Despite decades of research, documentation, and observations of diagnostic comorbidities in psychiatric syndromes, little is known about the mechanisms underlying this common phenomenon. This is largely a result of past conceptualizations and scientific paradigms, which assumed discrete disorders and static etiologic agents. However, as accumulating research has increasingly highlighted the many risk factors, brain abnormalities, cognitive deficits, and symptoms that are shared across diagnostic entities, these assumptions have been challenged. The prefrontal cortex (PFC) is among the neural regions consistently implicated in the pathogenesis of multiple forms of psychopathology and likely represents an important mechanism of comorbidity among disorders.
Critical shifts in research agendas propelled by the field of developmental psychopathology, and more recently the Research Domain Criteria (RDoC) initiative, have provided new and necessary approaches to understanding comorbidity and psychopathology. A core assumption of the developmental psychopathology perspective is that clinical disorders emerge from unfolding developmental process, and that etiology can only be explained through specification of individual-level vulnerabilities, contextual and environmental risk factors, and their complex interactions over time (Beauchaine & Gatzke-Kopp, Reference Beauchaine and Gatzke-Kopp2012; Rutter, Kim-Cohen, & Maughan, Reference Rutter, Kim-Cohen and Maughan2006). Thus, neither biological vulnerabilities nor environmental factors alone provide sufficient explanations of etiology and must be considered together. Meanwhile, the current RDoC initiative has called for a focus on neurobiological substrates and transdiagnostic processes in research on disorders that have traditionally been considered distinct, and it highlights disruptions in neural circuitry, rather than behavioral symptoms, as fundamental to classification (Insel et al., Reference Insel, Cuthbert, Garvey, Heinssen, Pine and Quinn2010). This initiative also emphasizes a dimensional approach that assumes continuity between normal and abnormal processes, and allows for nonlinear patterns to emerge. While the developmental psychopathology and RDoC approaches have different foci, they are synergistic and enhance research on transdiagnostic processes within an ontogenic perspective. Combining the two provides a promising avenue that assumes that comorbidities do not necessarily simply arise from co-occurrence of distinct disorders, but rather from dynamic changes in the expression of shared vulnerability processes across development. A clearer understanding of the mechanisms underlying comorbidity, discontinuity, and continuity is critical to translational research and intervention efforts.
The purpose of this review is to examine the role of the PFC in comorbidity and continuity from a transdiagnostic and ontogenic perspective. A foundational assumption here is that PFC circuits subserve core functions involved in key mechanisms of vulnerability for psychopathology. Through these various mechanisms, individual differences and impairments in PFC-related functions can then give rise to problematic behavioral manifestations that cut across multiple diagnostic categories. As we describe below, the developmental and functional characteristics of the PFC render this neural substrate pivotal to individual differences that have significant implications for transdiagnostic cognitive, mood, and reward processes. However, PFC vulnerabilities alone are not necessarily pathological and must be considered in relation to environmental and contextual factors across time. Accordingly, we aim to highlight how transactions between the developing PFC and environmental factors can potentiate the expression of vulnerability to psychopathology through interactions that span multiple levels of analysis. It is assumed that genetic factors draft the blueprint for PFC development, but that prenatal factors and postnatal exposures can modify the execution of the blueprint, and result in recursive processes that exacerbate or compensate for adverse developmental processes.
In this review, we focus specifically on PFC developmental characteristics and environmental factors in childhood and adolescence, given that two-thirds of adults with psychiatric syndromes exhibit signs of psychopathology early in life, and that even in later occurring disorders, the etiological pathways are usually established earlier in development (Copeland, Shanahan, Costello, & Angold, Reference Copeland, Shanahan, Costello and Angold2009). Our goals are to (a) review core PFC-mediated functions that subserve shared vulnerability processes across psychopathology, (b) highlight unique aspects of PFC development and function that are vulnerable to disruption, (c) identify common environmental risk processes (e.g., parenting, peers, stress, and substance use) that compromise and compound PFC-related vulnerability, and (d) provide a heuristic and case example illustrating how transactions between PFC-mediated processes, endogenous influences, and exogenous influences can give rise to comorbidity from a transdiagnostic and ontogenic perspective.
PFC as a Mechanism of Comorbidity
While there have been a number of published reports on rates and patterns of comorbidity (e.g., Kessler, Chiu, Demler, & Walters, Reference Kessler, Chiu, Demler and Walters2005), and temporal sequencing of disorders (e.g., Rutter et al., Reference Rutter, Kim-Cohen and Maughan2006), there has been relatively little attention to the mechanisms underlying these phenomena. As noted, this is due in part to current diagnostic systems, which utilize categories based on symptoms, rather than etiology, and may contribute to an illusion of distinct co-occurring disorders. However, accumulating behavioral and genetic research suggests that most forms of psychopathology share common genetic and neural vulnerabilities and are manifestations of a relatively few core underlying processes. These findings support the view that comorbidity, in most cases, arises, not from true co-occurrence of distinct disorders, but from the behavioral expression of shared vulnerability processes across the life span.
There is considerable evidence that the PFC plays a critical role in the pathogenesis of psychopathology and likely represents an important mechanism of comorbidity among disorders. The PFC is a large, anatomically and functionally heterogeneous brain region that occupies 25% of the cerebral cortex and is critical for behavioral adaptation in the human species (Diamond, Reference Diamond, Stuss and Knight2002). The PFC is well positioned for brainwide modulation and coordinated processing of the multimodal inputs needed to guide action in complex tasks, given its extensive connections with motor, motivational, reward, stress, and affective systems. Through reciprocal feedback pathways, the PFC can both regulate and stimulate subcortical and cortical regions, as well as brainstem neuromodulatory systems. In addition, the region demonstrates ongoing plasticity and adaptation to the demands of new tasks, challenges, and experiences.
These important structural and functional aspects of the PFC make it a critical brain region in behavioral maladaptation. Nearly every form of psychopathology involves one or more symptom dimensions that suggest PFC dysfunction, which is not surprising given the region's key role in the regulation of behavior, mood, motivation, and reward. Complex interactions among genetic factors, environmental exposures, and experiences drive the maturation of this region across development, providing multiple pathways for potential disruptions and adverse developmental outcomes. As we will discuss, many of the genetic, neurochemical, and environmental risk factors most commonly implicated across psychiatric disorders confer vulnerability directly or indirectly to the PFC, among other brain regions.
In adopting an ontogenic process perspective and drawing from the model laid out by Beauchaine and McNulty (Reference Beauchaine and McNulty2013), we suggest that comorbidities often arise from neurobiologically rooted vulnerabilities inherent in PFC circuitry, which lead to individual differences in core functional processes, which through transactions with environmental risk factors, promote psychopathology and maladaptive behaviors overtime. This review is not meant to be exhaustive and is necessarily limited in scope. There are, of course, multiple developmental and pathophysiological pathways that inform psychopathology and comorbidity. However, this review provides a starting point for considering neural mechanisms of comorbidity across multiple levels of analysis that incorporate both developmental and dimensional approaches.
Domains of Shared Vulnerability
While often referred to as a single brain region, the PFC contains many subdivisions, each of which possesses specific cytoarchitecture, neurochemistry, connectivity, and functional properties (Miller & Cohen, Reference Miller and Cohen2001; Ramnani & Owen, Reference Ramnani and Owen2004). See Figure 1 for an overview of key anatomical regions. A comprehensive discussion of the anatomical and functional distinctions among these subdivisions is beyond the scope of this paper. Throughout this review, however, we will denote specific PFC regions when such distinctions are possible and relevant.

Figure 1. (Color online) Anatomical divisions of the prefrontal cortex (PFC). The PFC can into be divided into dorsolateral (dlPFC), ventrolateral (vlPFC), medial (mPFC), orbitofrontal (OFC), and anterior (not pictured) cortical regions. Additional medial divisions, such as the ventral medial (vmPFC), are also observed. Different PFC subregions have distinct, yet overlapping connections and functions. For example, the dorsolateral portions are heavily interconnected with sensory and motor cortices and play key roles in regulating attention, thought, and action. Meanwhile, the vmPFC has connections with subcortical structures (e.g., amygdala) and plays a prominent role in regulating emotional responses. The OFC integrates information from sensory and subcortical regions to help guide emotional and reward-based decision making. The amygdala (Amyg), striatum, and anterior cingulate (ACC) are included here because they represent key nodes in the frontal circuits discussed throughout this review.
In this section, we highlight three PFC-mediated functions that we propose to underlie shared vulnerability across multiple forms of psychopathology, and thereby play pivotal roles in comorbid syndrome expressions. These are executive, emotion regulation, and reward-processing functions. As we will discuss throughout this review, there is considerable evidence that genetic, hormonal, and environmental factors frequently implicated in psychopathology converge on these core functions. For each function, we begin with a brief description of normal development to provide a reference frame from which we can contextualize deviations and understand both how individual differences arise over the course of development and why certain deviations confer vulnerability for psychopathology. We then highlight the functional consequences of individual differences and how they relate to transdiagnostic symptoms. These functional processes are of considerable interest not only because they provide mechanisms for transdiagnostic symptoms but also because they underlie behavioral tendencies that are likely to elicit and interact with environmental risk factors to further potentiate vulnerability. It is important to note that each of these functional domains is a complex, multidimensional construct that is influenced by multiple neural and biological processes, many of which are not reviewed here. However, given the pivotal role of the PFC in circuits subserving these functions, it is highly likely that PFC vulnerabilities will be manifested in all of these domains.
Executive function (EF) and dysfunction
Broadly defined, EFs encompass a set to cognitive processes orchestrated by activity within the PFC that underlie goal-directed behavior (see Diamond, Reference Diamond2013, for review). The coordination of these processes underlie multiple aspects of everyday functioning critical to behavioral adaptation, including self-regulation, decision making, sequencing actions, planning, prioritizing, and navigating new tasks (Banich, Reference Banich2009; Snyder, Miyake, & Hankin, Reference Snyder, Miyake and Hankin2015). While often referred to as a unitary construct in the literature, factor analytic approaches suggest that EFs are composed of a number of related, but at least partially distinct, processes (Miyake & Friedman, Reference Miyake and Friedman2012). The literature most consistently points to three core EFs, which include inhibition (including self-control and interference control), working memory, and cognitive flexibility (also called shifting; Best & Miller, Reference Best and Miller2010; Diamond, Reference Diamond2013; Prencipe et al., Reference Prencipe, Kesek, Cohen, Lamm, Lewis and Zelazo2011). These foundational EFs provide the building blocks for higher order EFs such as problem solving, reasoning, and planning (Lunt et al., Reference Lunt, Bramham, Morris, Bullock, Selway and Xenitidis2012).
Although the developmental trajectories of various EF components vary in pacing, findings have generally supported a linear progression of EF abilities throughout development. Rudimentary abilities emerge within the first years of life, rapidly improve in early childhood, and continue to develop during adolescence (for a review, see Best & Miller, Reference Best and Miller2010). Recent work suggests that EF development is characterized by both quantitative and qualitative changes, which may reflect PFC maturational processes and reorganization, respectively (Best & Miller, Reference Best and Miller2010). For example, increased functional development of EFs has been found to parallel PFC gray matter decline during middle childhood and adolescence (Huizinga, Dolan, & van der Molen, Reference Huizinga, Dolan and van der Molen2006; Luna, Garver, Urban, Lazar, & Sweeney, Reference Luna, Garver, Urban, Lazar and Sweeney2004) and is hypothesized to result from the neuromaturational processes of synaptic pruning and myelination. Consistent with this hypothesis, Tamnes et al. (Reference Tamnes, Walhovd, Grydeland, Holland, Østby and Dale2013) found that improvements in working memory were related to reductions in prefrontal and parietal cortical volumes between 9 and 20 years of age. At the same time, improvements in EF performance are also associated with age-related changes in activation patterns (both increases and decreases depending on PFC region), which may reflect increased neural efficiency and reorganization of the developing circuits (Durston et al., Reference Durston, Davidson, Tottenham, Galvan, Spicer and Fossella2006). Best and Miller (Reference Best and Miller2010) have also suggested that the development of metacognition may facilitate qualitative changes in EFs, as children are able reflect on their errors and deliberately change their approach. Taken together, these structural and functional developmental patterns suggest that EF improvements depend, in part, on increased maturation and neural efficiency of the PFC across development.
The development of core EFs (e.g., working memory, inhibition, and cognitive flexibility) provides the foundation for more complex, later developing functions related to problem solving, decision making, and emotion regulation (Best & Miller, Reference Best and Miller2010; Wiebe et al., Reference Wiebe, Sheffield, Nelson, Clark, Chevalier and Espy2011). As a result, youth who experience delayed or compromised development of basic EF processes are likely to experience continued (and compounded) difficulties across development as the demands for behavioral regulation increase and developmental tasks become more challenging (e.g., school entry and peer relationships). EFs have been associated with a range of long-term outcomes related to academic performance (Borella, Carretti, Riboldi, & De Beni, Reference Borella, Carretti, Riboldi and De Beni2010), job success (Bailey, Reference Bailey2007), criminal activity (Broidy et al., Reference Broidy, Tremblay, Brame, Fergusson, Horwood and Laird2003), and relationships (Eakin et al., Reference Eakin, Minde, Hechtman, Ochs, Krane and Bouffard2004).
Weaknesses in executive functioning have been well documented in both externalizing and internalizing youth (Eysenck, Derakshan, Santos, & Calvo, Reference Eysenck, Derakshan, Santos and Calvo2007; Snyder et al., Reference Snyder, Miyake and Hankin2015) and provide mechanisms for transdiagnostic symptom expression. For example, weaknesses in core EFs make it more challenging to control attention toward goal-relevant information (Diamond, Reference Diamond2013), shift attention away from threatening or emotional stimuli (Drabrick, Ollendick, & Bubier, Reference Drabick, Ollendick and Bubier2010), shield goals from interference (Hofmann, Schmeichel, & Baddeley, Reference Hofmann, Schmeichel and Baddeley2012), control behavioral impulses, and suppress ruminative or repetitive thoughts (Demeyer, De Lissnyder, Koster, & De Raedt, Reference Demeyer, De Lissnyder, Koster and De Raedt2012). While these consequences are not inevitably dysfunctional in a clinical sense, they have been linked to a number of maladaptive behaviors commonly observed in both internalizing and externalizing disorders, such as rumination (Demeyer et al., Reference Demeyer, De Lissnyder, Koster and De Raedt2012; Zetsche, D'Avanzato, & Joormann, Reference Zetsche, D'Avanzato and Joormann2012), worry (Snyder et al., Reference Snyder, Kaiser, Whisman, Turner, Guild and Munakata2014), impulsivity (Fino et al., Reference Fino, Melogno, Iliceto, D'Aliesio, Pinto and Candilera2014), aggression (Séguin & Zelazo, Reference Séguin, Zelazo, Archer, Tremblay, Hartup and Willard2005), and poor use of adaptive emotion regulation strategies (Andreotti et al., Reference Andreotti, Thigpen, Dunn, Watson, Potts and Reising2013). In addition to subserving symptoms, executive dysfunction is also associated with adverse environmental risk processes such as peer rejection (Razza & Blair, Reference Razza and Blair2009) and academic difficulties (Valiente et al., Reference Valiente, Eisenberg, Spinrad, Haugen, Thompson and Kupfer2013; Blair & Razza, Reference Blair and Razza2007).
Emotion regulation and dysregulation
Although there is considerable debate about the construct, emotion regulation is generally viewed as a suite of attentional, cognitive, and behavioral operations that modify emotional experience and expression (Lewis, Todd, & Xu, Reference Lewis, Todd, Xu, Lamb, Freund and Lerner2010). These modifications are modulated, in part, by the PFC and can include increasing (or decreasing) the intensity of emotional feeling states, inhibiting (or disinhibiting) the behavior that flows from emotions, and changing the form or content of the cognitive activities that correspond to various emotional states (Gross & Thompson, Reference Gross, Thompson and Gross2007; Lewis, Reference Lewis and Zelazo2013). Across development, there are significant changes in emotion regulation abilities and strategies characterized by a shift from reliance on external supports to self-initiated efforts (for review see Thompson & Goodman, Reference Thompson, Goodman, Barbarin and Wasik2009). While caregivers initially help regulate infant's emotions, youth begin to manage their own emotions with more behavioral strategies (e.g., help seeking and avoiding events) in childhood and with more cognitively based strategies in adolescence.
Improvements in emotion regulation are attributable, in part, to maturation and engagement of the PFC in regulatory processes across development (Ahmed, Bittencourt-Hewitt, & Sebastian, Reference Ahmed, Bittencourt-Hewitt and Sebastian2015). Perlman and Pelphrey (Reference Perlman and Pelphrey2011) have found that younger children recruit more ventral PFC areas when required to regulate emotions, while older children engage more dorsal areas. Dorsal regions of the PFC are involved in mediating smooth, deliberate control of emotion in a supervisory fashion, while ventral regions control impulses more rigidly, in anticipation of negative consequences (Lewis, Granic, & Lamm, Reference Lewis, Granic and Lamm2006). This shift from ventral to dorsal PFC engagement and concomitant maturation of EFs likely enhances emotional control and enables youth to respond in less impulsive and more strategic ways. In addition, the ability to evaluate emotional experiences via self-monitoring and cognitive appraisal that accompanies cognitive advances in capacities for abstract, reflective, and hypothetical thinking (Steinberg, Reference Steinberg2005) provide the basis for new cognitively based emotion regulation strategies (e.g., problem solving, information seeking, cognitive restructuring, acceptance, and catastrophizing). Consistent with this, a longitudinal study by Gullone, Hughes, King, and Tonge (Reference Gullone, Hughes, King and Tonge2010) found that youth between 9 and 15 years of age showed increases in their use of cognitive appraisal strategies and decreases in suppression strategies with age. A cross-sectional study of 10- to 22-year-olds found a linear, age-related increase in dorsolateral PFC (dlPFC) activation during a cognitive reappraisal task (McRae et al., Reference McRae, Gross, Weber, Robertson, Sokol-Hessner and Ray2012). Taken together, evidence suggests that reappraisal-related engagement of cognitive control processes increases linearly with age from late childhood to early adulthood.
Despite these modal developmental advances, there is a considerable amount of individual difference in emotion regulation. Given that emotion regulation is executed through reciprocal interactions between multiple regions of the PFC, limbic, and neurohormonal systems governing emotional arousal, individual differences can result from variations in any one of these component processes. For example, individual differences in emotion dynamics (e.g., speed of onset, persistence, magnitude, and lability) driven by early-emerging biases and/or heritable traits (e.g., trait anxiety) in subcortical regions can have long-standing influences on emotional regulatory processes, regardless of PFC maturation (Heller & Casey, Reference Heller and Casey2016). Cognitive appraisals and regulatory styles are also likely to differ widely among youth as a function of their vulnerability to certain emotions (e.g., anxious distress and sad affect), temperamental styles, and prior experiences with heightened emotions (Thompson & Goodman, Reference Thompson, Goodman, Barbarin and Wasik2009). In addition, researchers have suggested that emotion regulation is socialized through early parenting dynamics, providing an important opportunity for environmental influences to instantiate individual differences (Beauchaine, Reference Beauchaine2015; Goldsmith, Pollak, & Davidson, Reference Goldsmith, Pollak and Davidson2008).
Weaknesses in emotion regulation, which can result in mood states of abnormal intensity and duration, poorly controlled shifts in emotion, dysfunctional appraisal of emotion-eliciting situations, hypersensitivity to anticipatory emotional arousal, and inappropriate expression of emotions (Heller & Casey, Reference Heller and Casey2016; Zeman, Cassano, Perry-Parrish, & Stegall, Reference Zeman, Cassano, Perry-Parrish and Stegall2006), have been implicated in nearly all forms of psychopathology. Again, while these individual differences are not inherently dysfunctional, intense or prolonged states of anger, sadness, and fear are associated with greater irritability (Deveney et al., Reference Deveney, Connolly, Haring, Bones, Reynolds and Kim2013), negative affect (Gross & John, Reference Gross and John2003), anxiety (Buss et al., Reference Buss, Davis, Kiel, Brooker, Beekman and Early2013), and aggression (Gilliom, Shaw, Beck, Schonberg, & Lukon, Reference Gilliom, Shaw, Beck, Schonberg and Lukon2002). Youth who experience more intense and/or prolonged negative mood states are also more likely to resort to maladaptive avoidance coping strategies, such as worry, rumination, and/or aggression, to reduce the distress of the emotional arousal (Zeman et al., Reference Zeman, Cassano, Perry-Parrish and Stegall2006). While these strategies provide temporary relief, they can set the stage for enduring patterns of emotional dysregulation and maladaptive behaviors. Furthermore, youth who demonstrate poor emotion regulation are more likely to experience peer rejection (Kim & Cicchetti, Reference Kim and Cicchetti2010), which further enhances risk for psychopathology.
Reward processing
Reward-related processes encompass a set functions related to reward valuation, cost/benefit analysis, and reward based decision making (Der-Avakian & Markou, Reference Der-Avakian and Markou2012; Rogers et al., Reference Rogers, Owen, Middleton, Williams, Pickard and Sahakian1999, Reference Rogers, Ramnani, Mackay, Wilson, Jezzard and Carter2004). These functions are orchestrated by interactions between the striatum and the PFC, with different subregions participating in distinct yet coordinated aspects of reward processing. For example, the orbitofrontal cortex (OFC) and anterior cingulate cortex provide “calculations” of the relative reward value and effort required to pursue a goal, which are then integrated by the ventromedial PFC (vmPFC) and dlPFC to guide decision-making and goal-directed behavior (Grabenhorst & Rolls, Reference Grabenhorst and Rolls2011). Like the functions discussed above, reward is not a unitary construct, and a nuanced pattern of reward-processing differences (rather than global differences) likely underlies the continuum of reward-related behaviors.
Compared to other domains, considerably less is known about the developmental course of reward-related processes. Developmental neuroimaging studies have shown that children, adolescents, and adults recruit the same neural circuitry (i.e., PFC and striatum) when presented with rewards (e.g., Van Leijenhorst et al., Reference Van Leijenhorst, Moor, de Macks, Rombouts, Westenberg and Crone2010), although there are age-related differences in activation. For example, the activation of the PFC in reward-based tasks is more diffuse in youth, as compared to adults (Galvan et al., Reference Galvan, Hare, Parra, Penn, Voss and Glover2006). In addition, studies have found that compared to adults, adolescents show weaker dlPFC response to monetary reward (Ernst et al., Reference Ernst, Nelson, Jazbec, McClure, Monk and Leibenluft2005) and lower activation of the ventrolateral PFC when making risky decisions that could lead to a large reward (Eshel, Nelson, Blair, Pine, & Ernst, Reference Eshel, Nelson, Blair, Pine and Ernst2007). This pattern of finding suggests reduced and less efficient PFC engagement in reward-related processes earlier in development. The most striking developmental change in reward processes occurs during adolescence, when a transient increase in reward-seeking behaviors emerges. A number of studies posit that this normative increase in reward sensitivity is driven by hyperactivity of the striatum and changes in the dopamine (DA) system that subsequently stabilize in adulthood, in conjunction with PFC maturation (Casey, Jones, & Hare, Reference Casey, Jones and Hare2008; Somerville, Jones, & Casey, Reference Somerville, Jones and Casey2010; Steinberg, Reference Steinberg2008). This is consistent with the notion that protracted maturation of PFC regulatory abilities results in poor inhibitory control of hyperresponsive reward systems, among other subcortical systems (Spear, Reference Spear2000).
Individual differences in reward function have the potential to influence a wide range of reward-related processes, including hedonic capacity, calculations of reward valuations and required effort, motivation to pursue rewards, enjoyment of rewarding outcomes, and decision making (Forbes & Dahl, Reference Forbes and Dahl2005). Accordingly, altered reward responding has been found to increase vulnerability to anhedonia (Der-Avakian & Markou, Reference Der-Avakian and Markou2012; Forbes & Dahl, Reference Forbes and Dahl2012), avolition (Berridge, Robinson, & Aldridge, Reference Berridge, Robinson and Aldridge2009), irritability (Deveney et al., Reference Deveney, Connolly, Haring, Bones, Reynolds and Kim2013), and low positive affect (Forbes et al., Reference Forbes, Hariri, Martin, Silk, Moyles and Fisher2009), which are frequently observed in both depression and externalizing syndromes. It has been hypothesized that blunted reward responding often leads to chronically aversive, irritable mood states, which subsequently promote escape, avoidance, or immediate reward seeking as a way to elevate mood state (Laakso et al., Reference Laakso, Wallius, Kajander, Bergman, Eskola and Solin2003). The resulting compensatory behaviors can range from extreme avoidance (e.g., anhedonia) to excessive approach (e.g., impulsivity). Inaccurate reward predication, another manifestation of altered reward processes, has also been associated with poor decision making (Cohen et al., Reference Cohen, Asarnow, Sabb, Bilder, Bookheimer and Knowlton2010), impulsivity, and increased frustration, as well as reduced approach behaviors (Gradin et al., Reference Gradin, Kumar, Waiter, Ahearn, Stickle and Milders2011).
The PFC-mediated functions outlined above have the potential to underlie a host of transdiagnostic symptoms. Presumably, alterations in these functional domains can occur alone or in complex combinations, and in varying magnitudes, which will ultimately determine the behavioral expression. These PFC-mediated functions are multifactorially determined processes that are shaped by interactions among multiple genetic and environmental factors. While individual differences may be initially conferred by genetic, epigenetic, prenatal, and/or maternal programming effects, these functions are continuously shaped across development through bidirectional transactions with environmental and contextual factors. Consistent with the principle of equifinality, there are multiple pathways through which individual differences in these fundamental processes can arise to produce similar behavioral outcomes. As we will review below, these PFC-mediated domains of function may constitute intermediate mechanisms that can account for the relationship between more distal genetic and environmental risk processes, the combination of which is a common theme in clinical phenomena.
A central assumption here is that the pivotal role of the PFC in the transdiagnostic expression of psychopathology is best understood through multiple levels of analysis. In the following, we begin by identifying biological factors and processes that confer vulnerability to the PFC, followed by environmental and social contextual factors that interact with and modulate PFC vulnerabilities. Throughout these sections, we will refer to typical trajectories of maturation, which can be contrasted with the developmental deviations that give rise to psychopathology. While we address biological and environmental factors separately here, it is assumed that transactional processes across these levels of analysis ultimately determine the developmental trajectory.
PFC Development: Neurobiological Influences on Vulnerability
Research suggests that the functional capacities of the PFC are highly influenced by the region's maturational course (Casey et al., Reference Casey, Jones, Levita, Libby, Pattwell and Ruberry2010; Yurgelun-Todd, Reference Yurgelun-Todd2007), integrity of cortical–subcortical circuitry (Arnsten & Rubia, Reference Arnsten and Rubia2012), neurochemical milieu (Arnsten & Li, Reference Arnsten and Li2005), and genetic factors (Buckholtz & Meyer-Lindenberg, Reference Buckholtz and Meyer-Lindenberg2012). Disruptions in any of these processes are likely to have significant and widespread functional consequences.
Structural development
While a comprehensive review of PFC neurodevelopment is not intended here (see Diamond, Reference Diamond, Stuss and Knight2002; Stuss & Knight, Reference Stuss and Knight2013), we will describe several important developmental characteristics that demonstrate the unique vulnerability of the region to maturational disruption.
PFC pre- and perinatal development is highly sensitive to perturbations
During the prenatal period, developing cortical neurons are sensitive to a range of endogenous and exogenous factors, including prenatal drug exposures (e.g., cannabis, nicotine, and cocaine) and maternal stress, which have been found to impact cortical morphology (El Marroun et al., Reference El Marroun, Tiemeier, Franken, Jaddoe, van der Lugt and Verhulst2015; Weinstock, Reference Weinstock2008) and disrupt connectivity in frontal circuitry (Salzwedel et al., Reference Salzwedel, Grewen, Vachet, Gerig, Lin and Gao2015) during the postnatal period. In addition, the PFC undergoes a wave of neurogenesis and neural migration during late gestation, coinciding with peak perinatal vulnerabilities related to in utero complications, premature birth, and delivery complications (e.g., fetal distress and hypoxia).
The PFC's protracted developmental course provides a wide window for environmental factors to influence development
Like some other cortical gray matter regions, PFC structural development is characterized by an inverted U-shaped volume by age trajectory, such that volume generally increases during childhood, peaks in middle adolescence, and then declines into adulthood (Giedd, Reference Giedd2004; Lenroot et al., Reference Lenroot, Gogtay, Greenstein, Wells, Wallace and Clasen2007). Although research cannot precisely characterize the molecular basis of such changes in humans, the volumetric changes are hypothesized to reflect an initial overproduction of dendritic spines and proliferation of neural circuits, followed by the initiation and gradual escalation of synaptic pruning in middle adolescence that serves to enhance neural efficiency (Lenroot & Giedd, Reference Lenroot and Giedd2006). In contrast to gray matter, white matter development follows a more linear increase, during childhood and adolescence, which in conjunction with pruning processes increases the efficiency and stability of firing patterns in the PFC (Hagmann et al., Reference Hagmann, Sporns, Madan, Cammoun, Pienaar and Wedeen2010).
At the cellular level, when compared to other cortical regions, the PFC shows a pronounced plateau through childhood, followed by a gradual decrease in synapses during adolescence and young adulthood (Lenroot & Giedd, Reference Lenroot and Giedd2006). The PFC has one of the longest periods of developmental maturation of any brain region, and it is generally one of the last to complete neuronal/synaptic formation and elimination (Casey et al., Reference Casey, Jones, Levita, Libby, Pattwell and Ruberry2010; Elston, Oga, & Fujita, Reference Elston, Oga and Fujita2009; Luciana, Reference Luciana2013). It is generally assumed that brain regions that are more plastic over prolonged developmental periods are more susceptible to environmental factors (e.g., drugs, stress, social experiences, and hormones), which can alter the developmental course (Kolb & Gibb, Reference Kolb and Gibb2011). Thus, the protracted maturational course of the PFC broadens its window of vulnerability to environmental perturbations and enhances the likelihood it will play a role in deviations from the modal course of behavioral development.
The timing, rate, and slope of neuromaturational changes have significant implications for functioning
The pacing of PFC maturation appears to be particularly important to functioning, and volume changes are likely reflections of important neuromaturational processes (e.g., synaptic pruning and myelination). The timing of the onset and completion of these cellular processes appears to have measurable functional consequences. Longitudinal studies increasingly suggest that the developmental trajectory of the PFC, and most other brain regions, can be more predictive of outcome than its absolute volume and/or thickness. For example, in a cohort-sequential study design, adolescents with superior intellectual abilities exhibited greater peak thickness around puberty, followed by a greater cortical thinning into adulthood (Shaw et al., Reference Shaw, Greenstein, Lerch, Clasen, Lenroot and Gogtay2006). This is consistent with more recent research that has identified a positive relationship between the pace of prefrontal cortical thinning and cognitive control abilities (Tamnes et al., Reference Tamnes, Østby, Fjell, Westlye, Due-Tønnessen and Walhovd2010, Reference Tamnes, Walhovd, Grydeland, Holland, Østby and Dale2013), as well as emotion regulation capacities (Vijayakumar et al., Reference Vijayakumar, Whittle, Yücel, Dennison, Simmons and Allen2014). When these maturational processes are off pace, as indexed by morphological features by chronological age, adverse functional consequences are more likely. For example, when compared to controls, children with attention-deficit/hyperactivity disorder (ADHD) show a considerable lag in the neurodevelopment of the medial PFC (Shaw et al., Reference Shaw, Malek, Watson, Sharp, Evans and Greenstein2012), and among boys with conduct disorder the typical reductions in PFC gray matter are not observed (De Brito et al., Reference De Brito, Mechelli, Wilke, Laurens, Jones and Barker2009). Accordingly, interpretations of individual or group differences in PFC volume must be appropriately contextualized, because volume differences resulting from regressive changes (e.g., atrophy) and progressive changes (e.g., neuron proliferation and synaptogenesis) can have different functional implications depending on the developmental stage.
The developmental trajectory of the PFC in relation to other brain regions creates shifting balances in key circuits
Across brain regions, development occurs in a heterogeneous fashion, with regions varying in the onset and pace of development, and this has been posited to result in shifting balances in the relative influence of various regions and circuits on behavior. Even within the PFC, subregions differ in their maturational course, with gray matter volumes reaching adult levels earliest in the OFC, followed by the ventrolateral PFC, and the dlPFC (Walhovd, Tamnes, & Fjell, Reference Walhovd, Tamnes and Fjell2014). PFC maturation lags behind midbrain, limbic, and striatal regions, such that the disparity between the developmental trajectories among these regions is at its peak during adolescence (Mills, Goddings, Clasen, Giedd, & Blackmore, Reference Mills, Goddings, Clasen, Giedd and Blakemore2014). This “developmental mismatch” is hypothesized to have transient functional consequences, reflected in normative adolescent changes in mood and reward tendencies, as well as the expression or amplification of vulnerabilities for certain psychopathologies (Casey et al., Reference Casey, Jones and Hare2008; Van Leijenhorst et al., Reference Van Leijenhorst, Moor, de Macks, Rombouts, Westenberg and Crone2010). Thus, development of the PFC should always be contextualized within the whole brain, because its functional expression and maturational course is intimately tied to other brain regions.
Functional connectivity
Functional domains of the PFC rely on the development of distinct yet interconnected sets of anatomically distributed cortical and subcortical regions. The development of cortical/subcortical circuits is a complex process that is influenced by genetic predispositions, environmental factors, and neuroplastic responses to experiential demand (Tau & Peterson, 2010). PFC connections are organized in a topographical manner, such that regions regulating emotion are situated ventrally and medially (e.g., vmPFC) and have extensive connections with subcortical structures (e.g., amgydala, nucleus accumbens, and hypothalamus) that subserve emotional responses. Meanwhile, regions regulating thought, action, and attention are situated more dorsally and laterally (e.g., dlPFC), and have extensive connection with sensory and motor cortices (Casey et al., Reference Casey, Jones and Hare2008). The vmPFC and dlPFC regions also interconnect extensively with one another to coordinate higher order decision making and planning. As a result, the PFC is anatomically positioned to serve as a key substrate for the brainwide integration and regulation. While the PFC is often conceptualized as playing a top-down regulatory role in related circuits, this characterization is overly simplistic. Interactions between the regions that comprise circuits are dynamic, complex, and reciprocal in nature. Thus, although the PFC exerts top-down control over subcortical activity, it can also signal subcortical structures to influence response firing.
In the current review, we limit our focus to the corticolimbic and frontostriatal circuits, which have been subject to extensive investigation in both healthy and clinical populations. Disturbances in these circuits may contribute co-occurring deficits in controlling emotions, thoughts, and behaviors across seemingly disparate disorders and likely underlie individual differences in executive functioning, emotion regulation, and reward processing.
Corticolimbic circuit
The corticolimbic circuit is composed of the amygdala, medial PFC, and lateral PFC, which act in concert to regulate affect, arousal, and vigilance to positive and negative valenced stimuli (Kim et al., Reference Kim, Loucks, Palmer, Brown, Solomon and Marchante2011). Neuroimaging studies using diffusion tensor imaging and functional magnetic resonance imaging (fMRI) have consistently documented both structural and functional connectivity between these regions consistent with reciprocal connections, in which the PFC frequently exerts top-down inhibitory control of amygdalar activity and reactivity (Kim et al., Reference Kim, Loucks, Palmer, Brown, Solomon and Marchante2011; Swartz & Monk, Reference Swartz, Monk, Anderson and Pine2014).
Developmental research findings suggest that maturation of the corticolimbic circuit underlies the changes in emotional reactivity and regulation observed throughout childhood and adolescence (e.g., Nelson, Lau, & Jarcho, Reference Nelson, Lau and Jarcho2014; Silvers et al., Reference Silvers, McRae, Gabrieli, Gross, Remy and Ochsner2012; Tottenham, Hare, & Casey, Reference Tottenham, Hare and Casey2011). Earlier in development, subcortical circuitry appears to dominate emotional responses, as evidenced by heightened magnitudes of activity in subcortical regions in response to emotional cues (e.g., Gee et al., Reference Gee, Humphreys, Flannery, Goff, Telzer and Shapiro2013). As individuals continue through development, this subcortical dominance is increasingly modulated by PFC regulatory engagement, which is associated with enhanced capacities to incorporate emotional information into appropriate action (Heller & Casey, Reference Heller and Casey2016). To date, only a few studies have looked at developmental changes in functional connectivity within the corticolimbic circuit. In a cross-sectional fMRI study spanning ages 4 to 22, Gee et al. (Reference Gee, Humphreys, Flannery, Goff, Telzer and Shapiro2013) found a shift from positive (i.e., cofiring) to negative prefrontal–amygdalar connectivity during the transition to adolescence. This connectivity shift was inferred from functional connectivity, which suggests an increasingly reciprocal relationship between the PFC and amygdala, consistent with more efficient top-down modulation. Further, the shift in functional connectivity was paralleled by a steady decline in amygdala reactivity.
There is some evidence from tract-tracing studies conducted on rodents that amygdala to PFC projections emerge earlier than PFC to amygdala projections (Bouwmeester, Smits, & Van Ree, Reference Bouwmeester, Smits and Van Ree2002), and that this early bottom-up signaling may facilitate connectivity and augment development of connections between these regions. This is consistent with findings at the whole brain level that increased positive connectivity early in life may be necessary to establish a connection that later evolves into a regulatory circuit (Gee et al., Reference Gee, Humphreys, Flannery, Goff, Telzer and Shapiro2013). This also raises the important possibility that early developmental patterns, which are often viewed as “immature” (e.g., immature PFC regulatory capacities), may actually be instrumental in establishing healthy neural functioning later in development. Disruptions or differences in subcortical reactivity early in life will likely have significant consequences for the later developing PFC and emotion regulation processes. As circuitry becomes fine-tuned, repeated activation patterns in this network as a result of specific early experiences are likely to become stronger and more automatic.
Variations in corticolimbic circuit functioning appear to be associated with individual differences in threat detection, affective responsiveness, emotion regulation, and fear extinction (Swarz & Monk, Reference Swartz, Monk, Anderson and Pine2014), as well as the magnitude, duration, and habituation of emotional experiences (Heller & Casey, Reference Heller and Casey2016). At the more extreme ranges, these differences can subserve symptoms of irritability, aggression (Coccaro, McCloskey, Fitzgerald, & Phan, Reference Coccaro, McCloskey, Fitzgerald and Phan2007; Buckholtz & Meyer-Lindenberg, Reference Buckholtz and Meyer-Lindenberg2008; Hoptman et al., Reference Hoptman, D'Angelo, Catalano, Mauro, Shehzad and Kelly2010), anxiety (e.g., Monk et al., Reference Monk, Telzer, Mogg, Bradley, Mai and Louro2008), and emotional lability (see Churchwell, Morris, Heurtelou, & Kesner, Reference Churchwell, Morris, Heurtelou and Kesner2009).
Frontostriatal circuit
The frontostriatal circuit is composed of the lateral PFC, ventromedial PFC, OFC, amygdala, and striatum. Across various tasks, it has been consistently shown that interactions between the PFC and striatum are central to accomplishing goal-directed behavioral regulation (Belleine, Delgado, & Hikoshaka, Reference Belleine, Delgado and Hikosaka2007), suppressing impulses (Miller & Cohen, Reference Miller and Cohen2001), regulating of positive affect (Heller et al., Reference Heller, Johnstone, Shackman, Light, Peterson and Kolden2009), and integrating reward association with behavioral outputs (Pasupathy & Miller, Reference Pasupathy and Miller2005). Current neuroanatomical models emphasize important functional distinctions between the dorsal and ventral portions of the circuit. The dorsal portion, which includes reciprocal connections between the dorsal striatum (caudate nucleus and putamen) and OFC, mediates habit-and stimulus-response learning (Packard & Knowlton, Reference Packard and Knowlton2002), supporting self-regulatory functions, movement, and behavioral control. Meanwhile, the ventral portion, which includes connections between the ventral striatum (nucleus accumbens and olfactory tubercle) and prefrontal regions (anterior cingulate cortex and vmPFC) mediates reward, drive, and motivation, supporting reward-processing functions (Haber & Knutson, Reference Haber and Knutson2010). As a result, different components of the frontostriatal network play key roles in self-regulation, reward processing, and positive affect regulation.
Findings from fMRI studies indicate that functional changes within the dorsal frontostriatal circuits in healthy youth underlie age-related improvements in the capacity for self-regulation, cognitive control, and inhibitory control (Marsh, Maia, & Peterson, Reference Marsh, Maia and Peterson2009; Marsh et al., Reference Marsh, Zhu, Schultz, Quackenbush, Royal and Skudlarski2006; Rubia et al., Reference Rubia, Smith, Woolley, Nosarti, Heyman and Taylor2006). When compared to adults, children recruit distinct and often larger, more diffuse frontostriatal regions when performing cognitive control tasks (Casey, Tottenham, & Fossella, Reference Casey, Tottenham and Fossella2002; Luna et al., Reference Luna, Thulborn, Munoz, Merriam, Garver and Minshew2001; Bunge, Dudukovic, Thomason, Vaidya, & Gabrieli, Reference Bunge, Dudukovic, Thomason, Vaidya and Gabrieli2002), which is hypothesized to reflect greater effort to regulate subcortical structures. Developmental fMRI studies of reward processing suggest that adolescents differ from adults in the balance between PFC activity versus the motivation/emotional responses of risk and reward based in the striatum and amygdala (Casey et al., Reference Casey, Jones and Hare2008; Ernst & Fudge, Reference Ernst and Fudge2009). For example, fMRI studies of incentive processing have found that adolescents typically show greater ventral striatal activation and weaker prefrontal activation in comparison to adults (Bjork, Smith, Chen, & Hommer, Reference Bjork, Smith, Chen and Hommer2010; Cauffman et al., Reference Cauffman, Shulman, Steinberg, Claus, Banich and Graham2010; Padmanabhan, Geier, Ordaz, Teslovich, & Luna, Reference Padmanabhan, Geier, Ordaz, Teslovich and Luna2011; van Leijenhorst et al., Reference Van Leijenhorst, Moor, de Macks, Rombouts, Westenberg and Crone2010). It appears that the resulting imbalance between reward load and cognitive control, and the limited ability of PFC resources to sufficiently regulate reward responses, during adolescence may lead to a transient increase in reward-related behaviors during adolescence. This normative increase in striatal reactivity to reward during adolescence is likely to be particularly problematic in individuals with compromised PFC resources. In addition to top-down regulation of striatal activity, human and animal work also suggests that the PFC can activate the striatum and modulate reward-related firing in the striatum in a top-down manner (Heller et al., Reference Heller, Johnstone, Shackman, Light, Peterson and Kolden2009). Thus, the PFC can both up- and downregulate activity in the striatum.
Variations in frontostriatal development can manifest as individual differences in behavioral flexibility, reinforcement learning, decision making, approach motivation, hedonic responses, positive affect regulation, and salience attribution (Kehagia, Murray, & Robbins, Reference Kehagia, Murray and Robbins2010). At the ends of the distributions of these dimensions, individual differences can manifest as symptoms of impulsivity, compulsivity, and hedonic capacity (e g., anhedonia) that likely underpin atypical approach and avoidance behaviors observed across internalizing and externalizing disorders. For example, it has been hypothesized that reduced reward salience provokes a compensatory increase in reward-seeking behavior (Robbins & Everitt, Reference Robbins and Everitt1999), which in combination with reduced executive functioning abilities results in impulsivity (Marsh et al., Reference Marsh, Maia and Peterson2009). In contrast, anhedonia and depressive symptoms have been associated with patterns of reduced striatal activation in combination with heightened mPFC activation (Forbes & Dahl, Reference Forbes and Dahl2012). This pattern may reflect blunted reward sensitivity (Martin-Soelch, Reference Martin-Soelch2009) and/or mPFC overregulation that dampens sustained engagement of positive affect and reward responsivity (Feldman, Joorman, & Johnson, Reference Feldman, Joormann and Johnson2008), as well as increasing engagement in excessive self-processing (e.g., negative, self-focused rumination; Forbes & Dahl, Reference Forbes and Dahl2012). Meanwhile, symptoms of compulsivity, commonly observed in obsessive–compulsive disorder, substance use, and eating disorders, have been associated with hyperactivity of dorsal frontostriatal circuits in which perseverative behaviors and habits are maintained by maladaptive stimulus-response learning (Marsh et al., Reference Marsh, Maia and Peterson2009).
Of course, dysfunction within these circuits does not necessarily stem solely from the PFC. Variations in the activity of limbic and striatal regions may similarly compromise the developing circuit and overwhelm PFC resources. However, independent of the initial locus of disruption, the functional consequences at the circuit level can be similar. Perturbing connectivity in either the corticolimbic or the frontostriatal circuits would likely disrupt a range of mood, reward, and regulatory processes that are not disorder specific, thus increasing susceptibility to multiple clinical disorders.
Neurotransmitter and hormonal influences
The neurochemical environment of the PFC plays a critical role in driving cellular maturation processes, supporting cognitive functions, and modulating functional connectivity with other brain regions. The PFC has both direct and indirect connections to key neuromodulatory centers (e.g., dopaminergic nuclei from VTA; serotonergic nuclei from raphe nuclei) and through autoregulatory feedback mechanisms plays a critical role in regulating its own neurochemical milieu (Björklund & Dunnett, Reference Björklund and Dunnett2007; Puig & Gulledge, Reference Puig and Gulledge2011). Thus, disruptions in the PFC can result in altered neurochemical levels, which may further enhance PFC vulnerability. We briefly highlight the influence of DA, serotonin, glutamate, and cortisol on the PFC given the substantive bodies of research on these neural messengers, although other neurotransmitters (e.g., GABA) and neurohormones are also involved.
DA
DA is a key neuromodulator of the PFC and provides both inhibitory and excitatory influences that help facilitate neural information processing and exchange (Puig, Rose, Schmidt, & Freund, Reference Puig, Rose, Schmidt and Freund2014). Research in both humans and animals has consistently found that DA levels in the PFC influence cognitive performance on a range of tasks, including working memory, planning, and attention (Arnsten, Reference Arnsten2011; Puig et al., Reference Puig, Rose, Schmidt and Freund2014). These findings generally support an inverted-U relation, such that low and high DA levels impair performance (Vijayraghavan, Wang, Birnbaum, Williams, & Arnsten, Reference Vijayraghavan, Wang, Birnbaum, Williams and Arnsten2007), although research increasingly suggests that dose-response patterns vary by cognitive domain/task (Floresco, Reference Floresco2013). Among the psychological disorders in which altered DA is strongly implicated (e.g., psychotic disorders and ADHD), cognitive deficits are typically a feature. In addition to influencing cognitive performance, DA has modulatory influences on synaptic plasticity in the PFC, and is thought to influence important adolescent neuromaturational processes such as prefrontal pruning (Goto, Yang, & Otani, Reference Goto, Yang and Otani2010). Given the important integrative role the PFC plays with respect to afferents from subcortical and other cortical regions, changes in the synaptic plasticity mechanisms in the PFC have the potential for widespread effects on multimodal networks throughout the brain. Finally, due to unique characteristics of the DA system in the PFC, the region is reliant on secondary mechanisms (e.g., catechol-O-methyltransferase [COMT]) to inactive extracellular DA (Diamond, Reference Diamond2007). As a result, genetic polymorphisms and other factors affecting DA levels are hypothesized to have greater impacts on PFC function, relative to other brain regions (Diamond, Reference Diamond2007; Malhotra et al., Reference Malhotra, Kestler, Mazzanti, Bates, Goldberg and Goldman2002).
Serotonin
Current research provides evidence for serotonin influences on the cellular processes supporting early brain development (Daubert & Condron, Reference Daubert and Condron2010), cognitive functioning (Cowen & Sherwood, Reference Cowen and Sherwood2013; Schmitt, Wingen, Ramaekers, Evers, & Riedel, Reference Schmitt, Wingen, Ramaekers, Evers and Riedel2006), corticolimbic activity (Hariri, Drabant, & Weinberger, Reference Hariri, Drabant and Weinberger2006; Volman et al., Reference Volman, Verhagen, den Ouden, Fernández, Rijpkema and Franke2013), and synaptic plasticity (Lesch & Waider, Reference Lesch and Waider2012). For example, serotonin depletion has also been linked to impairments in a number of cognitive functions, including reversal learning (Clarke et al., Reference Clarke, Walker, Crofts, Dalley, Robbins and Roberts2005), attentional and cognitive flexibility (Lapiz-Bluhm, Soto-Piña, Hensler, & Morilak, Reference Lapiz-Bluhm, Soto-Piña, Hensler and Morilak2009), working memory (Cano-Colino, Almeida, Gomez-Cabrero, Artigas, & Compte, Reference Cano-Colino, Almeida, Gomez-Cabrero, Artigas and Compte2014; Enge, Fleischhauer, Lesch, Reif, & Strobel, Reference Enge, Fleischhauer, Lesch, Reif and Strobel2011), and behavioral flexibility (Roberts, Reference Roberts2011), as well as impulsivity (Dalley & Rosier, Reference Dalley and Roiser2012). The serotonin system has also been associated with variation in neural reactivity within corticolimbic circuitry to emotionally salient cues. For example, lower levels of serotonin (as indexed by either 5-HT genotype or 5-hydroxytryptamine receptor 2A [5-HTR2A] density) have been associated with weaker mPFC modulation of amygdalar responses to emotional cues (Fisher et al., Reference Fisher, Meltzer, Price, Coleman, Ziolko and Becker2009; Volman et al., Reference Volman, Verhagen, den Ouden, Fernández, Rijpkema and Franke2013). There is also accumulating evidence that, in addition to an immediate agnoism, certain classes of serotonin receptors (e.g., 5-HTR2A) also lead to activation of pathways involved in induction of synaptic plasticity, particularly through stimulation of brain-derived neurotrophic factor (BDNF). While more work is needed to elucidate this relationship, there is preliminary evidence that serotonin activity in the PFC may influence structural remodeling related to synaptic plasticity (Aznar & Klein, Reference Aznar and Klein2013). More work is needed to clarify the role of serotonin in the PFC, particularly given that serotonin signaling is altered in many forms of psychopathology and many psychotropic drugs target serotonin receptors in the PFC.
Glutamate
The medial PFC (mPFC) is rich in glutamatergic innervations and has been identified as a key site for the mediation of the behavioral effects of glutamate (Diamond, Reference Diamond, Stuss and Knight2002). In both human and animal studies, mPFC-task related activity is altered when glutamatergic agents are administered (e.g., Jackson, Homayoung, & Moghaddam, Reference Jackson, Homayoun and Moghaddam2004). Findings suggest that within the mPFC, glutamate receptors, both AMPA and NMDA, are necessary for set shifting, and that NMDA receptor hypofunction impairs the capacity to modify existing memory stores and diminished the inhibitory control of PFC neurons (Homayoun & Moghaddam, Reference Homayoun and Moghaddam2007). Glutamate concentration in the mPFC has also been found to predict rest-state, cortical–subcortical functional connectivity (Duncan et al., Reference Duncan, Wiebking, Tiret, Marjanska, Hayes and Lyttleton2013), highlighting an important role for glutamate in cortical–subcortical communication.
Neurohormones (adrenal and gonadal)
Research has also documented an influence of adrenal and gonadal hormones, such as cortisol, testosterone, and estrogen, on the expression of genes that govern PFC development and function (Brouwer et al., Reference Brouwer, Koenis, Schnack, van Baal, van Soelen and Boomsma2015; Peper & Dahl, Reference Peper and Dahl2013; Trotman et al., Reference Trotman, Holtzman, Ryan, Shapiro, MacDonald and Goulding2013). Through epigenetic processes, hormones can trigger transcription and protein synthesis of genes involved in neurodevelopment, which in turn influence neuronal structure and function, including neuronal growth, neurotransmitter synthesis and reuptake, and receptor density and sensitivity (Zhang & Ho, Reference Zhang and Ho2011). Thus, changes within these neurohormone systems during certain developmental periods, such as in utero and during puberty, are likely to have significant influences on genetically governed neurodevelopmental processes in the PFC, among other regions. There is also significant interaction and potentiation among neurohormones and neurotransmitter systems (e.g., cortisol and DA, estrogen and DA), which can lead to cascading biological effects (see Sinclair, Purves-Tyson, Allen, & Weickert, Reference Sinclair, Purves-Tyson, Allen and Weickert2014).
Genetic influences
It is now recognized that most of the genes that have been implicated in developmental, psychotic, and mood disorders are not specific to a particular diagnosis, but rather play various roles in cellular aspects of brain development, functional connectivity, neurotransmitter levels, and sensitivity to environmental influences (Buckholtz & Meyer-Lindenberg, Reference Buckholtz and Meyer-Lindenberg2012; Gill, Reference Gill2012; Scharinger, Rabl, Sitte, & Pezawas, Reference Scharinger, Rabl, Sitte and Pezawas2010). Many of the well-documented psychiatric risk genes play important roles in PFC development and functioning, providing further support for the assumption that this region is relevant to a range of psychopathological processes. Genetically determined differences in PFC maturational processes are assumed to moderate the expression of preexisting vulnerabilities and may also give rise to new vulnerabilities when normal maturation is derailed. Given the scope of this review, we briefly highlight genes that have received significant research attention and likely confer vulnerability to PFC development and functions.
The disrupted in schizophrenia 1 (DISC1) and BDNF genes are highly expressed in the PFC and code for proteins important for neural growth, dendritic arborization, and synaptic modulation (Kamiya et al., Reference Kamiya, Tomoda, Chang, Takaki, Zhan and Morita2006; Reichardt, Reference Reichardt2006). These genes are also associated with various structural neuroimaging phenotypes of the PFC (Kamiya et al., Reference Kamiya, Tomoda, Chang, Takaki, Zhan and Morita2006; Porteous, Thomson, Brandon, & Millar, Reference Porteous, Thomson, Brandon and Millar2006). More specifically, DISC1 Cys-allele carriers and BDNF Met-allele carriers both show reduced PFC volumes, and decreased PFC activation during EF tasks, compared to noncarriers (Hashimoto et al., Reference Hashimoto, Numakawa, Ohnishi, Kumamaru, Yagasaki and Ishimoto2006; Kim, Kang, et al., Reference Kim, Kang, Yun, Lee, Jung and Jang2013; Opmeer et al., Reference Opmeer, van Tol, Kortekaas, van der Wee, Woudstra and van Buchem2015; Pezawas et al., Reference Pezawas, Verchinski, Mattay, Callicott, Kolachana and Straub2004; Prata et al., Reference Prata, Mechelli, Fu, Picchioni, Kane and Kalidindi2008) suggesting a link between specific allelic variations and individual differences in PFC structure and function.
Other genes associated with neurotransmitter turnover, availability, and/or metabolism can also moderate individual differences in PFC functioning at both physiological and behavioral levels. For example, a polymorphism in COMT (i.e., Met/Val) confers different levels of enzymatic activity, which effects DA degradation in the PFC and influences the DA milieu in the region. Other genes implicated in PFC neurotransmitter function include DA receptor D2 (DRD2), whose A1 allele is associated with reduced DA binding sites and atypical frontostriatal connectivity during cognitive tasks (Krugel, Biele, Mohr, Li, & Heekeren, Reference Krugel, Biele, Mohr, Li and Heekeren2009; Stelzel, Basten, Montag, Reuter, & Fiebach, Reference Stelzel, Basten, Montag, Reuter and Fiebach2010), and the 5-HTTLPR gene, whose short allele is associated with altered functional and anatomical connectivity between the amgydala and PFC (Hariri et al., Reference Hariri, Mattay, Tessitore, Kolachana, Fera and Goldman2002; Volman et al., Reference Volman, Verhagen, den Ouden, Fernández, Rijpkema and Franke2013). These examples highlight how allelic variation in genes can lead to a variety of individual differences in PFC-related behavioral dimensions such as executive functioning and emotional reactivity.
There is also increasing evidence that de novo germline mutations (e.g., copy number variants) commonly linked to psychopathology (see Geschwind & Flint, Reference Geschwind and Flint2015) involve genes that play a role in cortical development, as well as the physiological processes related to the stress response (Cushion et al., Reference Cushion, Paciorkowski, Pilz, Mullins, Seltzer and Marion2014; Kerner et al., Reference Kerner, Rao, Christensen, Dandekar, Yourshaw and Nelson2013), both of which have obvious implications for PFC vulnerability. There has also been an increased interest in somatic cell mutations, which in contrast to risk alleles, or de novo or inherited germline mutations, can occur in a cell at any point in the life span and are passed along to progeny cells in the course of division (Poduri, Evrony, Cai, & Walsh, Reference Poduri, Evrony, Cai and Walsh2013). While cell replication of cortical neurons occurs primarily in the prenatal period, somatic mutations during this and subsequent periods could lead to changes in brain architecture manifested across development via influences on neuronal connectivity, excitability, and survival (Insel, Reference Insel2014). In conjunction with polygenicity, these types of mutations may help account, in part, for the discordance found in monozygotic twins in nearly every psychiatric disorder.
Of course, genetic vulnerability is not only conferred via risk polymorphisms and mutations. There is increasing evidence that a range of internal (e.g., hormonal) and external (e.g., nutrition and stress) environmental factors modulate genomic function via epigenetic processes, such as DNA methylation and histone modification (Smith, Parets, & Kim, Reference Smith, Parets, Kim, Rhee and Ronald2014). Animal studies have found that environmentally induced epigenetic modifications (hypermethylation and histone acetylation) of both the BDNF and the Reelin genes directly impact long-term potentiation and neural plasticity of the mPFC (Sui, Wang, Ju, & Chen, Reference Sui, Wang, Ju and Chen2012). This finding is particularly relevant because recent studies have found that the Reelin gene is essential for structural and functional organization of the developing PFC (Iafrati, Orejarena, Lassalle, Bouamrane, & Chavis, Reference Iafrati, Orejarena, Lassalle, Bouamrane and Chavis2014) However, the precise mechanisms and epigenetic processes are still to be determined. In addition, more recent evidence suggests that certain genetic polymorphisms and epigenetic processes function less like “vulnerability factors” and more like “plasticity factors,” thereby rendering some individuals more susceptible to both positive and negative environmental influences (e.g., Aguilera et al., Reference Aguilera, Arias, Wichers, Barrantes-Vidal, Moya and Villa2009; Kretschmer, Dijkstra, Ormel, Verhulst, & Veenstra, Reference Kretschmer, Dijkstra, Ormel, Verhulst and Veenstra2013; Wagner, Baskaya, Dahmen, Lieb, & Tadić, Reference Wagner, Baskaya, Dahmen, Lieb and Tadić2010; Wichers et al., Reference Wichers, Kenis, Jacobs, Mengelers, Derom and Vlietinck2008). Given the protracted development of the PFC, greater genetic susceptibility to environmental risks likely impacts PFC-related functions more strongly.
Given the relatively nonspecific role of both common and de novo susceptibility loci in cellular processes, and the relative dearth of findings for disorder-specific risk genes, it is possible that genetic vulnerabilities converge on similar biological pathways. The involvement of key putative psychiatric genes in multiple aspects of PFC neurodevelopment and function suggests that this region may represent a key neural substrate of genetic vulnerability.
PFC Development: Exogenous Influences on Vulnerability
As reviewed above, a range of biological processes influence the development and functional capacity of the PFC. Disruptions in any of these processes have the potential to compromise the PFC, as well as its related circuitry and functions. In addition to the biological processes outlined above, neurodevelopment of the PFC is particularly sensitive to exogenous factors (Kolb et al., Reference Kolb, Mychasiuk, Muhammad, Li, Frost and Gibb2012). While genes contribute to the basic blueprint for neurodevelopment, individual trajectories are driven by the interactions with other factors and forces, including efforts to adapt to unique environmental circumstances. Thus, PFC-mediated individual differences and the diversity of developmental outcomes result, in part, from the accumulation of experience and environmental-dependent shaping across development.
The protracted maturation of the PFC provides a wide window for environmental influences to shape PFC development in ways that can amplify or mollify vulnerability for psychopathology. A number of adverse environmental factors (e.g., abuse, maltreatment, poverty, stress, drugs, parenting, and peer relationships) have been linked to deficits in PFC-mediated EF, emotion regulation, and reward-processing functions (Hanson, Hariri, & Williamson, Reference Hanson, Hariri and Williamson2015; Kim, Evans, et al., Reference Kim, Evans, Angstadt, Ho, Sripada and Swain2013; Lawson, Hook, Hackman, & Farah, Reference Lawson, Hook, Hackman, Farah, Griffin, Freund and McCardle2014; Nikulina & Widom, Reference Nikulina and Widom2013; Raver, Blair, & Willoughby, Reference Raver, Blair and Willoughby2013), as well as psychopathology. The mechanisms through which such environmental experiences can alter PFC functions are extensive and can range from direct impacts on developing neural tissues, architecture, and circuitry, to indirect effects on epigenetic processes, connectivity, neurohormones, and neurotransmitters. In the following sections we briefly highlight some common and well-established exogenous factors that influence PFC development and potentiate neural vulnerability.
Social factors
The growing field of social neuroscience suggests that family, peer, school, neighborhood, and wider cultural contexts can moderate the development of neural circuitry via multiple bidirectional interchanges between social and neural processes, the accumulation of which jointly contribute to developmental outcomes. The influences of social contextual processes on the PFC are highly relevant not only because of the regions sensitivity but also because individual differences in PFC-mediated functions are also likely to shaped by early social contexts. PFC functions can be enhanced by supportive social environments (Bryck & Fisher, Reference Bryck and Fisher2012) and compromised by exposure to aversive, unpredictable, and chaotic environments (Hackman & Farah, Reference Hackman and Farah2009). To enhance the relevance of this review, we highlight the contributions of family and peer social contexts, which have been a growing focus in the literature, are universally experienced by youth, and likely operate as a shared risk factor across variants of psychopathology.
Parenting
Parenting styles provide the foundational social context in which children develop and refine regulatory abilities through observational learning, modeling, and social referencing (Morris, Silk, Steinberg, Myers, & Robinson, Reference Morris, Silk, Steinberg, Myers and Robinson2007). Among parenting factors, there is a large literature documenting the impact that parenting styles, such as emotional responsiveness, warmth, acceptance, criticism, validation, and control, have on a child's emotional development, internalizing problems, aggression, and depression (Bernier, Carlson, & Whipple, Reference Bernier, Carlson and Whipple2010; Bilsky et al., Reference Bilsky, Cole, Dukewich, Martin, Sinclair and Tran2013; Buckholdt, Parra, & Jobe-Shields, Reference Buckholdt, Parra and Jobe-Shields2014; Crowell et al., Reference Crowell, Baucom, McCauley, Potapova, Fitelson and Barth2013). These associations are likely to be complex and characterized by parent-driven effects, child-driven or evocative effects, and recursive processes.
Researchers have speculated that maladaptive parenting styles (e.g., invalidating, controlling, or unresponsive) may increase risk for psychopathology through compromised development of emotion regulation and attentional control in early childhood (Bernier et al., Reference Bernier, Carlson and Whipple2010). More specifically, maladaptive parenting strategies tend to limit opportunities for effective coping, increase dependence on adults, promote coercive interactions, and reinforce avoidance and aggressive regulatory strategies (Crowell et al., Reference Crowell, Baucom, McCauley, Potapova, Fitelson and Barth2013; Eisenberg et al., Reference Eisenberg, Losoya, Fabes, Guthrie, Reiser and Murphy2001; Suveg, Shaffer, Morelen, & Thomassin, Reference Suveg, Shaffer, Morelen and Thomassin2011). In contrast, multiple studies document the association between warm-sensitive and validating parenting and emerging youth emotion regulation (see review by Calkins & Marcovitch, Reference Calkins, Marcovitch, Calkins and Bell2010). These parenting styles provide external soothing and help children cope with frightening or frustrating situations in a way that models self-regulation strategies, which children internalize to develop effective emotion regulation strategies.
At a mechanistic level, parenting effects may operate, in part, through emotion regulation and EF, both of which implicate PFC involvement. This is supported by research that has found that warm-sensitive parenting is associated with child attention set-shifting skills in toddlers (Berneir et al., Reference Bernier, Carlson and Whipple2010) and executive functioning skills in preschool (Hughes, Ensor, Wilson, & Graham, Reference Hughes, Ensor, Wilson and Graham2009). In addition, harsh parenting, low maternal warmth, and maternal negative affect measured in at-risk groups have all been associated with atypical brain structure (e.g., larger regional volumes) and functional engagement (e.g., increased activation) of the PFC, among other brain regions (i.e., amygdala and striatum; Tan et al., Reference Tan, Lee, Dahl, Nelson, Stroud and Siegle2014; Taylor, Eisenberger, Saxbe, Lehman, & Lieberman, Reference Taylor, Eisenberger, Saxbe, Lehman and Lieberman2006; Whittle et al., Reference Whittle, Simmons, Dennison, Vijayakumar, Schwartz and Yap2014; Yap, Allen, & Ladouceur, Reference Yap, Allen and Ladouceur2008). For example, Taylor et al. (Reference Taylor, Eisenberger, Saxbe, Lehman and Lieberman2006) found that children from “risky” families (as indexed from a questionnaire assessing multiple aspects of parenting style and family environment) have different patterns of neural activation to tasks assessing reactivity and response to emotional stimuli, compared to children from “nonrisky” families. In the labeling task, the at-risk group was distinguished by increased amygdala activation and a strong, positive correlation between amygdala activation and right ventrolateral PFC, suggesting that these youth did not recruit the right ventrolateral effectively for regulating amygdala responses to threatening stimuli.
While most research has focused on how parenting affects the child's regulatory development, bidirectional influences are almost certainly at play. Children who are more emotionally dysregulated or inattentive may be more likely to elicit maladaptive parenting responses (e.g., criticism or control) as a result of increasing parental frustration and/or perceived need for external management. For example, Eisenberg, Spinrad, and Eggum (Reference Eisenberg, Spinrad and Eggum2010) found that when toddlers were less self-regulated at 30 months, their mothers used more directive parenting strategies. Through mutual reinforcement, a child with biological vulnerabilities for negative emotional reactivity and poor attention may pull for harsh and invalidating parenting, which in turn fosters coercive parent–child reciprocities during early childhood. As a result, ineffective parenting practices may compound biological vulnerabilities and potentially lead to the development of more serious deficits. While outside of the scope of this review, it is important to consider that parenting styles are often associated with a range of larger social contextual factors (e.g., ethnicity, community, and social economic status), and thus may be a mediator in relationships commonly documented between larger social factors and psychopathology.
Peers
Peer relationships provide another foundational context for development of important skills related to learning social norms, developing, social cognitive abilities, and perspective taking (Rubin & Ross, Reference Rubin and Ross1982). Multiple studies have found that childhood experiences of peer rejection influence the pathways of externalizing and internalizing symptoms (Arseneault, Bowes, & Shakoor, Reference Arseneault, Bowes and Shakoor2010; Brendgen et al., Reference Brendgen, Boivin, Vitaro, Bukowski, Dionne and Tremblay2008; Flanagan, Erath, & Bierman, Reference Flanagan, Erath and Bierman2008; Trentacosta & Shaw, Reference Trentacosta and Shaw2009), including depression and anxiety (Pedersen, Vitaro, Barker, & Borge, Reference Pedersen, Vitaro, Barker and Borge2007), antisocial behavior (Laird, Jordan, Dodge, Pettit, & Bates, Reference Laird, Jordan, Dodge, Pettit and Bates2001), and substance abuse (Fite, Colder, Lochman, & Wells, Reference Fite, Colder, Lochman and Wells2007).
Peer rejection and negative peer experiences deprive youth of important social experiences, opportunities to develop and refine social skills, and sources of support (Rubin, Bukowski, & Parker, Reference Rubin, Bukowski, Parker, Damon and Lerner2006). These experiences also lend themselves to feelings of resentment and cognitive beliefs related to exclusion and lack of belonging (Ladd, Reference Ladd2006). Over time these processes can reinforce restriction of social activities, increased relationship problems, and distress. In addition, decreased opportunities to join prosocial peer groups increases the risk that rejected peers will engage in more deviant behavior and peer groups (Fite et al., Reference Fite, Colder, Lochman and Wells2007). As a result, compounded social skills deficits, restricted social activities, and relationship problems can translate into more global impairments in functioning and psychiatric symptomatology over time.
Individual differences in executive functioning, emotion regulation, and reward processes also likely play a role in exacerbating or ameliorating children's risk for poor peer relationships. Problems managing behavior, inhibiting aggression and impulses, and regulating emotions have significant implications for a child's status in a peer group. A number of recent studies have demonstrated an association between self-regulation and peer relations during both childhood and adolescence (Farley & Kim-Spoon, Reference Farley and Kim-Spoon2014; Holmes, Kim-Spoon, & Deater-Deckard, Reference Holmes, Kim-Spoon and Deater-Deckard2015; Stenseng, Belsky, Skalicka, & Wichstrøm, Reference Stenseng, Belsky, Skalicka and Wichstrøm2015). From a relatively young age, children learn to avoid social interactions with peers who are more extreme in their emotional displays (e.g., anger or fearfulness; Ladd, Reference Ladd2006). Consequently, youth with more extreme disruptive or inhibited tendencies experience greater peer rejection and fewer positive relationships (Hasenfratz, Benish-Weisman, Steinberg, & Knafo-Noam, Reference Hasenfratz, Benish-Weisman, Steinberg and Knafo-Noam2015). Consistent with this, children with attention difficulties have been found to have fewer friendships (Hoza et al., Reference Hoza, Gerdes, Mrug, Hinshaw, Bukowski and Gold2005) and less stable relationships than typically developing peers (Blachman & Hinshaw, Reference Blachman and Hinshaw2002). Meanwhile, withdrawn children have been found to avoid initiating peer interactions (Rubin, Coplan, & Bowker, Reference Rubin, Coplan and Bowker2009).
The interaction between behavioral propensities endowed by PFC dysfunction (e.g., poor emotion regulation, hyperactivity, poor attention, and behavioral inhibition) and peer rejection can amplify existing vulnerabilities and propagate new ones. For example, although externalizing symptoms typically elicit initial peer rejection (Mrug, Hoza, & Gerdes, Reference Mrug, Hoza and Gerdes2001), peer rejection further aggravates the poor outcomes these children experience. Mrug and McCay (Reference Mrug and McCay2013) found that in ADHD youth followed after treatment, peer rejection predicted delinquency, anxiety, and global impairment at 6 years and global impairment at 8 years after baseline. Similarly, in anxious/withdrawn children, initial avoidance facilitates a pattern of restricted social engagement and peer exclusion that subsequently reinforces decreased engagement and motivation for future interactions and amplifies internalizing distress.
Peer experiences become more potent during adolescence. During this time it becomes more common to spend time with peers, place greater value on peers' approval, and to become more concerned about peers' acceptance (Albert, Chein, & Steinberg, Reference Albert, Chein and Steinberg2013; Brechwald & Prinstein, Reference Brechwald and Prinstein2011). This shift is driven, in part, by a suite of normative developmental changes in brain circuitry, neurotransmitters, motivational systems, sexual development, and cultural influences that increase the affective salience of socially related events (Casey & Jones, Reference Casey and Jones2010; Steinberg, Reference Steinberg2010). The combination of these biological changes and a more challenging social landscape increases opportunities and sensitivity to both positive and negative peer experiences. Social support also offers an important buffer to experiences of stress that accompany adolescence, and can provide corrective feedback to depressogenic cognitions that are likely to increase at this age (Panzarella, Alloy, & Whitehouse, Reference Panzarella, Alloy and Whitehouse2006). In adolescence, peer rejection has been associated with social avoidance, and higher levels of anxiety, depression, suicidality, excessive risk taking, and substance abuse (Rubin et al., Reference Rubin, Bukowski, Parker, Damon and Lerner2006).
Stress
A wide range of environmental experiences can affect the PFC through the biological systems that mediate the response to trauma and stress. The PFC is one of the most sensitive brain regions to the effects of stress exposure, and stress-mediated structural changes appear to occur more quickly in the PFC relative to other stress-sensitive brain regions (e.g., hippocampus; Arnsten, Reference Arnsten2009). Converging evidence from animal and human studies has shown that both suppressed and elevated stress levels compromise PFC structural development (Holmes & Wellman, Reference Holmes and Wellman2009; McEwen & Gianaros, Reference McEwen and Gianaros2011; Murmu et al., Reference Murmu, Salomon, Biala, Weinstock, Braun and Bock2006; Weinstock, Reference Weinstock2008), disrupt corticolimbic and frontostriatal circuitry (Arnsten, Reference Arnsten2009; Evans & Schamberg, Reference Evans and Schamberg2009), and impair PFC-dependent functions (e.g., executive functioning; Arnsten & Li, Reference Arnsten and Li2005; Lupien, Gillin, & Hauger, Reference Lupien, Gillin and Hauger1999; Mizoguchi, Ishige, Takeda, Aburada, & Tabira, Reference Mizoguchi, Ishige, Takeda, Aburada and Tabira2004; Vijayraghavan et al., Reference Vijayraghavan, Wang, Birnbaum, Williams and Arnsten2007). As mentioned previously, cortisol can also alter gene expression, which may further compromise neural structure and function.
Work by Pollak et al. (Reference Pollak, Nelson, Schlaak, Roeber, Wewerka and Wiik2010) has demonstrated an association between early life stress (e.g., abuse, neglect, or deprivation) on PFC structure and function in children. For example, children raised in institutionalized settings, an environment where neglect and deprivation are common, display delayed maturation of frontal circuitry (Pollack et al., Reference Pollak, Nelson, Schlaak, Roeber, Wewerka and Wiik2010), aberrant white matter organization in the PFC (Govindan, Behen, Helder, Makki, & Chugani, Reference Govindan, Behen, Helder, Makki and Chugani2010; Hanson et al., Reference Hanson, Adluru, Chung, Alexander, Davidson and Pollak2013), and decrements in PFC-dependent neurocognitive tasks (Bos, Fox, Zeanah, & Nelson, Reference Bos, Fox, Zeanah and Nelson2009; Pollak et al., Reference Pollak, Nelson, Schlaak, Roeber, Wewerka and Wiik2010). Early life stress has also been associated with reductions in OFC volumes in children who have suffered physical abuse, and these volumetric alterations are associated with social difficulties (Hanson, Chung, et al., Reference Hanson, Chung, Avants, Shirtcliff, Gee and Davidson2010). Similarly, Hanson et al. (Reference Hanson, Chung, Avants, Rudolph, Shirtcliff and Gee2012) found that individual differences in PFC volumes accounted for the association between cumulative life stress and spatial working memory in children. These findings suggest that the PFC is sensitive to stress-effects in early childhood and may be a central mechanism linking early life stress to later cognitive and behavioral outcomes.
In addition to early childhood, adverse effects of stress appear to be particularly pronounced during adolescence (Gee & Casey, Reference Gee and Casey2015), a period characterized by normative developmental increases in glucocorticoid secretion and receptors, heightened emotional sensitivity and reactivity, and a more prolonged and exaggerated cortisol response (Eiland & Romeo, Reference Eiland and Romeo2013; Romeo, Reference Romeo2013). For example, Andersen et al. (Reference Andersen, Tomada, Vincow, Valente, Polcari and Teicher2014) found that although hippocampal volume reductions were associated with child sexual abuse at ages 3–5 and 11–13 years, PFC volume reductions were observed only in individuals who experienced childhood sexual abuse at ages 14–16 years. These findings highlight the possibility that adolescence presents a sensitive period for PFC stress effects. This is of high relevance, given that this period is associated with greater stress response as well as increasing autonomy and opportunity for exposure to stressful/traumatic events. Animal studies have also found that stress-induced effects are more permanent and less reversible in adolescents relative to adults (Romeo, Reference Romeo2013).
However, the relationship between stress and the PFC is bidirectional. The PFC influences the stress response at the cognitive level through processing of perceived controllability and at the biological level via modulating effects on activity of the hypothalamus–pituitary–adrenal (HPA) axis. Perceived controllability over stress has been implicated as an important cognitive determinant of individual differences in the stress response. Studies suggest that perceived control is “detected” by the vmPFC, which initiates top-down inhibitory control over activation of limbic structures and blunts the stress response (Maier, Amat, Baratta, Paul, & Watkins, Reference Maier, Amat, Baratta, Paul and Watkins2006; Maier & Watkins, Reference Maier and Watkins2010). In addition, Maier & Watkins (Reference Maier and Watkins2010) work suggest that the joint occurrence of control perception and adverse events produces enduring plastic changes in top-down inhibitory vmPFC systems, such that the same system is activated by later adverse events, even if they are uncontrollable. This proposal provides a potential mechanism to help explain the large body of literature highlighting the influence of early experiences with stress on subsequent responses to stress (Chen & Baram, Reference Chen and Baram2015; Maniam, Antoniadis, & Morris, Reference Maniam, Antoniadis and Morris2014; Pechtel, & Pizzagalli, Reference Pechtel and Pizzagalli2011). Early positive experiences with control (e.g., parental attention, guidance, and warmth in response to distress) as well as negative experiences with control (e.g., abuse and neglect) may influence the development of the stress system in important ways through vmPFC mechanisms. This is particularly relevant because controllability of stress is likely to be lower in children and adolescents, given limitations in their ability to control aspects of their environment.
The mPFC can also provide downregulation of the stress response via negative feedback mechanisms in the HPA axis that regulate neural output from the amygdala (Delgado, Nearing, LeDoux, & Phelps, Reference Delgado, Nearing, LeDoux and Phelps2008). The neurodegenerative effects of chronic stress can compromise this PFC-mediated regulation of the HPA axis, thereby weakening the very region responsible for dampening the stress response, and indirectly facilitating a cycle of increased stress reactivity. As a result, recursive interactions between the stress response and PFC may facilitate an amplified stress response that is exacerbated over time. Clearly, more developmentally informed research is needed on the effects of stress on neural structures and mechanisms.
Substance use
Substance use and abuse has been associated with a number of changes in PFC-mediated functions and networks (Lopez-Larson et al., Reference Lopez-Larson, Bogorodzki, Rogowska, McGlade, King and Terry2011; Squeglia, Jacobus, & Tapert, Reference Squeglia, Jacobus and Tapert2009), and there is evidence from both human and animal research that younger brains are more susceptible to its deleterious effects than are older brains (Hermens et al., Reference Hermens, Lagopoulos, Tobias-Webb, De Regt, Dore and Juckes2013). While a number of drugs have been implicated, we briefly highlight cannabis and alcohol use, which represent the two substances most commonly used by adolescents (Johnston, O'Malley, Bachman, & Schulenberg, Reference Johnston, O'Malley, Bachman and Schulenberg2011). In addition, because most of the research is correlational rather than experimental, causal inferences should be tempered. Nonetheless, experimental animal research has confirmed many of the adverse effects of these substances that have been inferred from studies of humans.
Cannabis
Accumulating evidence suggests that the PFC is particularly vulnerable to cannabis during the early adolescent period, when cortical circuits are being refined (Rubino & Parolaro, Reference Rubino and Parolaro2008). The PFC is enriched with the CB1 subtype of cannabinoid receptor, which increases in density during adolescence. The repetitive use of cannabis during adolescence is hypothesized to blunt the normal developmental increase in CB1 and consequently disrupt PFC maturational processes (Hirvonen et al., Reference Hirvonen, Goodwin, Li, Terry, Zoghbi and Morse2012; Rubino & Parolaro, Reference Rubino and Parolaro2008). Multiple studies have found that early cannabis users (defined as before 16–18 years) show executive dysfunction (Gruber, Sagar, Dahlgren, Racine, & Lukas, Reference Gruber, Sagar, Dahlgren, Racine and Lukas2012; Hanson, Winward, et al., Reference Hanson, Winward, Schweinsburg, Medina, Brown and Tapert2010; Lisdahl & Price, Reference Lisdahl and Price2012), structural alterations (Churchwell, Lopez-Larson, & Yurgelun-Todd, Reference Churchwell, Lopez-Larson and Yurgelun-Todd2010; Gruber, Sagar, Dahlgren, Racine, & Lukas, Reference Gruber, Silveri, Dahlgren and Yurgelun-Todd2011; Lopez-Larson et al., Reference Lopez-Larson, Bogorodzki, Rogowska, McGlade, King and Terry2011; Shollenbarger, Pice, Wieser, & Lisdahl, Reference Shollenbarger, Price, Wieser and Lisdahl2015), and abnormal brain activation (Eldreth, Matochik, Cadet, & Bolla, Reference Eldreth, Matochik, Cadet and Bolla2004; Jager, Block, Luijten, & Ramsey, Reference Jager, Block, Luijten and Ramsey2010) in the PFC relative to noncannabis users. The functional consequences associated with cannabis use (e.g., sleep deprivation and lower grades) may further potentiate the negative consequences of use and confer additional environmental risk.
Alcohol use
Neuroimaging studies of chronic excessive alcohol use consistently reveal structural and functional impairments in the PFC as well as various other brain regions (Bava & Tapert, Reference Bava and Tapert2010; Squeglia, Jacobus, & Tapert, Reference Squeglia, Jacobus and Tapert2014). A smaller number of studies focused on more limited alcohol exposure in adolescents and young adulthood find that alcohol use is associated with reduced prefrontal volumes (De Bellis et al., Reference De Bellis, Narasimhan, Thatcher, Keshavan, Soloff and Clark2005; Medina et al., Reference Medina, McQueeny, Nagel, Hanson, Schweinsburg and Tapert2008), alterations in white matter integrity (De Bellis et al., Reference De Bellis, Van Voorhees, Hooper, Gibler, Nelson and Hege2008; Jacobus et al., Reference Jacobus, McQueeny, Bava, Schweinsburg, Frank and Yang2009; McQueeny et al., Reference McQueeny, Schweinsburg, Schweinsburg, Jacobus, Bava and Frank2009), and alterations in PFC response during certain tasks (Caldwell et al., Reference Caldwell, Schweinsburg, Nagel, Barlett, Brown and Tapert2005; Schweinsburg, McQueeny, Nagel, Eyler, & Tapert, Reference Schweinsburg, McQueeny, Nagel, Eyler and Tapert2010). The effects on white matter integrity are observed even in adolescents who engage in infrequent or subtle binge drinking (Squeglia et al., Reference Squeglia, Jacobus and Tapert2009). However, the direction of the deviation from healthy controls is debated, with inconsistent findings potentially being the result of differences in methodology.
In terms of neuropsychological correlates of adolescent alcohol use, the most consistent findings appear to be impaired executive functioning, especially working memory and attention (Hartley, Elsabagh, & File, Reference Hartley, Elsabagh and File2004; Townshend & Duka, Reference Townshend and Duka2005; Brown & Tapert, Reference Brown and Tapert2004), which appears to remain stably impaired even if abstinence occurs in younger adulthood (Lyvers, Reference Lyvers2000). This suggests that adolescent alcohol use is associated with irreversible changes to the PFC, likely due to the vulnerable nature of protracted PFC maturation. Both alcohol and cannabis have the potential to put youth at heightened risk of acute harm (e.g., accidents) and increased exposure to stressful experiences (e.g., physical fights, unwanted and/or risky sexual encounters, and legal problems).
Taken together, it is clear that PFC mechanisms operate across multiple levels of analysis and are involved in developmental processes that likely give rise to psychopathology. Individual differences in PFC functions that fall on the extreme ends of the spectrum not only underlie maladaptive behaviors but also increase vulnerability to further environmental risk through evocative processes. These transactions lead to an unfortunate cycle in which PFC dysfunction may be further compounded.
PFC Dysfunction in Psychopathology
Several reviews of structural and functional brain changes associated with psychopathology are available elsewhere (e.g., Cao, Shu, Cao, Wang, & He, Reference Cao, Shu, Cao, Wang and He2014; Fusar-Poli, McGuire, & Borgwardt, Reference Fusar-Poli, McGuire and Borgwardt2012; Mochcovitch, da Rocha Freire, Garcia, & Nardi, Reference Mochcovitch, da Rocha Freire, Garcia and Nardi2014; Mulders, van Eijndhoven, Schene, Beckmann, & Tendolkar, Reference Mulders, van Eijndhoven, Schene, Beckmann and Tendolkar2015; Zhang et al., Reference Zhang, Li, Wu, Chen, Hu and Chen2016), so an in-depth overview will not be provided here. Instead, we briefly highlight general trends in the literature to illustrate the PFC dysfunction documented in DSM disorders and focus on imaging finding in youth populations, which are less likely to be confounded by medication and illness duration effects. While we have drawn primarily from the research on internalizing and externalizing syndromes and dimensions in previous sections, we include psychotic disorders here for a number of reasons. First, increasing evidence on the prodromal period suggests that youth experience a range of internalizing and externalizing syndromes prior to psychosis onset (Meyer et al., Reference Meyer, Bearden, Lux, Gordon, Johnson and O'Brien2005). Second, many of the same biological and environmental risk factors that are nonspecific to diagnostic category are implicated in psychosis. Third, the delayed onset of psychosis, relative to other internalizing and externalizing disorders, may constitute the final outcome of the most severe trajectories. Given that the neuroimaging studies have been largely cross-sectional, and those with youth are conducted during periods when prefrontal regions are still developing, we cannot conclude that structural and functional differences represent a biological vulnerability, versus a compensatory adaption or a consequence of illness (Treadway & Pizaggali, Reference Treadway and Pizzagalli2014).
Internalizing disorders
Abnormalities in the structure and function of the PFC have been documented in youth and adults with a variety of internalizing disorders. Consistent with the adult depression literature, studies have identified smaller prefrontal volumes in pediatric depression (e.g., Hulvershorn, Cullen, & Anand, Reference Hulvershorn, Cullen and Anand2011; Steingard et al., Reference Steingard, Renshaw, Hennen, Lenox, Cintron and Young2002), although there is currently less evidence for structural PFC alterations in pediatric anxiety. In a recent review of fMRI studies, Kerestes, Davey, Stephnou, Whittle, and Harrison (Reference Kerestes, Davey, Stephanou, Whittle and Harrison2014) reported evidence of altered functional activation in mPFC regions during resting-state conditions, tasks of emotional processing, cognitive control, and reward-based decision making in medicated and unmediated depressed youth. Reports of both increased (Burghy et al., Reference Burghy, Stodola, Ruttle, Molloy, Armstrong and Oler2012; Sheline et al., Reference Sheline, Barch, Price, Rundle, Vaishnavi and Snyder2009) and decreased (Burghy et al., Reference Burghy, Stodola, Ruttle, Molloy, Armstrong and Oler2012; Guyer et al., Reference Guyer, Lau, McClure-Tone, Parrish, Shiffrin and Reynolds2008; Kim et al., Reference Kim, Loucks, Palmer, Brown, Solomon and Marchante2011) functional corticolimbic connectivity during task-based and resting-state studies have also been reported in internalizing youth. It has been hypothesized that reduced connectivity may reflect ineffective recruitment of the PFC to manage amygdala activity, while increased connectivity may reflect prolonged and persistent experience of negative emotion and/or self-referential rumination. Youth with depression also show altered neural processing of reward (Eshel & Roiser, Reference Eshel and Roiser2010; Price & Drevets, Reference Price and Drevets2010), that is most often characterized by reduced striatal and increased prefrontal activation (Forbes et al., Reference Forbes, Hariri, Martin, Silk, Moyles and Fisher2009; Keedwell, Andrew, Williams, Brammer, & Phillips, Reference Keedwell, Andrew, Williams, Brammer and Phillips2005), although strong positive frontostriatal connectivity in response to rewarding stimuli has also been reported in adolescents with depression (Healey, Morgan, Musselman, Olino, & Forbes, Reference Healey, Morgan, Musselman, Olino and Forbes2014; Morgan et al., Reference Morgan, Shaw, Olino, Musselman, Kurapati and Forbes2015). Across internalizing disorders, deficits in executive functioning (e.g., see meta-analysis by Snyder, Reference Snyder2013), emotion regulation (e.g., see meta-analysis by Aldao, Nolen-Hoeksema, & Schweizer, Reference Aldao, Nolen-Hoeksema and Schweizer2010), and reward processes (e.g., see Eshel & Roiser, Reference Eshel and Roiser2010, for review) have been observed and seem to precede the onset of disorders.
Externalizing disorders
Structural and functional prefrontal dysfunction is widely implicated across disorders in the externalizing spectrum. Longitudinal studies have provided evidence that youth with ADHD show delayed maturation of prefrontal cortical thickness compared to controls (Shaw et al., Reference Shaw, Eckstrand, Sharp, Blumenthal, Lerch and Greenstein2007, Reference Shaw, Malek, Watson, Greenstein, de Rossi and Sharp2013), and that volumes in the PFC are generally reduced, particularly in the dlPFC (Seidman, Valera, & Makris, Reference Seidman, Valera and Makris2005; Sowell et al., Reference Sowell, Thompson, Welcome, Henkenius, Toga and Peterson2003; Yeo et al., Reference Yeo, Hill, Campbell, Vigil, Petropoulos and Hart2003). A meta-analysis of 16 fMRI studies of ADHD revealed consistent brain activation deficits in virtually all regions of the PFC (Dickstein, Bannon, Castellanos, & Milham, Reference Dickstein, Bannon, Castellanos and Milham2006). There is also evidence of disruptions in the white matter tracts comprising the frontostriatal circuit (van Ewijk, Heslenfeld, Zwiers, Buitelaar, & Oosterlaan, Reference van Ewijk, Heslenfeld, Zwiers, Buitelaar and Oosterlaan2012), as well as some evidence for dysfunction in the corticolimbic networks (Castellanos, Sonuga-Barke, Milham, & Tannock, Reference Castellanos, Sonuga-Barke, Milham and Tannock2006; Galvan et al., Reference Galvan, Hare, Parra, Penn, Voss and Glover2006). Studies that have specifically looked at ADHD in comparison to oppositional defiant disorder (ODD)/conduct disorder (CD) suggest that ADHD is more closely associated with dysfunction in the dlPFC network, whereas ODD/CD is more closely associated with the OFC-limbic networks and volume reductions in the orbitofrontal cortex (Huebner et al., Reference Huebner, Vloet, Marx, Konrad, Fink and Herpertz2008; Marsh et al., Reference Marsh, Finger, Fowler, Jurkowitz, Schechter and Henry2011; Rubia et al., Reference Rubia, Halari, Cubillo, Smith, Mohammad and Brammer2011). In addition, studies have found that ODD/CD individuals display hypoactivation in the vmPFC and OFC during reward (Rubia et al., Reference Rubia, Smith, Halari, Matsukura, Mohammad and Taylor2009). Across externalizing disorders, deficits in executive functioning (e.g., Schoemaker, Mudler, Dekovic, & Matthys, Reference Schoemaker, Mulder, Deković and Matthys2013), emotion regulation (e.g., Halligan et al., Reference Halligan, Cooper, Fearon, Wheeler, Crosby and Murray2013), and reward processes (e.g., Crowley et al., Reference Crowley, Dalwani, Mikulich-Gilbertson, Du, Lejuez and Raymond2010; Finger et al., Reference Finger, Marsh, Blair, Reid, Sims and Ng2011) have been observed.
Psychotic disorders
Abnormalities in the structure and function of the PFC are well documented in individuals across the psychosis spectrum (Brent, Thermenos, Keshavan, & Seidman, Reference Brent, Thermenos, Keshavan and Seidman2013). Reduced PFC gray matter volume, particularly in the dorsolateral region, is one of the most consistently replicated findings in chronic (Gur et al., Reference Gur, Cowell, Latshaw, Turetsky, Grossman and Arnold2000; Sullivan et al., Reference Sullivan, Lim, Mathalon, Marsh, Beal and Harris1998), first-episode (Hirayasu et al., Reference Hirayasu, Tanaka, Shenton, Salisbury, DeSantis and Levitt2001; Kubicki et al., Reference Kubicki, Shenton, Salisbury, Hirayasu, Kasai and Kikinis2002), childhood early-onset (Arango et al., Reference Arango, Rapado-Castro, Reig, Castro-Fornieles, González-Pinto and Otero2012) patients, as well as youth at genetic and clinical high risk for schizophrenia (Borgwardt et al., Reference Borgwardt, McGuire, Aston, Gschwandtner, Pflüger and Stieglitz2008; Brent et al., Reference Brent, Thermenos, Keshavan and Seidman2013; Dazzan et al., Reference Dazzan, Soulsby, Mechelli, Wood, Velakoulis and Phillips2012). Widespread disrupted PFC connectivity, including corticolimbic and frontostriatal circuits, has been documented in psychosis-spectrum disorders (Bohlken et al., Reference Bohlken, Brouwer, Mandl, Van den Heuvel, Hedman and De Hert2016; Deserno, Sterzer, Wüstenberg, Heinz, & Schlagenhauf, Reference Deserno, Sterzer, Wüstenberg, Heinz and Schlagenhauf2012; Gee et al., Reference Gee, Humphreys, Flannery, Goff, Telzer and Shapiro2013; Simon et al., Reference Simon, Cordeiro, Weber, Friederich, Wolf and Weisbrod2015; Unschuld et al., Reference Unschuld, Buchholz, Varvaris, van Zijl, Ross and Pekar2014; Woodward et al., Reference Woodward, Waldie, Rogers, Tibbo, Seres and Purdon2009). Deficits in executive functioning (e.g., Fusar-Poli, Deste, et al., Reference Fusar-Poli, Deste, Smieskova, Barlati, Yung and Howes2012; Mesholam-Gately, Giuliano, Goff, Faraone, & Seidman, Reference Mesholam-Gately, Giuliano, Goff, Faraone and Seidman2009), emotion regulation (for reviews, see Kring & Elis, Reference Kring and Elis2013; O'Driscoll, Laing, & Mason, Reference O'Driscoll, Laing and Mason2014), and multiple aspects of reward processing (Gard, Kring, Gard, Horan, & Green, Reference Gard, Kring, Gard, Horan and Green2007; Treadway, Peterman, Zald, & Park, Reference Treadway, Peterman, Zald and Park2015; Waltz, Frank, Robinson, & Gold, Reference Waltz, Frank, Robinson and Gold2007) have been reported in both chronic and prodromal phases of illness.
A PFC-driven heuristic of comorbid expression
By adopting an ontogenic perspective and examining shared vulnerability mechanisms in relation to development, across multiple levels of analysis, we can gain a more complete understanding of how comorbidities, discontinuities, and continuities evolve. As reviewed above, there is considerable evidence that points to PFC-related deficits as pivotal vulnerabilities to psychopathology. Nearly every form of psychopathology includes a constellation of cognitive, mood, and reward disruptions subserved, at least in part, by the alterations in key PFC circuits. The functional consequences of weaknesses in executive functioning, emotion regulation, and reward processing lend themselves to a host of psychiatric symptoms that cut across diagnostic boundaries. Drawing upon previous sections and the ontogenic model outlined by Beauchaine and McNulty (Reference Beauchaine and McNulty2013), we provide a heuristic model of comorbidity, in which PFC-mediated vulnerabilities interact with environmental and contextual risk factors to compound maladaptive behaviors, across domains and dimensions, over time (see Figure 2).
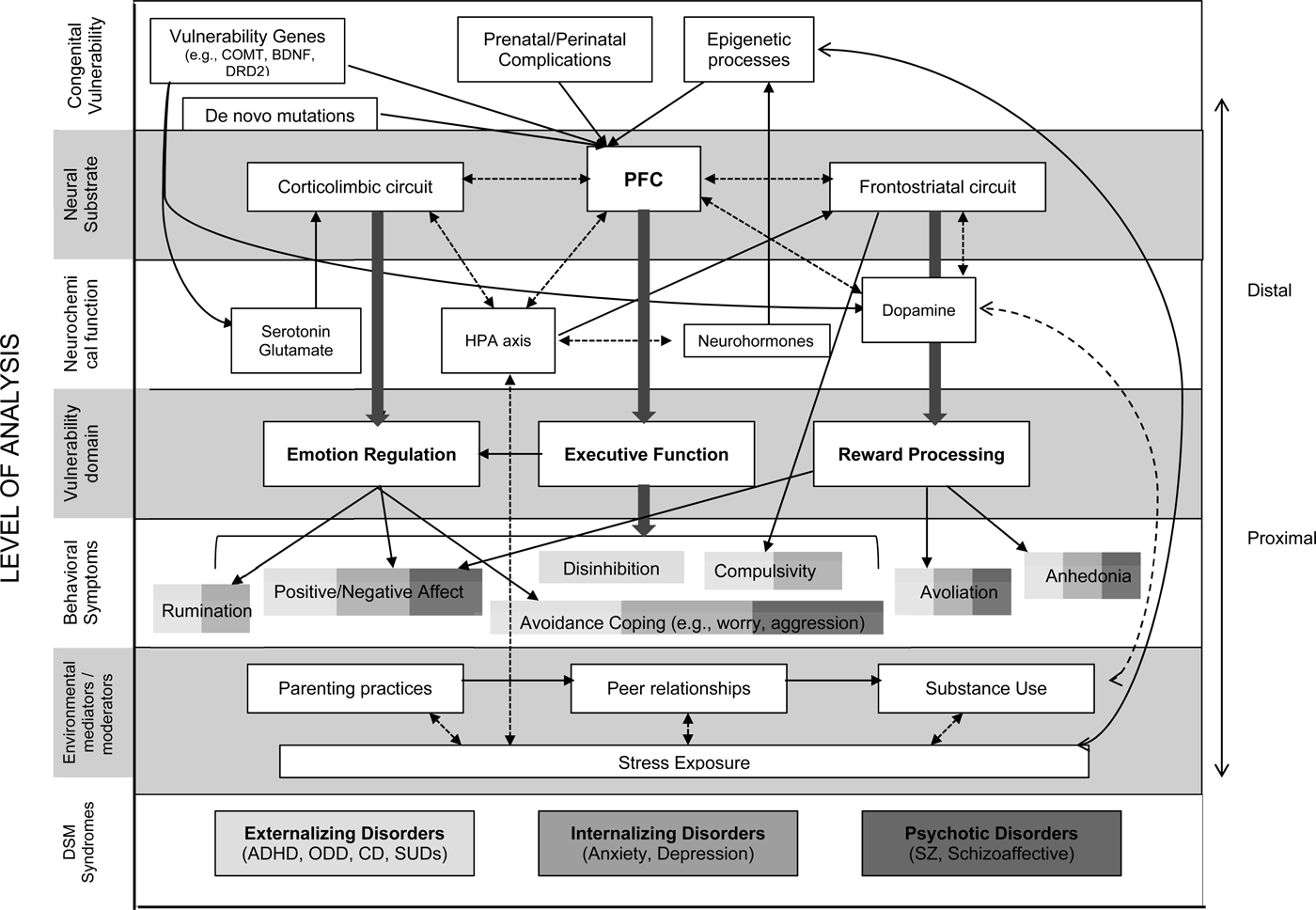
Figure 2. Heuristic model of prefrontal cortex (PFC) mechanisms involved in comorbidity. A heuristic model of PFC mechanisms underlying psychopathology and comorbidity in which levels of analysis is plotted on the y-axis. PFC circuits subserving core functions of executive functioning, emotion regulation, and reward processing are assumed to be key mechanisms of vulnerability for psychopathology. These core functions contribute to a number of transdiagnostic behavioral symptoms that comprise DSM syndromes. Disruption of the PFC and related circuits can arise from factors in the top panel and lead to alterations in core functions. However, PFC-mediated vulnerability can also be instantiated and/or amplified across development by feedback loops that span multiple levels of analysis (e.g., environmental mediators/moderators or neurochemical). Solid arrows denote hypothesized directional processes, and dashed arrows represent bidirectional processes. Gradient shading for the behavioral symptoms corresponds to the DSM diagnostic category each symptom occurs in and highlights a number of symptoms shared among disorder classes (likely contributing to comorbid diagnoses). This model is not comprehensive but highlights both the complexity and the necessity of considering brain mechanisms of psychopathology across multiple levels of analysis.
Given that this review focuses on PFC mechanisms, our heuristic is necessarily simplified and incomplete. There are numerous other biological systems (e.g., mesolimbic DA function), neurobiological substrates (e.g., hippocampus and thalamus), and environmental risk factors at play. Given the PFC's protracted development, it is highly likely that other systems play a foundational role at earlier points in development and influence development of later developing structures like the PFC (see Beauchaine, Reference Beauchaine2015; Beauchaine & McNulty, Reference Beauchaine and McNulty2013). We outline the primary components of our heuristic below.
A pivotal assumption underlying the heuristic in Figure 2 is that, early in life, individual differences in PFC-mediated functional domains produce behavior patterns that render some youth vulnerable to developing psychopathology in the presence of environmental risk. As reviewed above, there are multiple neurobiological pathways (e.g., genetic factors, neurotransmitter alterations, and prenatal insults) through which PFC vulnerability may be initially conferred. It is important to note that vulnerability may not necessarily be conferred congenitally, but may be acquired later in life due to other biological agents and events (e.g., infection agents, encephalitis, neuroinflammatory processes, and traumatic brain injury). These biological influences can precipitate further alterations in developing tissue and circuitry, disruptions of cellular maturational processes, and/or neurotransmitter imbalances that result in individual differences among PFC-mediated functions. While at the extreme ends of the continuum these individual differences may lead directly to problematic behavioral and cognitive tendencies, the majority of individual differences are within the normal range, and risk for psychopathology typically depends on additional environmental and contextual factors.
In some cases, individual differences increase vulnerability for psychopathology, in part, because they are evocative and elicit greater environmental risks. As we have reviewed above, both parenting and peer responses are likely to be shaped, in part, by biological propensities of an individual. For example, individual differences in executive functioning, emotion regulation, and reward processing can lead to expressions such as anxious distress, irritable mood, or disruptive behavior. These behaviors are more likely to elicit negative parenting responses and peer rejection, resulting in limited opportunities for the child's own development of normal emotional, social, and regulatory competencies. In addition, individual differences can also compromise an individual's ability to handle and cope with environmental risks. Research indicates that youth with poorer executive functioning and emotion regulation abilities have greater difficulties coping with peer rejection (Holmes et al., Reference Holmes, Kim-Spoon and Deater-Deckard2015) and may be more vulnerable to stress (Agoston & Rudoph, Reference Agoston and Rudolph2015; Wenzel & Gunner, Reference Wenzel, Gunnar and Masten2013). For example, in a recent study, it was shown that exposure to peer stress predicted heightened risk for depression in girls, but only in those with high levels of executive functioning deficits (Agoston & Rudoph, Reference Agoston and Rudolph2015). Thus, PFC-related deficits not only increase exposure to environmental risk but also simultaneously constrain a youth's ability to effectively manage environmental risks.
Progression to psychopathology occurs through bidirectional transactions between the youth and the environment, which produce a cascade of changes in multiple biological systems. As a result of early interactions between PFC-mediated behavioral tendencies and social environment, dysregulated patterns are increasingly reinforced, and opportunities for normal developmental competencies become constrained. At the biological level, these experiences are likely initiating a cascade of consequences across multiple systems. For example, chronic stress resulting from a negative family climate, poor social interactions, and/or academic failure can affect PFC structure and function through disruptions of cellular processes, functional connectivity, and the neurochemical/hormonal environment. Dynamic interactions among cortisol, DA, serotonin, and glutamate will likely influence corticolimbic, frontostriatal, and HPA systems, among many others. As a result, deficits in PFC functions may be further amplified and biased toward greater dysfunction. During development, when the PFC and related circuitry are still immature, these transactions can instantiate enduring deficiencies within the developing brain circuits, HPA axis, and neurotransmitter systems.
While the PFC is a key neural substrate of individual difference in EF, emotion regulation, and reward processes, the further along one moves through development, the more complex the mediating and moderating pathways from PFC functions to psychopathology become. This complexity results from recursive transactions between biological vulnerability and the environment that initiate cascading effects across social and biological levels. Over the course of development, both dysfunctional biological processes (e.g., stress responding and biased circuitry) and behavioral processes (e.g., avoidance/escape coping) are further reinforced and exacerbated, increasing risk for and severity of psychopathology.
Symptom-driven behaviors (e.g., abnormal reward-seeking or excessive withdrawal) amplify PFC-related deficiencies, which leads to more enduring forms of psychopathology. As an individual continues through development and PFC-related deficits become further entrenched, he/she becomes more likely to seek out nonoptimal environments and engage in maladaptive behaviors that are symptom driven (e.g., abnormal reward seeking or extreme withdrawal). This is particularly the case during adolescence, when increased autonomy provides new environmental affordances, peer pressures, and motivation for substance use, risk taking, and deviant peer affiliations, which introduce additional environmental risks and further compromise PFC functioning. For example, poor regulation of behavior, mood, and reward (all of which implicate the PFC) place individuals at risk for substance abuse (George & Koob, Reference George and Koob2010). As reviewed previously, exposure to alcohol and drugs, whether driven by risk-taking or self-medication motives, can compromise PFC structure and function, amplifying preexisting deficiencies to promote further problems. Substance use can subsequently increase impulsivity and/or withdrawal and imbue functional consequences (e.g., sleep deprivation or lower grades) that confer additional environmental risk.
Moderators of symptom expression
In conjunction with the processes described above, important developmental and contextual moderators shape symptom expression of an underlying (and evolving) vulnerability. These moderators can change the behavioral expression, such that shared vulnerability processes may look different at the syndrome level across times.
Developmental moderators
There are a number of normal developmental processes that likely facilitate age-related differences in PFC mechanisms of symptom expression. For example, clinical phenomena are likely to vary with cognitive maturation, particularly in regard to behavioral responses to negative emotionality and anxious distress. For example, the enhanced cognitive capacities (e.g., rumination, abstract thinking, hypothetical thinking, and future expectances; Steinberg, Reference Steinberg2005) that accompany PFC maturation, and become operative during the adolescent transition, will change how youth experience, cope with, and express negative emotions. While deficits in emotion regulation and executive functioning are more likely to manifest behaviorally (e.g., aggression, tantrum, or defiance) in response to negative mood states in early childhood, these same underlying deficits may assume a more internalizing presentation as development proceeds and new cognitive capacities put youth at greater risk for mood disorders. This is hypothesized to be a contributing factor to the increase of depression rates during adolescence. The cognitive abilities to generate future expectations, engage in hypothetical thinking, and anticipate distal rewards may provide the basis for hopelessness, which is not typically present in younger children.
The potency and availability of certain risk factors also change across development. For example, peer rejection and poor social competence will likely have a stronger effect during the adolescent transition as interpersonal relationships become highly valued and biological systems become more attuned to and motivated by social experiences (Albert et al., Reference Albert, Chein and Steinberg2013). It is possible that the effects of negative peer relationships during adolescence may confer additional internalizing distress on an individual whose presentation had previously been primarily externalizing in nature. With age, opportunities to join deviant peer groups, engage in drug use, and experience significant relationship stress (i.e., friendship turmoil and romantic breakups) also accrue. The advent of intimate and romantic relationships, as well as the increasingly complexity of social groups in early adolescence, introduces new opportunities for rejection and loss. Pubertal development and sexual exploration, particularly among females, similarly affords new opportunities for negative and high-risk sexual experiences.
The predominant expression of symptoms at different ages is often informed by normative development changes and challenges. For example, nonclinical childhood anxiety is typically predominated by fears of separation and specific objects/situations (e.g., animals) in early childhood, by fears about danger and death in middle childhood, and by fears about social competence and performance in adolescence (Weems, Reference Weems2008). This shift parallels the age-related patterns frequently observed across clinical anxiety diagnoses. Thus, developmental changes or “comorbidities” in anxiety diagnoses likely represent continuity of a common vulnerability that is shaped by normal maturational trends, rather than the development of discrete disorders. Finally, transient developmental changes in the biological stress and reward systems certainly affect all adolescents, but for those with preexisting vulnerabilities, this period of instability may be the time in which tendencies toward internalizing, externalizing, and psychotic symptoms become full-blown disorders. For these vulnerable youth, normative adolescent changes may further instantiate long-term alterations in PFC-related functions that would otherwise be temporary and more time limited.
Contextual moderators
In addition to developmental moderators, there are also important contextual moderators that will influence how, if, and to what degree symptoms are expressed. The characteristics and concerns presented by the current context will likely bias symptoms expression. For example, an unstable, threatening environment, or one filled with unpredictable stress, will be more likely to exacerbate and maintain anxiety symptoms by generating emotions of fear and presenting concerns related to safety and consistency. Meanwhile, a social context that is characterized by recent experiences of loss, rejection, and failure may be more likely to manifest as depression, by generating instances of sad affect and concerns of worth and belonging. Thus, while the underlying biological mechanisms may be the same, the emotion and concerns presented by the context will modulate the expression of vulnerability at that time point (Nolen-Hoeksema & Watkins, Reference Nolen-Hoeksema and Watkins2011). With regard to comorbidity, it is possible that contexts that include both characteristics will be more likely to give rise to a comorbid symptom presentation. However, social contexts are far from static and can shift across development, presenting an individual with new concerns. For example, the developmental progression that is often observed between and adolescents and depression (Rutter et al., Reference Rutter, Kim-Cohen and Maughan2006) may result from the introduction of new experiences with rejection, loss, and failure that typically accompanies the normal adolescent experience.
Clinical application
We provide a case example below to illustrate how a comorbid presentation of an externalizing and internalizing disorder may arise based on our heuristic, and how the behavioral expression of PFC vulnerabilities can change across development. While there are a number of potential comorbidities that could be illustrated through our proposed heuristic, we intentionally choose to illustrate an example of heterotypic comorbidity to highlight how synergy between ontogenic and transdiagnostic perspectives can help account for comorbidity, even among disorders that have traditionally been considered distinct (see Beauchaine & McNulty, Reference Beauchaine and McNulty2013; Cummings, Caporino, & Kendall, Reference Cummings, Caporino and Kendall2014, for reviews on homotypic comorbidity). Further, research from both clinical and community samples have found that externalizing disorders frequently co-occur with internalizing disorders (e.g., ADHD and anxiety; CD and depression; Angold, Costello, & Erkanli, Reference Angold, Costello and Erkanli1999; Biederman, Faraone, Mick, & Lelon, Reference Biederman, Faraone, Mick and Lelon1995; Kazdin & Whitley, Reference Kazdin and Whitley2006; Kessler et al., Reference Kessler, Avenevoli, McLaughlin, Green, Lakoma and Petukhova2012).
Case example
Brandon was born at 36 weeks gestational age, weighing 5 lb, 4 oz. Medical records indicate a complication with the umbilical cord during delivery that likely resulted in mild hypoxia. However, Brandon was otherwise in good condition and his neonatal course was uneventful. Brandon's mother described him as a “fussy and temperamental” infant and noted difficulties self-soothing and sleeping. As a toddler, Brandon's mother reported that while he seemed to be more emotionally reactive and more easily frustrated than her other children, there were no significant concerns.
At the start of first grade Brandon began to show behavioral problems both at school and at home. His teachers reported that he was defiant in the classroom, often stubbornly refusing to read aloud or engage in classroom activities. When corrected or redirected by teachers, he quickly became sullen and angry, and had been observed to break his own pencils and tear up his paper. While he was generally a diligent student and seemed motivated to perform, he tended to have difficulty organizing his work and materials, became easily upset by careless mistakes, found it difficult to stay on task, and often fell behind other students in his pace of work. This continued through the second grade, although Bandon was performing above grade level and interacted well with peers.
Brandon's mother also reported difficulties managing his behavior at home. At first, she attributed this to Brandon's failure to adjust to his parents' recent divorce and subsequent stressors in the home. However, as his behavior escalated and persisted, she became exasperated. While she expected to have “normal” arguments about doing chores and homework, she was surprised that she and Brandon fought even about doing fun things (e.g., going to friends birthday party or a trip to an amusement park). She reported that she had never seen such a “wimpy child” and was frustrated at his inability to “toughen up.” She also noted that Brandon seemed unable to just “let things go” and seemed to get stuck on the littlest things. For example, Brandon liked to spend hours in his room building elaborate Lego structures. However, this activity would inevitably end with frustration and toys being thrown. When his mother came to check on him, she reported that he would scream about how he hated Legos, the world, and even her. Homework was also a battle. Brandon often refused to do his work and would break pencils to irritate his mother. This led to verbal arguments that escalated until both parties gave up and Brandon stormed off and slammed doors.
Brandon had always been a friendly and sociable child, but he began to experience difficulties with peers in the third grade. He was often demanding and insisted that other children do things his way. When they did not, he would either sulk or act out aggressively. He was quick to retaliate when he felt teased or embarrassed. As a result, many of his peers began to distance themselves and avoid him. During most of elementary and middle school, Brandon continued to exhibit aggression, defiance, irritable mood, and worry.
During the transition to high school Brandon became less interested in activities he used to enjoy (e.g., basketball and building model airplanes). His mother reported that he seemed to be constantly “down” and “completely unmotivated.” While previously a motivated, albeit difficult student, his academic performance significantly dropped, and he reported it “was not worth his time.” Brandon told his guidance counselor that the future seemed pretty hopeless for him, and it was better not to waste his time trying when he was likely to be disappointed. Early in his freshman year, Brandon became a peripheral member of a deviant peer group. Brandon and his new friends preferred to spend their time smoking marijuana after school and binge drinking on the weekends in various parks. They also regularly stole things from the local shops and vandalized properties. Brandon's mother reported that Brandon sometimes made suicidal threats in response to punishment, but she was not sure if he was serious or just trying to “get a rise out of her.”
This case example aptly illustrates how heterotypic comorbidity can arise from developmental interactional processes. There are, of course, multiple ways in which this particular symptom presentation could have unfolded. The explanation most consistent with our heuristic suggests that shared PFC-related vulnerabilities underlie both oppositional and anxious syndrome presentations, via contributions of PFC-mediated functions. As described in earlier sections, functional alterations in executive functioning, emotion regulation, and reward processing at the extreme ends of the continua, or when exacerbated by environmental factors, can subserve a host of symptoms that fall across traditional diagnostic categories (e.g., worry, rumination, irritable mood, low positive affect, impulsivity, and aggression). While these individual differences do not begin as psychiatric symptoms, they are exacerbated by experiences of chronic stress (e.g., parent divorce or financial stress), negative parental interactions, poor peer relationships, reinforced escape coping, and academic struggles across development. While DSM criteria indicate both ODD and anxiety diagnoses here, this clinical picture is best understood by considering the common vulnerability processes underlying these symptoms.
While this review has focused on the assumption that comorbidities arise from shared vulnerability processes, a developmental cascade account is also possible and not mutually exclusive. In this conceptualization, the functional consequences of one syndrome (e.g., ODD) are hypothesized to potentiate the onset of another syndrome (e.g., anxiety). Previous work suggests that negative developmental outcomes from one disorder can influence other functioning domains that subsequently potentiate risk another disorder (Masten & Cicchetti, Reference Masten and Cicchetti2010). In our case example, the child's early externalizing behaviors begin to garner functional consequences in elementary school, a time in which increased demands for regulation are likely to unmask underlying weaknesses. These behavioral tendencies trigger evocative effects in both the home and the school environment. Negative interactions between the child and his mother reinforce his emotional dysregulation and promote avoidance coping methods, in place of more adaptive coping styles. This socialization effect carries over to his peer interactions, which suffer as a result of his behavioral tendencies. In this case, while the initial impetus is externalizing behaviors, the functional consequences that occur across other domains (e.g., school, peers, and parent), increase the risk for serious internalizing distress (Moilanen, Shaw, & Maxwell, Reference Moilanen, Shaw and Maxwell2010; Obradović, Burt, & Masten, Reference Obradović, Burt and Masten2009; Patterson, DeBaryshe, & Ramsey, Reference Patterson, DeBaryshe, Ramsey, Gauvain and Cole1989).
The developmental trajectory outlined by this case example also illustrates the phenomena of heterotypic continuity (i.e., sequential development of different disorders across the life span). The changes in the child's symptom presentation, while still likely derived from the same underlying vulnerability processes, are further compounded and modulated by developmental factors. For example, increased cognitive capacities (e.g., future thinking and rumination) and accruing experiences of social and academic disappointment introduce new experiences of hopelessness. In addition, peer difficulties early in life increase the risk for deviant peer affiliation in adolescence. The combination of negative peer influences (which have high saliency during adolescence), as well as increased freedom and autonomy associated with adolescence, provides new opportunities to engage in criminal acts and substance use. Although substance use may be driven by deviant peer influence or desires to self-medication (likely both), the usage similarly compounds his PFC deficits (see previous sections) and exacerbates symptoms. While this youth's diagnostic history could include at least four different DSM disorders (depending on the date of assessment), this case is best understood by considering the longitudinal transactions that have occurred across development.
Treatment implications
Drawing upon our heuristic and the themes discussed throughout this review, we offer a few suggestions to guide intervention efforts.
Focus on transdiagnostic processes
As the field moves toward conceptualizing disorders based on transdiagnostic neural circuits and processes, rather than collections of symptoms that exceed a clinical threshold, clearer targets for intervention are likely to be revealed. It will be important for these findings to inform treatment development, such that interventions can be tailored to remediating compromised transdiagnostic processes as observed from symptoms. This is likely to not only increase treatment efficacy by targeting the underlying process (rather than the behavioral expression) but also simultaneously address comorbidity, because treatments would be targeting shared vulnerability processes.
Given the importance of the PFC and its related functions addressed in this review, interventions developed to capitalize on skill acquisition in executive functioning and emotion regulation may be particularly fruitful. Diamond and Lee (Reference Diamond and Lee2011) have reviewed a number of approaches with some evidence for improving EFs in early school years, although further research is needed to address their generalizability and efficacy. In addition, inclusion of techniques specifically aimed at increasing emotional awareness and understanding, effectively managing expressions of sadness and of anger, and reducing engagement in rumination and other avoidant coping methods are important targets of youth interventions.
Target contextual factors
The plasticity and sensitivity of the PFC to environmental factors throughout much of development highlights important treatment avenues that should be a critical component of current preventative interventions, regardless of disorder. As stated repeatedly throughout this review, biological vulnerability is not inevitably expressed in maladaptive behavior or clinical disorder. Thus, there is an incredible opportunity to modify environmental risk processes that may prevent the progression of psychopathology. Given the available research on common environmental risk factors, positive parenting training, social skills training, and general stress management should complement current preventative interventions. From a prevention standpoint, these types of interventions have the potential to mitigate the very processes that often exacerbate existing difficulties and “socialize” further dysfunction. Intervening early, and at multiple levels, has the potential to alter the course of development and provide protective buffers for individuals with biological/preexisting vulnerabilities. These same environmental interventions will be similarly effective for youth with internalizing, externalizing, and comorbid presentations, given their increased risk of eliciting negative parenting interactions, reduced social competences, and greater stress. While medication and therapy can target certain levels of analysis effectively (i.e., neurochemical), attention to contextual environmental factors is necessary, though sometimes neglected.
Consider development
It is imperative that interventions take development into account, in terms of both treatment initiation and design. The relative plasticity of younger brains provides opportunities to confer protective long-term changes in regions such as the PFC, particularly when supported by enriched environments. As reviewed above, maladaptive behaviors and PFC dysfunction becomes increasingly entrenched over time, making it more difficult to intervene and potentially impossible to reverse. Thus, it is critical that intervention efforts are initiated earlier in the developmental trajectory. The selection of appropriate treatment approaches should also consider cognitive maturation. While cognitive behavioral therapy is often the gold standard, it is important to consider whether cognitive approaches are commensurate with the youth's current cognitive abilities and whether cognitive vulnerabilities plays a prominent role in the generation of symptoms at that point in development. It is possible that targeting more reward-related processes via behavioral activation approaches in younger children may be more appropriate. Of course, these decisions will likely vary on a case-by-case basis, depending on the constellation of symptoms and history of the child.
Anticipate continuity
Finally, clinical providers should consider and anticipate comorbidity and continuity. While our understanding of the mechanisms underlying these phenomena is currently limited, descriptive reports of diagnostic patterns warn us of what is likely to lie ahead. Thus, it is important to address risks for disorders that are not yet apparent, but may lie further down the continuum, as well as domains of functioning that are likely to be negatively impacted. For example, while treatment of ADHD in young children may focus on reducing hyperactivity and impulsivity via stimulant medication and behavioral parent training, it is important to consider how risk for compromised peer relationships may unfold and potentiate risk for more serious externalizing symptoms, as well as internalizing problems, down the road. Thus, treatments that address social skills early on, before problems occur, may confer a buffer against worsening psychopathology. The same anticipation of continuity could take place for anxious youth, many of whom will go one to experience depression in adolescence (Rutter et al., Reference Rutter, Kim-Cohen and Maughan2006).
Future Directions
Past assumptions about diagnostic entities and preoccupations with differential diagnoses have significantly impeded our understanding of the developmental interactional processes that set the stage for comorbidity and psychopathology more broadly. These paradigms forced researchers to make difficult, often arbitrary, decisions regarding research design (e.g., to exclude based on comorbidity) that have placed constraints on our understanding of etiologic processes. It is perhaps not unsurprising that diagnostic distinctions have not held up at the genetic, neural, or environmental levels of analysis. The next generation of research must seek to transcend traditional diagnostic boundaries and focus on core dimensional processes and underlying neurobiology, consistent with our contemporary understanding of developmental psychopathology as well as the RDoC initiative.
A focus on transdiagnostic processes is likely to help resolve issues related to comorbidity by identifying shared processes that causally contribute to symptoms that cut across multiple diagnostic entities. Current symptom-based categories can create illusions of distinct and separable processes, when this is unlikely to be true. At the same time, diagnostic categories can similarly create an illusion of similarity, despite significant clinical heterogeneity. A focus on transdiagnostic processes will also have important treatment implications for treating comorbidity. By focusing on the shared underlying processes, clinicians may be alleviating the symptoms across “multiple disorders.” However, these transdiagnostic investigations must also adopt an ontogenic perspective to be fruitful. Traditional transdiagnostic models have difficulty addressing divergent developmental trajectories of symptoms as well as the clinical phenomena of heterotypic continuity. This is where an ontogenic perspective can provide clarity. By delineating transactions between multiple levels of analysis and considering both developmental and contextual moderators, we can begin to understand how individual differences in core functions are shaped into various psychiatric syndromes.
Following this, it will be important to identify core dimensional functions that can best inform our understanding of psychopathology. While the RDoC criteria are intended to do this, they are not yet comprehensive nor do they necessarily reflect wholly or partially independent dimensions. They should map on to mechanisms linking more distal risk factors to symptom expression, as we have attempted to in this review. Obviously, the functions presented in our heuristic are complex, multidimensional, and nuanced. They are only meant to be a starting point for thinking about the larger vulnerability processes at play in psychopathology and comorbidity. Going forward, it will be important for these constructs to be further parsed. For example, different aspects of reward processing are likely to result in different patterns of symptom expression. Relatedly, it will be important to consider these processes in relation to development and tease apart the extent to which these domains are congenital, socialized, and/or compounded by environmental influences.