Stress early in life dramatically affects the prefrontal cortex (PFC; e.g., Demir-Lira, Prado, & Booth, Reference Demir-Lira, Prado and Booth2016; Noble, McCandliss, & Farah, Reference Noble, McCandliss and Farah2007) although less is known about the ways the specific stress related to depressed or anxious prenatal maternal mood affects PFC structure and/or subsequent function during childhood (Neuenschwander & Oberlander, Reference Neuenschwander, Oberlander, Deater-Deckard and Panneton2017). Given that the PFC has the highest density of glucocorticoid receptors in the primate brain and PFC glucocorticoid receptors are important in regulating PFC dopamine levels (Butts, Weinberg, Young, & Phillips, Reference Butts, Weinberg, Young and Phillips2011; Sanchez, Young, Plotsky, & Insel, Reference Sanchez, Young, Plotsky and Insel2000), the child hypothalamic–pituitary–adrenal (HPA) axis may play a significant role in mediating effects of depressed and anxious prenatal maternal mood on child executive functions (EFs) that rely on the PFC.
In the animal literature, it is well established that the maternal and offspring HPA axis plays a crucial role in mediating prenatal stress effects on various outcomes in the offspring (Weinstock, Reference Weinstock2008). Whereas in human studies it is less clear if the maternal HPA axis is responsible for prenatal maternal stress effects on child outcomes (Beijers, Buitelaar, & de Weerth, Reference Beijers, Buitelaar and de Weerth2014; Zijlmans, Riksen-Walraven, & de Weerth, Reference Zijlmans, Riksen-Walraven and de Weerth2015), there is certainly a dearth of human research examining whether altered child/offspring HPA axis activity mediates the association between prenatal maternal stress or prenatal maternal mood disturbances and child outcomes (Glover, Reference Glover and Antonelli2015; Glover, O'Connor, & O'Donnell, Reference Glover, O'Connor and O'Donnell2010). The present study was undertaken in a prospective longitudinal cohort of now 6-year-olds, to examine whether child HPA axis activity under two conditions (diurnal cortisol levels across four typical days and cortisol reactivity in response to a lab challenge stress) contributes to the association between prenatal maternal stress-related mood disturbances (reflected in measures of depressed and anxious maternal mood) and child EFs (assessed with a traditional computerized task; see Figure 1).

Figure 1. Theoretical framework: Mediation model of prenatal stress exposure, hypothalamic–pituitary–adrenal (HPA) axis activity, and executive functions.
Prenatal Maternal Mood and Fetal Programming
Pregnancy is a dramatic biological and psychological period in a woman's life that can affect health and behavior across two generations (Dunkel Schetter & Tanner, Reference Dunkel Schetter and Tanner2012; Matthews & Phillips, Reference Matthews and Phillips2011). It is therefore not surprising that pregnancy and the postpartum period tend to heighten risk for the development or recurrence of maternal mood disorders (Leight, Fitelson, Weston, & Wisner, Reference Leight, Fitelson, Weston and Wisner2010). Prenatal maternal depression occurs in 10%–20% of pregnancies (Bennett, Einarson, Taddio, Koren, & Einarson, Reference Bennett, Einarson, Taddio, Koren and Einarson2004; Marcus, Flynn, Blow, & Barry, Reference Marcus, Flynn, Blow and Barry2003) and about 5% of women take a selective serotonin reuptake inhibitor (SSRI) antidepressant during pregnancy (Oberlander, Warburton, Misri, Aghajanian, & Hertzman, Reference Oberlander, Warburton, Misri, Aghajanian and Hertzman2006). Furthermore, 18%–24% of women suffer from self-reported anxiety symptoms (Dennis, Falah-Hassani, & Shiri, Reference Dennis, Falah-Hassani and Shiri2017). Prenatal maternal mood disturbances have been characterized on multiple dimensions, including stress-related disorders such as anxiety and/or depression (Dunkel Schetter, Reference Dunkel Schetter2011). For the current study, we were interested in symptom severity of such mood disturbances and not in diagnostics or the specific phenotype domain depression versus anxiety. The reason being that programming effects may not be specifically associated with a particular clinical diagnosis, but the natural range of adverse conditions or life events, which have the potential to make the mother feel generally stressed (Glover, Reference Glover2011).
A well-established research literature has found that even before birth, a mother's depressed and/or anxious mood shapes her child's subsequent development of self-regulation, of which, EFs are its cognitive hallmark (e.g., Glover, Reference Glover2011; Mennes, Stiers, Lagae, & van den Bergh, Reference Mennes, Stiers, Lagae and van den Bergh2006; van den Bergh, Mulder, Mennes, & Glover, Reference van den Bergh, Mennes, Oosterlaan, Stevens, Stiers, Marcoen and Lagae2005). The premise that fetal experiences set pathways for health and well-being across the life span is characterized as fetal programming (cf. developmental origins of health and disease; Barker, Reference Barker2003). This implies that fetal development is altered in a way that prepares the offspring for a particular environment it might expect to find after birth (cf. predictive adaptive response; Gluckman & Hanson, Reference Gluckman and Hanson2005). Stress regulatory systems play a pivotal role in the organism's adaptation to the demands of the external and internal environment (cf. allostasis; McEwen & Wingfield, Reference McEwen and Wingfield2003), and individual differences in stress reactivity have been theorized to be evolutionarily selected adaptations that enable the developing organism to match its phenotype to different environmental conditions (Hostinar & Gunnar, Reference Hostinar and Gunnar2013). Therefore, stress regulatory systems and the HPA axis in the offspring, in particular, may be key mechanisms underlying fetal programming and thus mediating the effects of maternal stress on child outcomes (Glover et al., Reference Glover, O'Connor and O'Donnell2010), although other neurocircuits, such as the dopaminergic and serotonergic systems, are likely to be involved as well (Talge, Neal, & Glover, Reference Talge, Neal and Glover2007).
A series of developmental studies in animals, both rodent and nonhuman primate, established the central role of the HPA axis in mediating prenatal stress effects in both mother and offspring (for a review see Weinstock, Reference Weinstock2008). In humans, however, empirical evidence examining offspring HPA activity as a mediator is still missing (for an exception see, van den Bergh, van Calster, Smits, van Huffel, & Lagae, Reference van den Bergh, van Calster, Smits, van Huffel and Lagae2008). The maternal HPA axis, in contrast, has been the most widely investigated biological mechanism of transmission of risk, with mixed results. A recent systematic review (Zijlmans et al., Reference Zijlmans, Riksen-Walraven and de Weerth2015), for instance, found nonsignificant associations between prenatal maternal cortisol concentrations and various child outcomes (e.g., health, cognitive, motor, behavioral, and cortisol) in as many as 76% of studies reviewed. The authors argue that apart from methodological issues (e.g., variation in statistical procedures, variation in assessment of maternal cortisol, and failure to account for confounders and moderators), additional mechanisms that may act in conjunction with, or as moderators or mediators of, the effects produced by the HPA axis should be further explored (e.g., placental functioning of the 11β-HSD2 enzyme and altered health behaviors including eating, sleep, and exercise; Beijers et al., Reference Beijers, Buitelaar and de Weerth2014). Nevertheless, the fact that maternal mood disturbances (anxiety, depression, and perceived stress) during pregnancy are linked to later child outcomes, even after controlling for effects of postnatal maternal mood and other relevant prenatal and postnatal confounders, suggests that, as in animal models, some of the risk is conferred prenatally via changes in women's mood-based physiology affecting fetal neurobehavioral development.
Executive Functioning and Stress
A key assumption underlying the fetal programming hypothesis is that biological systems undergoing rapid developmental changes are especially vulnerable to organizing and disorganizing influences (Seckl & Meaney, Reference Seckl and Meaney1993). Stress early in life, and specifically prenatal maternal stress, may have a particularly large effect on PFC structure and function because of rapid brain development during pregnancy and the high density of glucocorticoid receptors in the PFC region (Arnsten, Reference Arnsten2009; Fuster, Reference Fuster2008; Sanchez et al., Reference Sanchez, Young, Plotsky and Insel2000), which are important in regulating dopamine levels (Butts et al., Reference Butts, Weinberg, Young and Phillips2011; Sanchez et al., Reference Sanchez, Young, Plotsky and Insel2000). In particular, EFs (a set of higher order cognitive processes, such as working memory, inhibition, and cognitive flexibility, associated with PFC and integral to emerging self-regulatory behavior; Blair & Diamond, Reference Blair and Diamond2008; Diamond, Reference Diamond2013) are the first to suffer, and suffer disproportionately, under stress (Arnsten, Reference Arnsten2009; Diamond & Ling, Reference Diamond and Ling2016).
In adults, acute psychosocial stress impairs EFs (e.g., Alexander, Hillier, Smith, Tivarus, & Beversdorf, Reference Alexander, Hillier, Smith, Tivarus and Beversdorf2007; Lupien, Gillin, & Hauger, Reference Lupien, Gillin and Hauger1999). Stress early in life also appears to affect PFC function (e.g., Demir-Lira et al., Reference Demir-Lira, Prado and Booth2016; Noble et al., Reference Noble, McCandliss and Farah2007). One study found that growing up under social or economic disadvantage increased young toddlers’ cortisol levels, which in turn mediated the effects of poverty and parenting on EFs at the age of 3 years (Blair et al., Reference Blair, Granger, Willoughby, Mills-Koonce, Cox and Greenberg2011). When stressful conditions are chronic or persistent, stress response systems are under high allostatic load and adapt to the environment with over- or underactivation to an extent that impedes flexible regulation of stress physiology (McEwen & Wingfield, Reference McEwen and Wingfield2003), which underlies efficient self-regulation and optimal functioning of EFs (Ramos & Arnsten, Reference Ramos and Arnsten2007). To some extent, these effects are believed to reflect the fact that glucocorticoid levels (i.e., cortisol) modulate synaptic activity in the neural circuitry of the PFC.
The functional relationship between cortisol levels and PFC activity or EF performance has been long recognized to be curvilinear (Arnsten, Reference Arnsten2009; de Kloet, Oitzl, & Joels, Reference de Kloet, Oitzl and Joëls1999; Lupien, Maheu, Tu, Fiocco, & Schramek, Reference Lupien, Maheu, Tu, Fiocco and Schramek2007), such that very high or very low levels of stress impair EF performance whereas moderate stress levels lead to optimal EF performance (Blair & Ursache, Reference Blair, Ursache, Vohs and Baumeister2011; Cools & D'Esposito, Reference Cools and D'Esposito2011; Vijayraghavan, Wang, Birnbaum, Williams, & Arnsten, Reference Vijayraghavan, Wang, Birnbaum, Williams and Arnsten2007). There is some evidence, however, that this may only be true for males but not females. Diamond (Reference Diamond2011) summarized differential effects of mild stress on male versus female animals, indicating that male animals perform better on tasks dependent on the PFC when they are mildly stressed as compared to when they are unstressed, but female animals, in contrast, perform more poorly when mildly stressed as compared to when they are unstressed.
This inverted U-shaped relationship may have important implications for beneficial effects of prenatal exposure to mild and moderate levels of maternal stress on certain child outcomes. Evidence of a nonlinear relationship between prenatal stress exposure and child outcomes is supported by DiPietro, Novak, Costigan, Atella, and Reusing (Reference DiPietro, Novak, Costigan, Atella and Reusing2006), who found that exposure to moderate levels of prenatal stress was associated with advanced motor and mental development in the offspring of healthy mothers with low-risk pregnancies.
Prenatal Maternal Mood Shaping Child Executive Functions
Recently, human studies have begun to examine the neurodevelopmental consequences of exposure to maternal stress during gestation. Laboratory-based measures of child neurocognitive development (behavioral measures of EFs and neurophysiological measures indexing PFC structure and activity) offer critical insights into the neural correlates (i.e., specific aspects of children's EFs including underlying structure–function relations) that may be affected by prenatal maternal stress.
Longitudinal studies indicate that prenatal stress is associated with each key component of EFs: inhibition (Buss, Davis, Hobel, & Sandman, Reference Buss, Davis, Hobel and Sandman2011; van den Bergh, Mennes, et al., Reference van den Bergh, Mennes, Oosterlaan, Stevens, Stiers, Marcoen and Lagae2005; van den Bergh et al., Reference van den Bergh, Mennes, Stevens, van der Meere, Börger, Stiers and Lagae2006), cognitive flexibility (i.e., shifting; Mennes et al., Reference Mennes, Stiers, Lagae and van den Bergh2006), and working memory (Buss et al., Reference Buss, Davis, Hobel and Sandman2011; Entringer, Buss, et al., Reference Entringer, Buss, Kumsta, Hellhammer, Wadhwa and Wuest2009; Pearson et al., Reference Pearson, Bornstein, Cordero, Scerif, Mahedy, Evans and Stein2016), as well as with reductions in PFC volume (Buss, Davis, Muftuler, Head, & Sandman, Reference Buss, Davis, Muftuler, Head and Sandman2010) and cortical thinning in the right frontal lobes (Sandman, Buss, Head, & Davis, Reference Sandman, Buss, Head and Davis2015). These findings, however, lack robustness and consistent associations between prenatal stress and EFs given that in several studies multiple tasks tapping various EF components were administered, but associations with prenatal maternal stress were only found for some of the EF tasks. For instance, some authors report impaired performance on working memory tasks (Buss et al., Reference Buss, Davis, Hobel and Sandman2011; Pearson et al., Reference Pearson, Bornstein, Cordero, Scerif, Mahedy, Evans and Stein2016), others do not (Mennes et al., Reference Mennes, Stiers, Lagae and van den Bergh2006; van den Bergh, Mennes, et al., Reference van den Bergh, Mennes, Oosterlaan, Stevens, Stiers, Marcoen and Lagae2005), and still others only find a difference between prenatally stress-exposed individuals and nonexposed individuals after hydrocortisone administration (Entringer, Buss, et al., Reference Entringer, Buss, Kumsta, Hellhammer, Wadhwa and Wuest2009).
While it is unclear which specific aspects of children's EFs and underlying PFC structures and functions are most strongly or consistently altered following exposure to prenatal maternal stress, emerging evidence suggests that prenatal maternal stress is associated with subtle changes in EFs and the PFC in middle childhood, adolescence, and early adulthood. We build on this literature by examining whether prenatal maternal mood predicts child EFs (assessed with a task tapping all three EFs components) at 6 years. We also examined whether the functional relationship between early exposure to stress-related mood disturbances and EF performance is curvilinear (cf. Blair & Ursache, Reference Blair, Ursache, Vohs and Baumeister2011; Cools & D'Esposito, Reference Cools and D'Esposito2011; Vijayraghavan et al., Reference Vijayraghavan, Wang, Birnbaum, Williams and Arnsten2007) such that moderate levels of stress exposure may actually enhance EFs performance.
Some of the studies reviewed above reported sex specific findings. Buss et al. (Reference Buss, Davis, Hobel and Sandman2011) found an association between maternal pregnancy-specific anxiety and inhibitory control in girls but not boys, whereas van den Bergh et al. (Reference van den Bergh, Mennes, Stevens, van der Meere, Börger, Stiers and Lagae2006) detected impaired endogenous response inhibition in adolescent boys but not in girls. Maternal stress may thus induce sex-specific changes in the HPA axis during critical gestation periods, but to date, few human studies have addressed sex differences in child outcomes following prenatal stress exposure, and of those that have, results are mixed. Therefore, we examine whether sex moderates the effects of prenatal stress on the activity of the HPA axis in relation to EFs. Finally, indirect evidence that prenatal maternal stress affects brain development in a way that may also affect the regulation of the HPA axis in offspring (Buss et al., Reference Buss, Davis, Muftuler, Head and Sandman2010; Entringer, Buss, et al., Reference Entringer, Buss, Kumsta, Hellhammer, Wadhwa and Wuest2009) provides an important direction for understanding potential mechanisms.
Prenatal Maternal Mood Shaping Child Stress Regulation
Evaluating how children's stress regulation is affected by prenatal stress exposure should help elucidate our understanding of potential pathways through which the development of EFs may be affected by prenatal maternal mood disturbances. The stress system comprises two main components: the HPA axis with its end product cortisol, and the sympathetic adrenal medullary system (which is part of the autonomic nervous system) with end products epinephrine and norepinephrine. The acute secretion of glucocorticoids (corticosterone in most animals and cortisol in humans) and catecholamines (epinephrine and norepinephrine, also known as adrenaline and noradrenaline) constitute the primary agents in the chain of hormonal events triggered in response to stress. These neurochemicals act together: the catecholamines give rise to the fast “fight-or-flight response” reflected in increased heart rate and blood pressure, while the HPA axis gives rise to a slower response that mobilizes metabolic pathways; affects skeletal muscles, vascular reactivity, nervous system activity, and the immune function; and facilitates physiological and behavioral coping mechanisms. In this way, stress responses serve an adaptive survival mechanism consisting of a carefully orchestrated yet near-instantaneous sequence of hormonal changes and physiological responses enabling an individual to react quickly to threat.
However, frequent or chronic activation can result in a persisting dysregulation of the HPA axis, particularly when experienced during phases of rapid brain development such as the prenatal period, including the fetal period ex utero (in preterm neoantes) and during infancy (Grunau et al., Reference Grunau, Haley, Whitfield, Weinberg, Yu and Thiessen2007; Gunnar & Quevedo, Reference Gunnar and Quevedo2007). Chronic stress exposure, resulting in chronic cortisol elevations, will eventually result in downregulation of glucocorticoid receptors, and alterations in the finely tuned balance among the nervous, endocrine, and immune systems (Chrousos, Reference Chrousos2009; Frodl & O'Keane, Reference Frodl and O'Keane2013). This can utlimately increase neuroinflammation, which itself may underlie both behavioral changes and the development of diseases or disorders later in life, including depression/anxiety, cardiovascular disease, diabetes, and autoimmune disorders (Capuron & Miller, Reference Capuron and Miller2011; Frodl & O'Keane, Reference Frodl and O'Keane2013; Miller & Raison, Reference Miller and Raison2016). Thus, chronic stress exposure has long-term effects on physical and psychological health such as high blood pressure, increased risk of infection, arterial disease, and brain changes that may contribute to anxiety, depression, and addiction (for a general review, see Felitti & Anda, Reference Felitti, Anda, Lanius, Vermetten and Pain2010; Felitti et al., Reference Felitti, Anda, Nordenberg, Williamson, Spitz, Edwards and Marks1998; McEwen, Reference McEwen2000).
Hyperactivation is in general suggested to be indicative of a currently stressed HPA axis (e.g., McEwen & Wingfield, Reference McEwen and Wingfield2003), whereas hypoactivation reflects reduced cortisol production, possibly due to more chronic stress that has downregulated glucocorticoid receptors and resulted in HPA dysregulation (e.g., Doom, Cicchetti, & Rogosch, Reference Doom, Cicchetti and Rogosch2014; Grunau et al., Reference Grunau, Cepeda, Chau, Brummelte, Weinberg, Lavoie and Turvey2013). Moreover, exposure to chronic stress in the early years of life, when the nervous system is still developing, may result in a distinct pattern of dysregulation.
In rodents, studies have found that prenatal stress causes both an increase in basal levels and an increase in corticosterone responses to stress in the offspring, with factors such as sex, age of the offspring, nature and timing of stressor during pregnancy, and so on, playing a modulatory role (for a review, see Weinstock, Reference Weinstock2008). In humans, there appear to be similar fetal programming effects. In Glover et al.’s (Reference Glover, O'Connor and O'Donnell2010) review of the literature 11 studies in the last 10 years revealed an association between prenatal maternal mood or stress and some aspect of HPA axis function in the child. However, perhaps unsurprisingly, the nature of this association varied and solid replications seem to be missing. Overall, these and some additional studies indicate that prenatal stress is linked to both raised diurnal cortisol levels and increased stress responsivity in infancy (Brennan et al., Reference Brennan, Pargas, Walker, Green, Newport and Stowe2008; Davis, Glynn, Waffarn, & Sandman, Reference Davis, Glynn, Waffarn and Sandman2011; Diego et al., Reference Diego, Field, Hernandez-Reif, Cullen, Schanberg and Kuhn2004; Grant et al., Reference Grant, McMahon, Austin, Reilly, Leader and Ali2009; Tollenaar, Beijers, Jansen, Riksen-Walraven, & de Weerth, Reference Tollenaar, Beijers, Jansen, Riksen-Walraven and de Weerth2011), and early to middle childhood (Gutteling, de Weerth, & Buitelaar, Reference Gutteling, de Weerth and Buitelaar2004, Reference Gutteling, de Weerth and Buitelaar2005; O'Connor et al., Reference O'Connor, Ben-Shlomo, Heron, Golding, Adams and Glover2005; Simons, Beijers, Cillessen, & de Weerth, Reference Simons, Beijers, Cillessen and de Weerth2015). Later in development, some studies have found either a reduced cortisol awakening response (CAR) and blunted diurnal cortisol decline in adolescents (O'Donnell et al., Reference O'Donnell, Glover, Jenkins, Browne, Ben-Shlomo, Golding and O'Connor2013; van den Bergh et al., Reference van den Bergh, van Calster, Smits, van Huffel and Lagae2008, however, see Vänskä et al., Reference Vänskä, Punamäki, Lindblom, Tolvanen, Flykt, Unkila-Kallio and Tiitinen2015, for an intensified CAR but nonaffected diurnal cortisol decline in 10- to 12-year-olds) or no differences in diurnal patterns but increased cortisol reactivity during the Trier Social Stress Test among young adults exposed to early stress versus an age-matched comparison group (Entringer, Kumsta, Hellhammer, Wadhwa, & Wust, Reference Entringer, Buss, Kumsta, Hellhammer, Wadhwa and Wuest2009).
These findings provide evidence that early stress exposure is associated with elevated or hyperactivation of the HPA axis (diurnal profiles and reactivity patterns) that could, over time, lead to adrenocortical counterregulation and hypoactivation (Miller, Chen, & Zhou, Reference Miller, Chen and Zhou2007). More research, however, is needed to confirm this longitudinal pattern of the HPA axis following prenatal stress exposure, where glucocorticoid receptor downregulation leads to long-term dampened stress responses.
Whether alterations in HPA axis function mediate the association between early stress exposure and altered behavioral outcomes remains a critical question (Glover et al., Reference Glover, O'Connor and O'Donnell2010). To the best of our knowledge, only one study (van den Bergh et al., Reference van den Bergh, van Calster, Smits, van Huffel and Lagae2008) has so far provided evidence that an altered diurnal cortisol profile in adolescent offspring associated with prenatal anxiety was underlying, thus mediating, an altered behavioral phenotype (i.e., depressive symptoms) in adolescent girls (but not in boys). Here, we investigate both diurnal cortisol levels and reactivity of the HPA axis following prenatal stress exposure, which has not been done in most previous work. Basal (diurnal) levels of HPA functioning, which follow a circadian rhythm, are important to cortisol output, which helps to maintain children's capacity to regulate their emotions and cope with stress. Reactivity of the HPA axis, in contrast, is associated with elevated cortisol levels in response to conditions that are threatening, unpredictable, and lacking in support. Thus, here, we investigate in a comprehensive way if HPA axis activity mediates the association between prenatal maternal mood and later child EFs.
Present Study
Based on prior research and theory, we hypothesize that depressed and/or anxious prenatal maternal mood is associated with poorer EFs in 6-year-olds. Further, we hypothesize that heightened HPA axis activity, reflected in two key components of the child/offspring HPA axis, namely, diurnal and reactivity cortisol levels, mediates the association of depressed and/or anxious prenatal maternal mood and children's EFs (see Figure 1). We also ask whether sex moderates the effects of prenatal maternal mood on HPA axis activity and EFs.
Method
Participants
Participants included 107 children and their mothers from southwestern Canada. A cohort of middle- to high-income pregnant women (n = 191) and their singleton fetuses were recruited during their second trimester (at 26 weeks gestation) from community midwifery clinics and family physician clinics in the greater Vancouver metropolitan area to examine the impact of prenatal SSRI exposure and depressed and anxious prenatal maternal mood on child development. All SSRI-treated mothers had started taking medications based on clinical need, had a diagnosis of a mood disorder, and were already taking SSRIs at the time of conception. Women in both groups (SSRI and nonexposed) had a wide range of anxious and/or depressive symptoms during pregnancy (see Table 1).
Table 1. Descriptives and correlations of child and mother variables and child sex

Note: Child sex: 1 = boys, 2 = girls. Prenatal SSRI exposure: 0 = no, 1 = yes. SSRI, selective serotonin reuptake inhibitor antidepressants exposure. EFs, executive functions. H&F ACC, accuracy in Hearts & Flowers task. AUCg, area under the curve with respect to ground (raw cortisol values). †p = .052. *p < .05. **p < .001.
Of the original 191 mothers, 4 withdrew before the baby was born and another 4 withdrew before the end of the child's first year. At 6 years, an additional 45 children were unavailable for study (families had moved and 4 mothers had withdrawn by 3 years). At the 6-year assessment 107 children (57% girls; M age = 5;11 years, SD = 7 months; n = 44 SSRI-exposed, n = 63 nonexposed) had complete EF data, complete maternal mood measures, and complete diurnal cortisol and/or complete cortisol reactivity data.
Procedure
The study was approved by the University of British Columbia Research Ethics Board and the BC Womens Hospital Research Review Committee. Informed consent was obtained from mothers at the beginning of the study. Mothers were interviewed by trained research assistants using the Hamilton Rating Scales for Depression and Anxiety (HAM-D and HAM-A; Hamilton, Reference Hamilton1960) during the third trimester of pregnancy and again when their children were 6 years of age. At age 6 years, children came to the study center midmorning and were tested individually by trained research assistants. The MacArthur Assessment Battery for Middle Childhood (Alkon et al., Reference Alkon, Goldstein, Smider, Essex, Kupfer and Boyce2003) served as the lab challenge stress procedure and was administered by two research assistants blinded to exposure group status and without the parent present. This 15-min autonomic reactivity protocol for children aged 4–8 years consists of seven blocks, including the following stressors: a social task (questions about family and school); a cognitive task (number-recall task up to six digits); a physical challenge (lemon-juice taste-identification task); and an emotional challenge (two short, emotion-evoking video to elicit fear [a boy having a nightmare] and sadness [a young girl whose pet bird has died]). Age-appropriate relaxing stories were read aloud at the beginning and end of the protocol, and between challenges a quiet inactivity period separated each task. Saliva was collected before, during, and after this procedure to yield measures of children's stress reactivity cortisol. The Hearts & Flowers task (Davidson, Amso, Anderson, & Diamond, Reference Davidson, Amso, Anderson and Diamond2006) was administered to assess EFs as part of a larger battery of tasks, all of which totaled 2 hr of administration on 1 day. In the week before the lab study, the research coordinator visited children's homes and instructed mothers how to collect saliva from the children to yield measures of children's diurnal salivary cortisol.
Measures
Child EFs
Children completed the Hearts & Flowers task, a widely used computerized EFs measure that has been validated with children 4–13 years of age and with adults (previously called the Dots task; Davidson et al., Reference Davidson, Amso, Anderson and Diamond2006; Diamond, Barnett, Thomas, & Munro, Reference Diamond, Barnett, Thomas and Munro2007; Wright & Diamond, Reference Wright and Diamond2014; Zaitchik, Iqbal, & Carey, Reference Zaitchik, Iqbal and Carey2014). This task captures performance of all three components of EFs (inhibition, working memory, and cognitive flexibility [task switching]; Diamond, Reference Diamond2013).
In this task, the child responds to a stimulus (heart or flower) by pressing one of two locations on opposite sides of a touchscreen. There are three blocks of trials. During the first block, which consists of 12 trials, a heart appears on either the left or the right side of the screen and children are instructed to press on the same side as the heart. In the second block, which also consists of 12 trials, a flower appears on either the left or the right side of the screen and children are instructed to press on the side opposite the flower. Thus, children had to resist their prepotent response and instead press on the side opposite the stimulus. Both blocks include a short set of instructions with 4 practice trials (which if necessary can be repeated up to three times). The last block consists of 33 trials where a heart or flower appears on either side of the screen (i.e., mixed block). Children are instructed to press according to the previously learned rules. That is, they had to remember the two rules, mentally translate “same side” or “opposite side” into a left or right response on each trial, and flexibly shift between the two rules, inhibiting one to apply the other.
Stimuli were presented for 1500 ms. Responses >2000 ms were considered incorrect (inattentive) and those <250 ms, impulsive (too fast to have been in response to the stimulus). Both responses were excluded. Outlier trials were removed by using a lower and upper threshold of 2 SD from the mean reaction time per trial type per block and per subject. The dependent variable used in the current analyses was the proportion of correct responses in the mixed block.
Child stress regulation
Children's HPA axis function was studied in two settings: under challenge stress (lab setting) and in an everyday (home) setting. Salivary cortisol served as a biomarker for HPA axis activity. Samples were obtained using a sterile Sorbette (Salimetrics) placed in the inside surface of the cheek for 2–3 min and stored at –20 °C until later assayed. All samples were assayed twice using a commercially available chemiluminescent technique (IBL-Hamburg) at the Technical University of Dresden (Dresden, Germany), and average values were used in analyses. The assay has a sensitivity of 0.16 ng/ml, with intra-assay and interassay coefficients of variation less than 9%.
Area under the curve with respect to ground (AUCg; Pruessner, Kirschbaum, Meinlschmid, & Hellhammer, Reference Pruessner, Kirschbaum, Meinlschmid and Hellhammer2003) was calculated to assess the total hormone concentration over a specific time period (i.e., the day and challenge stress procedure). AUCg indicies were log transformed to minimize skew. Values greater than 3 SD above or below the mean were winsorized (2 participants or 3.4% of all values).
Diurnal cortisol
In the week before the lab challenge study, saliva was collected 3 times on 4 consecutive days to yield measures of diurnal salivary cortisol. Saliva samples were obtained upon awaking (M time = 7:34 a.m., SD = 0.57 min), 20 min postawakening (M time = 7:57 a.m., SD = 0.57 min), and after dinner (M time = 6:50 p.m., SD = 0.57 min). For the current analyses, we calculated the mean for each time point across the 4 days. Due to missing samples, for three children the means were calculated from 3 days (one child) or 2 days (two children). The dependent variable used in the current analyses was AUCg calculated from the three saliva samples.
Stress reactivity cortisol
To determine children's stress reactivity cortisol, five saliva samples were collected over the course of the study day when the lab challenge stress procedure was administered. The sample collected upon arrival to the lab was taken at least 15 min after the child had anything to eat or drink. Two other saliva samples were taken before the lab challenge stress procedure started (one upon completion of the Hearts & Flower task and one upon completion of the Kaufman Brief Intelligence Test). The mean of these first three saliva measures served as the baseline measure (rs = .54–.62, all ps < .001). The next saliva sample was collected 40 min post-stress onset and served as the reactivity measure. Given that the hypothalamic stress responses can be observed in salivary cortisol concentrations approximately 20–30 min post-stress onset and given that our stress challenge procedure lasted 15 min, the probability that we were able to capture children's stress reactivity to the lab challenge stress procedure is relatively high. Finally, a saliva measure was collected at home in the evening (recovery; M time = 6:47 p.m., SD = 71 min). For the current analyses, we replaced missing evening (recovery) values with the mean of evening cortisol values from the diurnal assessements (n = 26). The dependent variable used in the current analyses was AUCg calculated from the three saliva samples (baseline, reactivity, and recovery).
Depressed and/or anxious maternal mood
HAM-D and HAM-A (Hamilton, Reference Hamilton1960) are two clinician rated scales that measure the severity of depression and anxiety sympotms in adults. The HAM-D is based on 21 items scored on a 5-point scale (9 items), a 4-point scale (11 items), or a 3-point scale (1 item) ranging from 0 = none to 4 = disabling, encompassing a range from 0 to 61. The HAM-A is based on 14 items scored on a 5-point scale (ranging from 0 = none to 4 = disabling) with a range from 0 to 56. A trained research assistant administered the scales during the third trimester of pregnancy (M = 34.6 weeks, SD = 1 week), and again at the 6-year assessment (M mothers’ age = 39 years, SD = 5 years). The dependent variable used in these analyses was the mean of both scales to yield a composite measure of depressed and/or anxious maternal mood (the two scales were highly correlated within each time point: r = .89/.92, ps < .001).
Missing data
Of the total 107 children, n = 103 had complete diurnal cortisol data (4 did not have reactivity cortisol), and n = 104 had complete cortisol reactivity data (3 did not have diurnal cortisol). No data were missing for EFs or maternal mood.
Statistical analyses
To test our hypotheses, multiple linear regression models were run (SPSS 20). First, to test the total effect, (i.e., our first hypothesis), we regressed child EFs on prenatal maternal mood, controlling for child age and sex, concurrent maternal mood, and prenatal SSRI antidepressant exposure (binary group status). Second, to examine whether children's stress regulation mediated the association between prenatal maternal mood and child EFs (i.e., our second hypothesis), we estimated two separate mediation models, one for each HPA activity component (i.e., stress reactivity and diurnal levels). For these analyses, we used the “indirect” macro designed for SPSS (Hayes, Reference Hayes2013). This is an empirical bias-corrected bootstrapping procedure, which involves obtaining 50,000 artificial samples drawn with replacement from the existing data and thereby reduces Type 1 error rates by using resampling. This approach is well suited for small sample sizes and accounts for the possibility of nonnormality and/or asymmetry for the indirect effect. In addition, contrary to the more traditional causal steps logic, which requires that both paths a and b are statistically significant (Baron & Kenny, Reference Baron and Kenny1986), individual paths a and b are not required to be significant in order to determine whether M mediates the effect of X on Y (Hayes & Rockwood, Reference Hayes and Rockwoodin press). Parameter estimates and 95% confidence intervals (CI) for all indirect paths were derived and mediation is supported when the CIs do not contain zero.
As child sex may moderate the effects of prenatal stress on the HPA axis, we tested if our mediation models were moderated by sex (i.e., we calculated moderated mediation models where all paths are simultaneously tested for an interaction of sex with the predictor variable). All variables were mean-centered prior to creating the interaction term. Significant interactions were tested for significance in simple slopes to detect areas of significance.
In all our models we controlled for concurrent maternal mood (at 6 years of children's age) in order to isolate the effect of prenatal maternal mood on child outcomes. In addition, we controlled for prenatal SSRI exposure, and time of the day of saliva assessment where required.
Results
Preliminary analyses
Table 1 presents the descriptive data and shows zero-order correlations among the study variables as well as their correlations with sex. As expected, depressed and/or anxious prenatal maternal mood was marginally related to EFs (r = .19, p = .052); thus, the poorer the child EFs, the worse the maternal depression and/or anxiety symptoms during pregnancy. Furthermore, greater cortisol reactivity was associated with poorer EFs. Diurnal cortisol levels, however, were not significantly associated with child EFs. There were no significant associations between prenatal maternal mood and children's HPA axis activity (AUCg scores). Concurrent maternal mood, in contrast, showed small to moderate negative associations with both diurnal and reactivity cortisol levels; that is, the greater the concurrent maternal depression and/or anxiety symptoms, the lower the child cortisol levels. The two indices of HPA axis activity were highly correlated with each other. Depressed and/or anxious maternal mood showed moderate to high stability over the 6-year period and moderate correlations with prenatal SSRI antidepressants intake. Apart from these associations, prenatal SSRI antidepressants exposure was not correlated with any of the study variables, except a positive trend with child sex was found (mothers of girls were slightly more likely to be on SSRI antidepressants during pregnancy). No other significant associations with sex were observed.
Total effect of depressed and/or anxious prenatal maternal mood on child EFs
To test the first hypothesis, a multiple linear regression model was run to examine whether child EFs at 6 years was predicted by prenatal maternal mood, while controlling for mother and child characteristics. Prenatal depressed and/or anxious maternal mood was, as hypothesized, a significant predictor of child EFs at 6 years (β = –.639, p = .014), after controlling for child age, child sex, prenatal SSRI antidepressant exposure, and concurrent maternal mood (Table 2). The quadratic term for prenatal maternal mood was also significantly related to child EFs (β = .511, p = .042), but in an unexpected way. As can be seen in Figure 2, we found a “hockey stick” relationship. At lower levels of depressed and/or anxious prenatal maternal mood, the association between child EFs and maternal mood was linear; namely, fewer correct responses on the third block of the Hearts & Flowers task was associated with increased maternal mood disturbances during pregnancy. However, when prenatal maternal mood reached a certain level of severity, this relationship plateaued and increasing mood disturbances had no further impact on EFs. The relation between prenatal maternal mood and child EFs was not moderated by sex (β = –.020, p = .819). However, in this model sex was marginally related to child EFs (β = –.158, p = .059), indicating that boys had somewhat better EFs than girls.
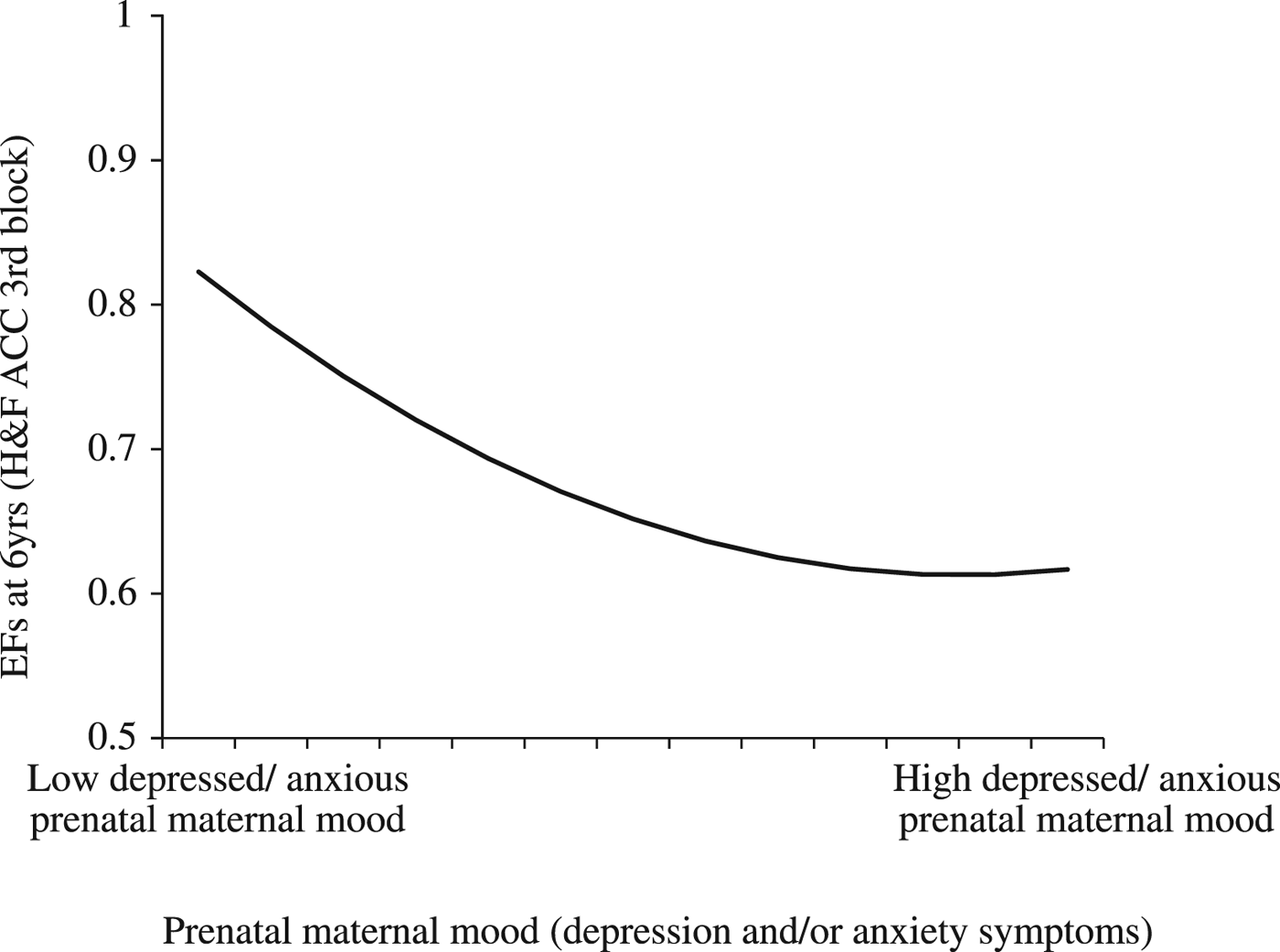
Figure 2. Prenatal maternal mood predicts child executive functions (EFs; accuracy in Hearts & Flowers task) at 6 years while controlling for child sex, child age, prenatal antidepressant exposure, and concurrent maternal mood (linear effect: β = –.639, p = .014, quadratic effect: β = .511, p = .042). At lower levels of depressed and/or anxious prenatal maternal mood, the association between child EFs and maternal mood was linear, namely, fewer correct responses on the third block of the Hearts & Flowers task was associated with increased mood disturbances. However, when prenatal maternal mood reached a certain level of severity, this relation disappeared, and increasing mood disturbances had no ongoing impact on EFs. This may have implications for interventions. Namely, among highly depressed/anxious women, it may be crucial to know that improvements in maternal mood alone may not directly translate into better child outcomes.
Table 2. Multiple linear regression predicting child EFs from prenatal maternal mood (total effect)

Notes: Significant p values are in bold face. B, unstandardized beta coefficients. β, standardized beta coefficients. R 2, explained variance. Child sex: 1 = boys, 2 = girls. Prenatal SSRI exposure: 0 = no, 1 = yes. SSRI, selective serotonin reuptake inhibitor antidepressants exposure. EFs, executive functions.
In sum, depressed and/or anxious prenatal maternal mood (linear effect: β = –.639, p = .014, quadratic effect: β = –.511, p = .042) predicted child EFs after controlling for child age and sex, concurrent maternal mood, and prenatal SSRI antidepressant exposure, suggesting that greater prenatal maternal depression and/or anxiety symptoms are associated with poorer child EFs.
Child HPA axis stress reactivity mediates the association of prenatal maternal mood and child EFs
To test the first mediation hypothesis, a moderated mediation model was run with cortisol stress reactivity as a mediator. The index of moderated mediation was .0053 (.0036) 95% CI [.000001, .0141], indicating that the mediation was moderated by sex (i.e., the CI does not contain zero). Specifically, we found a significant indirect effect of depressed and/or anxious prenatal maternal mood → heightened cortisol reactivity → child EFs for boys, B = –.0053 (.0035) 95% CI [–.0142, –.0004], but not for girls, B = 0 (.0011) 95% CI [–.0021, .0025]. Complete model results, including all covariates, are reported in online-only supplementary materials Table S.1. The mediation model for cortisol reactivity is depicted in Figure 3. Looking at the individual paths, path a1 indicates that more depressed and/or anxious prenatal maternal mood symptoms were associated at trend level with heigthened cortisol reactivity, B = .021, p = .075. However, path a1 also indicates that there was a trend-level interaction of prenatal maternal mood with sex, B = –.038, p = .072.

Figure 3. Bootstrapping results testing the mediation model. Unstandardized estimates. Dashed lines indicate marginally significant paths (p < .10). Significant indirect effects for boys, B = –.0053 (.0035) 95% CI [–.0142, –.0004], but not for girls, B = 0 (.0011) 95% CI [–.0021, .0025]. Controlling for time of the day of saliva assessment, prenatal antidepressant exposure, and concurrent maternal mood.
To further explore this interaction, using z-standardized variables, we examined how prenatal maternal mood interacts with sex to predict cortisol reactivity to lab challenge (z-standardized AUCg scores, log transformed) while controlling for prenatal antidepressant exposure, concurrent maternal mood, and time of the day of saliva assessment (Figure 4). Inspection of the simple slopes revealed that for boys only cortisol reactivity depended on prenatal maternal mood (simple slope for boys: B = .381, p < .05): more depressed and/or anxious prenatal maternal mood symptoms were associated with increased cortisol reactivity to lab challenge. For girls, however, cortisol reactivity remained unchanged even as prenatal maternal mood symptoms worsened (simple slope for girls: B = .022, p = .867).
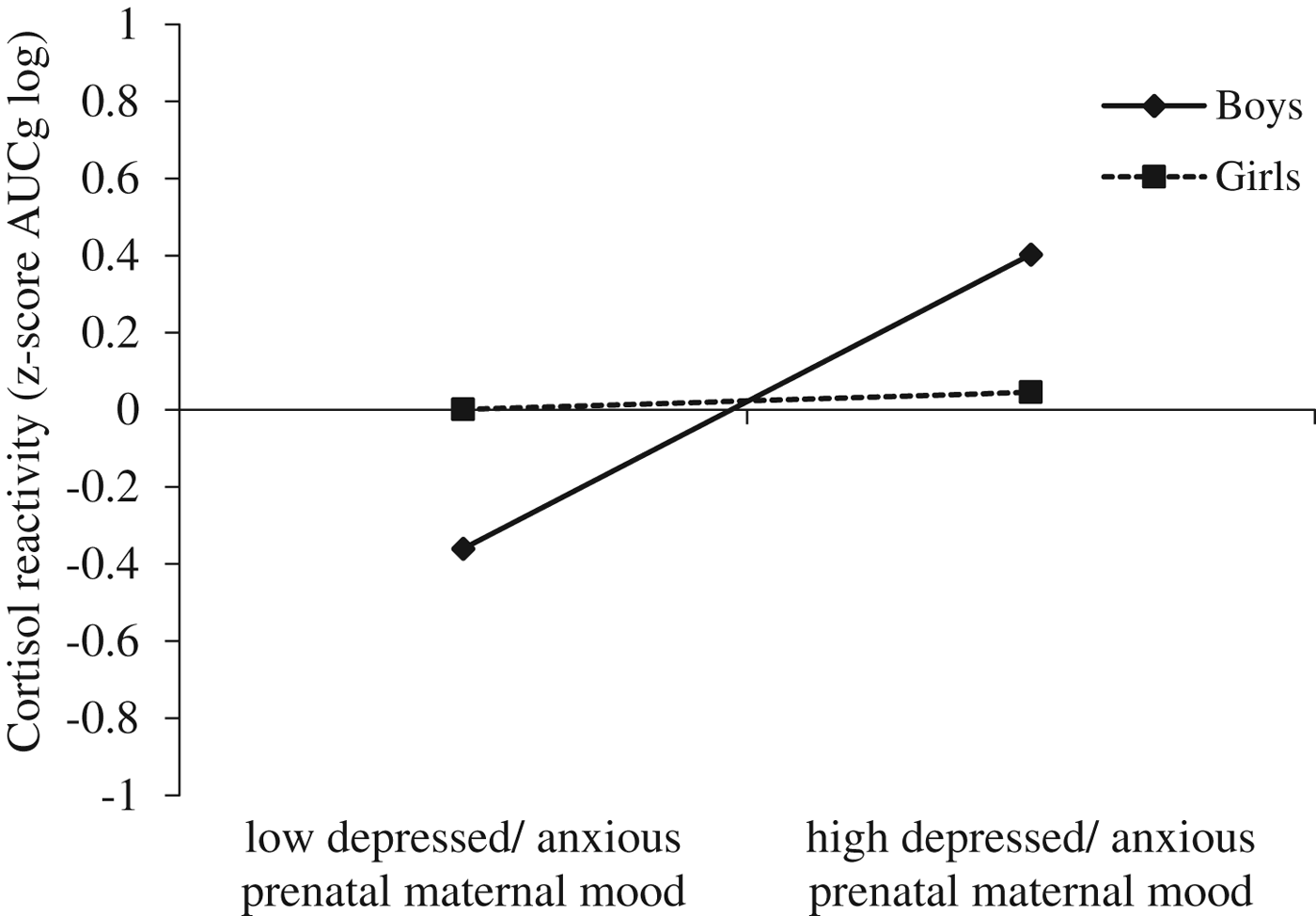
Figure 4. Prenatal maternal mood interacts with sex to predict cortisol reactivity to lab challenge (z-standardized AUCg scores, log transformed) while controlling for prenatal antidepressant exposure, concurrent maternal mood, and time of the day of saliva assessment. For boys only, cortisol reactivity depended on prenatal maternal mood (simple slope for boys: B = .381, p < .05): more depressed/ anxious prenatal maternal mood was associated with increased cortisol reactivity to lab challenge. For girls, however, cortisol reactivity was not associated with exposure to prenatal maternal mood (simple slope for girls: B = .022, p = .867).
Furthermore, path b1 indicates that cortisol reactivity (z-standardized AUCg scores, log transformed) interacted at trend level with sex to predict child EFs at 6 years, controlling for prenatal antidepressant exposure, concurrent maternal mood, and time of the day of saliva assessment, B = .127, p = .095 (see Figure 5). That is, for boys (simple slope: B = –.066, p = .049) but not for girls (simple slope: B = .008, p = .819), higher cortisol reactivity was associated with poorer EFs. Finally, path c1 (direct effect) was significant across girls and boys (B = –.011, p = .022; i.e., it was not moderated by sex).

Figure 5. Cortisol reactivity (z-standardized AUCg scores, log transformed) interacts with sex to predict child executive functions (EFs; accuracy in Hearts & Flowers task) at 6 years, while controlling for prenatal antidepressant exposure, concurrent maternal mood, and time of the day of saliva assessment. For boys only, EFs depended on cortisol reactivity: higher cortisol reactivity was associated with poorer EFs (simple slope for boys: B = –.066, p = .049). For girls, in contrast, cortisol reactivity was not associated with EFs (simple slope for girls: B = .008, p = .819).
In sum, these findings indicate that depressed and/or anxious prenatal maternal mood was negatively associated with child EFs via heightened cortisol reactivity, but only for boys. Prenatal maternal mood, however, had a significant direct effect on child EFs for both girls and boys.
Child diurnal HPA axis activity mediates the association of prenatal maternal mood and child EFs
To test the second mediation hypothesis, we again ran a moderated mediation model with diurnal cortisol levels as a mediator. The index of moderated mediation was –.0023 (.0035) 95% CI [–.0048, .0087], indicating that this mediation was not moderated by sex (i.e., the CI contains zero). There was neither an indirect effect for boys, B = –.0022 (.0034) 95% CI [–.0086, .0047], nor for girls, B = 0 (.0008) 95% CI [–.0014, .0020]. Complete model results, including all covariates, are reported in online-only Supplementary Table S.2. The mediation model for diurnal cortisol is depicted in Figure 6. Looking at the individual paths, path a2 indicates that more depressed and/or anxious prenatal maternal mood symptoms were associated with heightened diurnal cortisol levels, B = .023, p = .029. However, path a2 also shows that there was a significant interaction of prenatal maternal mood and sex, B = –.52, p = .008.

Figure 6. Bootstrapping results testing the mediation model. Unstandardized extimates. Dotted lines indicate nonignificant paths. No indirect effect. Controlling for time of the day of saliva assessment, prenatal antidepressant exposure, and concurrent maternal mood.
To further understand this interaction, using z-standardized variables, we examined how prenatal maternal mood interacts with sex to predict diurnal cortisol levels (z-standardized AUCg scores, log transformed) while controlling for prenatal antidepressant exposure, concurrent maternal mood, and time of the day of saliva assessment (Figure 7). For boys (simple slope: B = .492, p = .002), but not for girls (simple slope: B = –.027, p = .833), diurnal cortisol levels depended on prenatal maternal mood. Namely, more depressed and/or anxious prenatal maternal mood symptoms were associated with higher diurnal cortisol levels.

Figure 7. Prenatal maternal mood interacts with sex to predict diurnal cortisol levels (z-standardized AUCg scores, log transformed) while controlling for prenatal antidepressant exposure, concurrent maternal mood, and time of the day of saliva assessment. For boys only, diurnal cortisol levels depended on prenatal maternal mood: more depressed and/or anxious prenatal maternal mood was associated with higher diurnal cortisol levels (simple slope for boys: B = .492, p = .002). For girls, however, diurnal cortisol levels were not associated with exposure to prenatal maternal mood (simple slope for girls: B = –.027, p = .833).
Path b2 was not significant, B = –.008, p = .870 (also no significant interaction with sex), indicating that children's diurnal cortisol levels were not associated with EFs performance. Finally, path c2 (direct effect) was significant, B = –.014, p = .008, across girls and boys.
Taken together, among boys we observed that heightened cortisol reactivity but not diurnal cortisol levels mediated the association between depressed and/or anxious prenatal maternal mood and EFs. However, it is important to note that prenatal maternal mood was also associated with EFs in girls as indicated by the significant total effect and both significant direct effects in the diurnal cortisol model and the cortisol reactivity model.
Discussion
Prenatal exposure to maternal mood disturbances has been reported to shape a child's subsequent development of EFs. However, specific mechanisms that link the prenatal environment with postnatal outcomes require further investigation. By examining children's cortisol responses under both unstressed conditions and following stress, this study sought to determine the role of the child/offspring HPA axis in mediating effects of prenatal maternal mood on child EFs at age 6 years. We found that in boys but not girls, depressed and/or anxious prenatal maternal mood is associated with both heightened diurnal cortisol levels and heightened cortisol reactivity to a lab challenge, and that heightened reactivity is associated with poorer EFs. Further, among boys, cortisol reactivity but not diurnal cortisol levels mediated the association between depressed and/or anxious prenatal maternal mood and EFs. The total effect of depressed and/or anxious prenatal maternal mood on child EFs, however, was significant for both girls and boys. Specifically, depressed and/or anxious prenatal maternal mood predicted child EFs up to a point and then asymptoted so that even worse prenatal maternal mood symptoms had no further impact on child EFs. To the best of our knowledge, these are the first findings that demonstrate a role for child stress regulation in mediating the relationship between prenatal maternal stress-related mood disturbances and child EFs, thereby providing insight into potential pathways through which EF development (at least in boys) may be affected by depressed and/or anxious prenatal maternal mood.
Total effect on executive functions
Given the central role of EFs for child development and health, these findings highlight the importance of incorporating the prenatal period into our models of EF development. In line with the notion of equifinality (Cicchetti & Rogosch, Reference Cicchetti and Rogosch1996), a central tenet of developmental psychopathology denoting that there are many developmental pathways that may lead to the same outcome, our findings expand on the child development literature showing that EFs are malleable, and context-specific experiences matter both at home and at school (for reviews, see Diamond & Lee, Reference Diamond and Lee2011; Hughes, Reference Hughes2011; Ling, Kelly, & Diamond, Reference Ling, Kelly, Diamond, Freund, McCune, Esposito, Gee and McCardle2016). Specifically, our findings add to the emerging literature within the field of prenatal maternal stress showing that depressed and/or anxious prenatal maternal mood is associated with subtle changes in EFs and frontal lobe structures in middle childhood, adolescence, and early adulthood (e.g., Buss et al., Reference Buss, Davis, Hobel and Sandman2011; van den Bergh, Mennes, et al., Reference van den Bergh, Mennes, Oosterlaan, Stevens, Stiers, Marcoen and Lagae2005).
Contrary to our hypothesis, we were not able to detect an inverted U-shaped relation between prenatal stress and EFs (Arnsten, Reference Arnsten2009). In our sample, the data were best represented by a regression line that flattened when depressed and/or anxious prenatal maternal mood reached a certain level of severity. This may have implications for interventions. Namely, among highly depressed and/or anxious women, it may be crucial to know that improvements in maternal mood alone may not directly translate into better child outcomes. Possibly, beyond mood itself, related but less prominent maternal characteristics of mood disorders such as maternal cognitive disturbances may also shape child outcomes (Snyder, Reference Snyder2013). Clearly, replication of our findings with another cohort and/or other EF tasks is important. Future research is also needed to address the question when and how moderate stress/cortisol levels experienced during the prenatal period may lead to optimal EF performance.
Mediation through child HPA axis stress reactivity
Our findings may also reflect multifinality, the other central tenet of psychopathology, which refers to the notion that any one process may function differently across systems or within individuals (Cicchetti & Rogosch, Reference Cicchetti and Rogosch1996). Thus, not all children are affected equally by prenatal maternal stress or mood disturbances. Our finding indicating that cortisol reactivity but not diurnal cortisol levels mediated the effect of prenatal maternal mood on EFs for boys only suggests that there probably are different mechanisms underlying the effects of exposure to depressed/ anxious prenatal maternal mood symptoms on EFs for girls (e.g., immune system and inflammation, such as the pro-inflammatory cytokines, Coussons-Read, Okun, & Nettles, Reference Coussons-Read, Okun and Nettles2007; epigenetics, Oberlander et al., Reference Oberlander, Weinberg, Papsdorf, Grunau, Misri and Devlin2008; autonomic nervous system responses, Suurland et al., Reference Suurland, van der Heijden, Smaling, Huijbregts, van Goozen and Swaab2017; dopaminergic systems, Zhang, Chretien, Meaney, & Gratton, Reference Zhang, Chretien, Meaney and Gratton2005).
Within the literature of developmental effects of prenatal exposure to substance abuse (e.g., nicotine), animal studies have demonstrated sex differences, with males generally showing higher vulnerability than females (e.g., Shacka, Fennell, & Robinson, Reference Shacka, Fennell and Robinson1997). Similarly, among humans, substance-exposed boys showed greater cognitive deficits than exposed girls (Moe & Slining, Reference Moe and Slining2001). In contrast, a study showing that altered HPA activity played a mechanistic role in fetal programming of child behavioral outcomes found evidence for mediated effects of prenatal anxiety on depressive symptoms for 14- to 15-year-old girls but not boys (van den Bergh et al., Reference van den Bergh, van Calster, Smits, van Huffel and Lagae2008). The authors argue that maternal anxiety may induce sex-specific changes in the HPA axis during critical gestation periods, which only become apparent postpuberty, when the HPA axis has reached its full maturation. Alternatively, they argue, it may also be plausible that only during puberty do females become more vulnerable to depressive symptoms because of crucial sex differences in gonadal hormones. It will be interesting to see what we find when the children in our study reach puberty. As well, future research needs to explore further the possibility that the timing of the exposure to maternal depression and anxiety as well as the amount of sex hormones in the developing fetus may be relevant for sex differences in the outcome (de Bruijn, van Bakel, & van Baar, Reference de Bruijn, van Bakel and van Baar2009; Glover & Hill, Reference Glover and Hill2012).
Prenatal stress has been associated with HPA axis dysregulation in infants, adolescents, and adults (reviewed in Glover et al., Reference Glover, O'Connor and O'Donnell2010). Our findings add to and expand on this literature as our study is one of the very few showing that prenatal maternal mood is associated with both aspects of children's HPA axis activity (heightened diurnal cortisol and heightened stress reactivity levels). Whether and how sex moderates the effects of prenatal stress or maternal mood on the development of the HPA axis remains a critical question. Contrary to our results, prior research has found that both prenatal maternal anxiety and prenatal exposure to synthetic glucocorticoids increased stress reactivity in girls only (Alexander et al., Reference Alexander, Rosenlocher, Stalder, Linke, Distler, Morgner and Kirschbaum2012; de Bruijn et al., Reference de Bruijn, van Bakel and van Baar2009). Whether HPA axis dysregulation appears to be sex specific during specific developmental periods (i.e., if it appears early on and again later in girls or whether it continues to be true only for boys in our sample) remains unknown. Future studies analyzing how sex moderates the relationship between prenatal maternal depression and/or anxiety symptoms and development of the HPA axis are necessary to reconcile inconsistent findings in the literature.
Apart from sex, genetics have been shown to be powerful moderators of prenatal maternal exposures. A recent study (Buchmann et al., Reference Buchmann, Zohsel, Blomeyer, Hohm, Hohmann, Jennen-Steinmetz and Laucht2014), for example, shows that in 19-year-old adolescents exposed to prenatal maternal stress, only carriers of the dopamine D4 receptor gene (DRD4) seven-repeat allele were found to have altered (i.e., attenuated) cortisol secretion during the Trier Social Stress Test. These results suggest that prenatal maternal stress may only affect the HPA axis of carriers of certain “risk alleles” (the DRD4 7r allele has been shown to be a “risk allele” for externalizing problems, particularly in the presence of environmental adversity; Bakermans-Kranenburg & van IJzendoorn, Reference Bakermans-Kranenburg and van IJzendoorn2006). In our own cohort, we found that among children whose mothers exhibited some degree of depressive-anxious affect during pregnancy, a child's EFs at age 6 varied depending on the child's serotonin transporter (SLC6A4) genotype and his/her mother's mood (Weikum et al., Reference Weikum, Brain, Chau, Grunau, Boyce, Diamond and Oberlander2013). The EFs of children with at least one short allele of the gene were well preserved even if their mothers reported many depressive symptoms (i.e., they showed resilience), whereas the EFs of children with two long forms of the SLC6A4 gene appeared to be very sensitive to their mothers’ mood. If their mothers were more symptomatic, these children showed worse EFs than those in any other group; but if their mothers were happier, these children's EFs were better than those of any other group. Thus, context counts whereby children of more depressed mothers with one or two short alleles of the SLC6A4 gene showed the better EFs, but given a mother who was not symptomatic, children with two long alleles showed the best EFs. Another intriguing example of multifinality or “biological sensitivity to context”/ “differential susceptibility” (Ellis, Boyce, Belsky, Bakermans-Kranenburg, & van IJzendoorn, Reference Ellis, Boyce, Belsky, Bakermans-Kranenburg and van IJzendoorn2011) was provided by Pluess et al. (Reference Pluess, Velders, Belsky, van IJzendoorn, Bakermans-Kranenburg, Jaddoe and Tiemeier2011), who showed that the association between maternal anxiety during pregnancy and negative emotionality in early infancy was only significant in infants carrying one or more copies of the serotonin transporter linked polymorphic repeat (5-HTTLPR) short allele but not in those homozygous for the long allele. In this way, the 5-HTTLPR allelic variations might increase vulnerability to adverse environmental influences as early as the fetal period for some infants. Furthermore, in a study of infants born very preterm and exposed to the stress of multiple procedures during fetal life ex-utero, Chau et al. (Reference Chau, Ranger, Sulistyoningrum, Devlin, Oberlander and Grunau2014) showed that variation in the catechol-O-methyltransferase (COMT) genotype moderated the relationship between early stress and internalizing behaviors at 18 months corrected age. The idea of biological sensitivity to context/differential susceptibility offers a critical perspective that allows us to move our thinking about fetal programming from “invariant” developmental outcomes associated with early adverse exposure to a perspective that outcomes in childhood represent interactions between biological (a child's genotype) and contextual (maternal mood) variables enabling both positive and negative outcomes.
Executive functions and cortisol responses
Physiological arousal or children's cortisol responses and EF skills are not linked in a fixed linear pattern, but are more accurately represented in a complex dynamic interplay. In our sample, a heightened HPA axis reactivity but not a heightened diurnal HPA axis profile was associated with poorer EFs. These findings are mostly in line with a small number of studies with children suggesting that heightened cortisol reactivity and heightened baseline cortisol levels are negatively associated with effortful control and EF performance (Blair et al., Reference Blair, Granger, Willoughby, Mills-Koonce, Cox and Greenberg2011; Donzella, Gunnar, Krueger, & Alwin, Reference Donzella, Gunnar, Krueger and Alwin2000; Wagner et al., Reference Wagner, Cepeda, Krieger, Maggi, D'Angiulli, Weinberg and Grunau2015). In contrast, other studies have found that cortisol reactivity was associated positively with preschoolers’ effortful control and EFs (Blair, Granger, & Peters Razza, Reference Blair, Granger and Peters Razza2005; Spinrad et al., Reference Spinrad, Eisenberg, Granger, Eggum, Sallquist, Haugen and Hofer2009). More nuanced analyses suggest, however, that the interplay between physiological arousal/cortisol responses and EF skills is even more complex. Blair and Berry (Reference Blair and Berry2017), for instance, recently reported that young children with relatively low cortisol levels, showing moderate fluctuations in their cortisol levels over the first 4 years of their lives, tended to show better EF performance at 5 years of age than did children with either highly stable or highly variable temporal profiles. Recent research also suggests that heightened cortisol reactivity may promote EF skills in nurturing contexts, but undermine them in less resourced or less supportive contexts (probably because the children are too stressed). In a study of kindergarteners, cortisol responses interacted with family income to predict EFs (Obradović, Portilla, & Ballard, Reference Obradović, Portilla and Ballard2016): heightened cortisol levels were associated with better EFs in children from higher income families and with worse EFs in children from lower income families. It is an open question if children in our sample experienced a less resourced or less supportive context and therefore their heightened HPA axis reactivity was associated with worse EFs. Clearly context matters, and research is needed to clarify under which conditions heightened cortisol reactivity and/or heightened baseline cortisol levels are adaptive versus maladaptive and may therefore represent a plasticity versus risk factor.
It is somewhat surprising that we only found a relationship between heightened cortisol reactivity and lower EFs for boys and not girls. Based partly on the animal literature, researchers have emphasized that it is important to examine potential differential sex effects when investigating relationships between stress reactivity and child outcomes (Spinrad et al., Reference Spinrad, Eisenberg, Granger, Eggum, Sallquist, Haugen and Hofer2009). In line with our findings, Donzella et al. (Reference Donzella, Gunnar, Krueger and Alwin2000) reported that all but one of the children who showed an increase in cortisol in response to a competition were male. These boys were described by teachers as more surgent and lower in effortful control. One possible mechanism for these sex differences may be that boys and girls may respond to stress differently (Stroud, Salovey, & Epel, Reference Stroud, Salovey and Epel2002). Specifically, females are more likely to respond to challenge by focusing more on managing interpersonal relationships (building alliances and calming others) whereas males, when faced with stress, tend to engage more in risky behaviors (Booth, Granger, & Shirtcliff, Reference Booth, Granger and Shirtcliff2008). As a case in point, Shirtcliff, Granger, Booth, and Johnson (Reference Shirtcliff, Granger, Booth and Johnson2005) found that lower basal cortisol levels predicted higher externalizing problems for boys, but not for girls. In addition to these behavioral mechanisms, we may not have been able to detect a relationship between cortisol reactivity and EFs for girls because females are more likely to show increases in autonomic responses to stress as opposed to males who are more likely to show increased cortisol to stress (Kudielka & Kirschbaum, Reference Kudielka and Kirschbaum2005). Furtermore, bodies of females evidence stress in different ways than do the bodies of males (even though the levels of stress might be comparable; Kudielka & Kirschbaum, Reference Kudielka and Kirschbaum2005). Thus, boys' and girls' stress responses may have different implications for their social functioning and possibly also for their cognitive functioning. Future research may explore implications of these sex-specific associations in the context of prenatal stress exposure.
Limitations and future directions
It is important to note several limitations of the present study. First, as in all human studies investigating prenatal stress or mood disturbances, where randomizing exposure is not possible, we cannot speak to the causal effects of prenatal maternal mood on child behavior. However, as we took care to control statistically for postnatal maternal mood (as commonly done in this field), our findings support the concept of fetal programming, namely, that some of the risk is conferred prenatally from mother to child. Nevertheless, potential confounding by genetic influences cannot be ruled out completely. Rice et al. (Reference Rice, Harold, Boivin, van den Bree, Hay and Thapar2010), for instance, leveraged in vitro fertilization to tease apart genetic and putative prenatal stress effects on offspring development and reported results suggesting offspring attention-deficit/hyperactivity disorder (ADHD) may be due to shared inheritance of characteristics, rather than prenatal stress effects of the in utero environment. It remains questionable, however, to what extent these findings translate to EFs deficits following prenatal stress exposure. Cognitive deficits associated with ADHD are usually thought of in terms of poor EFs (Johnson, Reference Johnson2015), but as other crucial neuropsychological factors also contribute to the complex symptoms of this developmental disorder (Pauli-Pott & Becker, Reference Pauli-Pott and Becker2011), caution is warranted when translating ADHD findings to EFs outcomes.
Second, and related to the issue of causality, we recognize that by using mediational models, the mediator is presumed to cause the outcome and not vice versa (cf. reverse causation; Cole & Maxwell, Reference Cole and Maxwell2003). However, in our case, the timing of the measurement of the mediator (HPA axis activity) and outcome (EF performance) was not optimal; that is, the two variables were measured at the same time point (at 6 years). However, given our strongly theoretically based hypotheses, reverse causal effects appear unlikely. Nevertheless, future work with our cohort where we will be able to measure the outcome after the mediator will improve the strength of our conclusions.
Third and finally, it is important to bear in mind that no measurement of EFs is perfect. Likewise, cortisol is an imperfect measure of level of stress, especially in females (Kudielka & Kirschbaum, Reference Kudielka and Kirschbaum2005). Furthermore, the same experience is differentially stressful to different persons; and the cognitive testing (EFs tests and Kaufman Brief Intelligence Test) may have been stressful for some children but not others, hence providing an imperfect baseline. The fact, however, that we assessed baseline cortisol levels with three saliva measures (one upon arrival to the lab) strengthens our conclusions. As to the recovery saliva measure taken at home after the lab visit, we are not aware of any other study applying such a measure in combination with lab-based stress reactivity. Unfortunately, no perceived stress state of child concurrent with time of home recovery sample was assessed, so we cannot determine the emotional/arousal state the child was in when this last saliva sample was taken. Based on previous research showing lower stress reactivity evoked in home-based studies compared to childcare- and/or lab-based studies (Alkon et al., Reference Alkon, Goldstein, Smider, Essex, Kupfer and Boyce2003), we are though inclined to assume that our recovery saliva measure is not only a reliable indicator of stress recovery but also may actually maximize ecological validity of this study.
Despite these limitations, this study makes innovative contributions to the field of the developmental origins of psychopathology. It is a first step in the attempt not only to better understand how prenatal maternal mood shapes children's EF development but also to illuminate child/offspring HPA axis reactivity as a prominent mechanism in fetal programming. Future research may identify other mechanisms (e.g., autonomic nervous system responses, epigenetics, dopaminergic systems, or immune system and inflammation, such as the pro-inflammatory cytokines) through which children's, and maybe in particular girls’, EFs are affected by prenatal maternal mood.
Clearly, more work needs to be done to better understand how sex moderates the effects of prenatal stress on the development of stress responsivity and regulation. Future work may also gain critical insight by investigating whether altered EFs act as a mediator between early stress exposure and subsequent child outcomes. A recent study (Pearson et al., Reference Pearson, Bornstein, Cordero, Scerif, Mahedy, Evans and Stein2016), reported that prenatal anxiety was associated with impaired working memory in the offspring at age 8, which then mediated the effect of prenatal anxiety on math grades at age 16. Such findings may allow us to understand how exposure to prenatal stress affects children's functioning across different developmental domains.
Finally, research is needed to examine interactions of prenatal stress with postnatal environment and the child's genetic profile. Despite the popularity of the fetal programming model, it is also important to acknowledge that such effects are not necessarily permanent as animal research has shown that young animals show remarkable neuronal resilience if the stress is discontinued (cf. McEwen & Morrison, Reference McEwen and Morrison2013). Thus, while in these analyses we focused on prenatal maternal mood exposure and its associations with child stress regulation and EFs (i.e., while controlling for postnatal maternal mood), it is important to bear in mind that postnatal factors (e.g., postnatal maternal mood, parenting, secure attachment, and socioeconomic status) are important as well and may reverse the effects of prenatal stress exposure (or exacerbate them). The congruence between the demands of prenatal and postnatal environments may be a crucial factor. For instance, Sandman, Davis, and Glynn (Reference Sandman, Davis and Glynn2012) reported accelerated motor and mental development during the first year of life among infants whose mothers experienced congruent levels of depressive symptoms during and after pregnancy, even when the levels of symptoms were relatively high and the prenatal and postnatal environments were unfavorable. In this sense, prenatal environments prepare the fetus for postnatal life and confer an adaptive advantage for critical survival functions during early development. Furthermore, maternal prenatal and postnatal mental health problems may be differentially associated with later outcomes in the offspring. For instance, Vänskä et al. (Reference Vänskä, Punamäki, Lindblom, Tolvanen, Flykt, Unkila-Kallio and Tiitinen2015) showed that both maternal prenatal and postnatal mental health problems predicted children's later stress regulation, but in unique ways (maternal prenatal mental health problems predicted an intensified CAR, whereas mothers’ early postpartum mental health problems predicted a reduced CAR). Future research will hopefully explore in more detail the combination of early life stress, genetics, and ongoing stress that may ultimately determine an individual's responsiveness to stress and the vulnerability or resilience in developmental outcomes, such as EFs and psychiatric disorders (e.g., depression; Charney & Manji, Reference Charney and Manji2004).
Supplementary Material
To view the supplementary material for this article, please visit https://doi.org/10.1017/S095457941800041X.