission in the iCLF positions as compared to that of other studies conducted in the same area (Bosi et al., Reference Bosi, Pezzopane and Sentelhas2020; Pezzopane et al., Reference Pezzopane, Bernardi, Bosi, Oliveira, Marconato, Pedroso and Esteves2019). This increase was more intense in the autumn and winter periods because of the near east-west orientation of the tree rows and the local latitude, which together with high solar declination promoted shading throughout the inter-row areas. During the corn cycle (late spring and early summer), PAR transmission at iCLF_1 was 55.6%, whereas in the study of Pezzopane et al. (Reference Pezzopane, Bernardi, Bosi, Oliveira, Marconato, Pedroso and Esteves2019), it was 44.6% in the same system. In this study, average PAR transmission in all iCLF positions was 65% during the corn crop production. During the pasture growth cycles of autumn and winter, PAR transmission was lower and more homogeneous between the iCLF positions than in other seasons. In spring and summer, PAR transmission was higher at iCLF_2 and iCLF_3 than at iCLF_1 and iCLF_4. PAR transmission values at iCLF_1 observed in this study were similar to that found by Prasad et al. (Reference Prasad, Korwar, Rao, Mandal, Rao, Rao, Ramakrishna, Venkateswarlu, Rao, Kulkarni and Rao2010) in an agroforestry system in India and by Pezzopane et al. (Reference Pezzopane, Bosi, Nicodemo, Santos, Cruz and Parmejiani2015) and Simão et al. (Reference Simão, Gontijo Neto, Oliveira Neto, Galvão, Borghi, Martins and Resende2018) in Brazil.
When PAR transmission during the corn cycle of 2016/2017 was compared with the same period in 2017/2018, a decrease in its value was identified (from 65.3 to 54.8%). This decrease was an effect caused by the lateral growth of the tree canopies, which is an adaptation strategy of trees to occupy the spaces left by the thinned trees. In this case, the highest decrease in PAR transmission occurred at iCLF_1, which was the position most affected by the trees.
Competition for water in agroforestry systems may or may not be essential for plant production, depending on the local climate. In general, competition for water was more relevant in regions with dry periods in summer (Benavides et al., Reference Benavides, Douglas and Osoro2009; Pollock et al., Reference Pollock, Mead and McKenzie2009). In our study, no relevant differences were observed in soil water content between positions, especially in the 0.0–0.5 m layer. In the driest period of our experiment, iCLF_3 had higher soil water content than did iCL. Bosi et al. (Reference Bosi, Pezzopane and Sentelhas2019) also observed higher soil water content in a silvopastoral system compared with a pasture without trees during some dry periods. This may be a consequence of the positive effect of shading in reducing evapotranspiration (Benavides et al., Reference Benavides, Douglas and Osoro2009). Lower soil moisture was observed in the 0.5–1.0 m layer at iCLF_1 compared with that of iCLF_3 and iCL, which may be attributed to higher water uptake by the tree roots and higher drainage caused by better soil structure promoted by the tree root systems (Benavides et al., Reference Benavides, Douglas and Osoro2009; McIvor et al., Reference McIvor, Douglas, Hurst, Hussain and Foote2008).
Despite the increase in PAR incidence in the iCLF positions promoted by the thinning of trees, corn plants still presented morphologic alterations when compared to the corn grown in iCL. Aspects related to light quality (red:near red) under the tree canopies may have influenced apical dominance, promoting higher plant and ear insertion heights, which affected corn morphological characteristics (Sangoi et al., Reference Sangoi, Gracietti, Rampazzo and Bianchet2002). Taking into account the microclimate changes promoted by thinning, this practice was considered beneficial for corn yield, because no differences were identified for this variable between iCL and iCLF positions. In the same experimental area, Pezzopane et al. (Reference Pezzopane, Bernardi, Bosi, Oliveira, Marconato, Pedroso and Esteves2019) observed competition and lower corn for silage yield at iCLF_1 before the thinning (2014/2015 crop). This was also observed in other studies in tropical regions, for oat (Nicodemo et al., Reference Nicodemo, Castiglioni, Pezzopane, Tholon and Carpanezzi2016), corn (Moreira et al., Reference Moreira, Gontijo Neto, Lana, Borghi, Santos, Alvarenga and Viana2018), and soybean + corn (Magalhães et al., Reference Magalhães, Pedreira, Tonini and Farias Neto2018) crops, with yield reductions near the trees from the second or third year after the planting of trees. The results obtained for the corn crop in this study are similar to those presented by Silva et al. (Reference Silva, De Moraes, Moletta, Pelissari, Dieckow and Oliveira2015) and Moreira et al. (Reference Moreira, Gontijo Neto, Lana, Borghi, Santos, Alvarenga and Viana2018).
Pasture vegetative characteristics were different between treatments in the iCLF and iCL (Table 2), but with a lower magnitude than those observed by Guenni et al. (Reference Guenni, Seiter and Figueroa2008) and Paciullo et al. (Reference Paciullo, Gomide, Castro, Fernandes, Muller, Pires, Fernandes and Xavier2011). Pasture height was different between iCLF_2 and iCL in the autumn and winter cycles, seasons in which PAR transmission was lower than in the summer and spring cycles (Figure 1b).
As for corn, thinning was beneficial for pasture production, considering there were no differences in forage accumulation between systems. In the spring and summer cycles, pre-grazing forage mass was higher in iCL and at iCLF_3 only in spring. These positions had higher PAR transmission. In these cycles, PAR transmission achieved values close to 50% at the most shaded positions (iCLF_1 and iCLF_4), which indicated excessive shading. Shading higher than 35–40% may decrease tillering, and consequently, reduce pasture yield (Bosi et al., Reference Bosi, Pezzopane, Sentelhas, Santos and Nicodemo2014; Paciullo et al., Reference Paciullo, Gomide, Castro, Fernandes, Muller, Pires, Fernandes and Xavier2011; Pezzopane et al., Reference Pezzopane, Bernardi, Bosi, Oliveira, Marconato, Pedroso and Esteves2019). Under such conditions, grazing must be more lenient to avoid plant death (Paciullo et al., Reference Paciullo, Gomide, Castro, Fernandes, Muller, Pires, Fernandes and Xavier2011).
The nutritive value of the corn for silage and the pasture was higher in the iCLF treatments than in iCL. Corn for silage presented a higher proportion of grains in the total biomass and higher CP content in the iCLF treatments compared with that in iCL. Pasture CP content was also higher in the iCLF positions in the autumn, winter, and spring cycles. Paciullo et al. (Reference Paciullo, Carvalho, Aroeira, Morenz, Lopez and Rossiello2007), Barro et al. (Reference Barro, Saibro, Medeiros, Silva and Varella2008), Pezzopane et al. (Reference Pezzopane, Bernardi, Bosi, Oliveira, Marconato, Pedroso and Esteves2019), and Santos et al. (Reference Santos, Guimarães Junior, Vilela, Maciel and França2018) also observed a higher nutritive value for pastures grown under tree shading than under full sun. In general, these authors stated that higher CP content in shaded plants is caused by higher organic matter mineralization in the soil under shading compared with soil under full sun.
When total aboveground biomass yield was analyzed (Figure 3c), a higher yield was observed in the iCLF system, indicating better land use for this type of integrated system. Agricultural production diversification has been cited as a great advantage of iCLF systems in comparison to monocultures (Figueiredo et al., Reference Figueiredo, Jayasundara, Bordonal, Reis, Wagner-Riddle and La Scala2017; Gil et al., Reference Gil, Siebold and Berger2015). Despite this, competition between the components of iCLF systems may be a limiting factor for their adoption by farmers because of the reduction in pasture productivity and consequent reduction in beef production per area, if corrective management practices, such as thinning of trees, are not adopted.
Several studies in iCLF systems demonstrated the negative effect of adult trees on crop and pasture yields, mainly because of lower light availability for the understory plants and competition for water (Barro et al., Reference Barro, Saibro, Medeiros, Silva and Varella2008; Simão et al., Reference Simão, Gontijo Neto, Oliveira Neto, Galvão, Borghi, Martins and Resende2018). Tree management practices in iCLF systems, such as pruning and thinning, reduce competition for light in these systems, at least for a short time (Nicodemo et al., Reference Nicodemo, Castiglioni, Pezzopane, Tholon and Carpanezzi2016), which contribute to the proper productive balance between the system’s components. This occurred in our study, in which corn for silage and pasture yields were not different between iCLF and iCL systems after thinning.
Conclusions
The thinning was efficient in reducing competition for solar radiation between eucalyptus trees and corn or palisadegrass.
Yields of corn for silage and palisade grass were similar among different positions of the crop–livestock–forest and the crop–livestock system, which indicated that thinning maintained competition within a level that did not decrease understory plant yield. Additionally, the nutritive value of corn for silage and palisadegrass was higher in the crop–livestock–forest positions compared with the crop–livestock system.
Total aboveground biomass yield was higher in the iCLF system, indicating better land use for this type of integrated system.
Acknowledgments
To São Paulo Research Foundation (FAPESP), which funded this study (grant 2016/02959-1) and granted scholarships to the second and third authors (grants 2016/14538-0 and 2014/11931-8, respectively). To Associação Rede ILPF, which funded this study. To National Council for Scientific and Technological Development (CNPq) for the research productivity fellowship granted to the first author.
Supplementary material
To view supplementary material for this article, please visit https://doi.org/10.1017/S0014479720000162