As one of the quality indexes of oil products, alkaline nitride affects light stability, oxidation stability and additive sensitivity. Under photocatalysis, nitrides can be converted into coloured compounds, which directly affect the colour properties of oil products (Prado et al., Reference Prado, Rao and de Klerk2017). In the presence of a small amount of basic nitride, the solubility of chloride increases, which promotes chloride corrosion in the distillation column, thereby enhancing the corrosion rate compared to in the absence of alkaline nitride, which affects the stability of oil refining and processing (Sagues et al., Reference Sagues, Davis and Johnson1983). In addition, the combination of basic nitrides and acids in oil products may also cause various problems such as pipe blockage and tower corrosion.
At present, the commonly used denitrification processes in major refineries are clay refining and hydrofining (Ho, Reference Ho1988; Bej et al., Reference Bej, Dalai and Adjaye2001; Furimsky & Massoth, Reference Furimsky and Massoth2005; Baia et al., Reference Baia, Souza, de Souza, Veloso, Chiaro and Figueiredo2017). The former makes use of the strong adsorption capacity of clays and their large specific surface areas to adsorb basic nitrides; this is a low-cost but environmentally unfriendly approach. The latter process can achieve removal by transforming nitride through hydrogenation. However, mild hydrogenation cannot remove most nitrides, and increasing hydrogen pressure means high demand for hydrogen sources and high production costs. Moreover, in hydrofining, hydrodemetallization, hydrodesulfurization, hydrodeoxidization, hydrodearomatization and olefin saturation are all carried out in parallel with hydrodenitrogenation (Raje et al., Reference Raje, Liaw, Srinivasan and Davis1997; Prado et al., Reference Prado, Rao and de Klerk2017), which is not conducive to the denitrification and sulfur retention in lubricating oil. Furthermore, some researchers (Madgavkar & Washecheck, Reference Madgavkar and Washecheck1988; Qi et al., Reference Qi, Yan, Su, Qu and Dai1998; Feng, Reference Feng2004; Hernández-Maldonado & Yang, Reference Hernández-Maldonado and Yang2004; Huh et al., Reference Huh, Zazybin, Palgunadi, Ahn, Hong and Kim2009; Asumana et al., Reference Asumana, Yu, Guan, Yang, Zhou and Chen2011; Dharaskar Swapnil, Reference Dharaskar Swapnil2012; Han et al., Reference Han, Li, Cao, Zhai and Yuan2013; Chen et al., Reference Chen, Yuan, Abdeltawab, Al-Deyab, Zhang, Yu and Yu2014; Martínez-Palou & Luque, Reference Martínez-Palou and Luque2014; Laredo et al., Reference Laredo, Likhanova, Lijanova, Rodriguez-Heredia, Castillo and Perez-Romo2015; Ali et al., Reference Ali, Yang, Fine, Jin, Zhang, Xing and Ren2016; Hizaddin et al., Reference Hizaddin, Hadj-Kali, Ramalingam and Hashim2016) have proposed processes such as solvent extraction, complex denitrification and the use of ionic liquids, all of which have achieved varying degrees of success.
In general, the liquid–liquid extraction process is achieved in two ways: in the first one, physical extraction is achieved by using the various levels of solubility of basic nitrides in various solvents; and in the second one, the extractant reacts with basic nitrides and selectively dissolves the reaction product to achieve separation. The extraction process is limited by the properties of the solvent, including its boiling point, volatility, solubility, density, polarity, acidity, alkalinity or other chemical properties. Most of the liquid–liquid extraction processes are used in oil products at room temperature, and their denitrification rates are quite different from those of solvents under various conditions. Polar solvents (such as metal salt solutions), acidic solvents, ionic liquids and eutectic solvents have all been proved to be effective extractants, and the latter two have recently become the focus of research because they are environmentally friendly, highly efficient and can be customized (Madgavkar & Washecheck, Reference Madgavkar and Washecheck1988; Qi et al., Reference Qi, Yan, Su, Qu and Dai1998; Feng, Reference Feng2004; Hernández-Maldonado & Yang, Reference Hernández-Maldonado and Yang2004; Huh et al., Reference Huh, Zazybin, Palgunadi, Ahn, Hong and Kim2009; Asumana et al., Reference Asumana, Yu, Guan, Yang, Zhou and Chen2011; Dharaskar Swapnil, Reference Dharaskar Swapnil2012; Han et al., Reference Han, Li, Cao, Zhai and Yuan2013; Chen et al., Reference Chen, Yuan, Abdeltawab, Al-Deyab, Zhang, Yu and Yu2014; Martínez-Palou & Luque, Reference Martínez-Palou and Luque2014; Laredo et al., Reference Laredo, Likhanova, Lijanova, Rodriguez-Heredia, Castillo and Perez-Romo2015; Ali et al., Reference Ali, Yang, Fine, Jin, Zhang, Xing and Ren2016; Hizaddin et al., Reference Hizaddin, Hadj-Kali, Ramalingam and Hashim2016).
Adsorption is also an important denitrification process, and clays are the most commonly used adsorbents. Adsorption may be either physical or chemical, with the latter usually being more selective but also posing a challenge to the recovery of adsorbents because of the easily modified structures and large specific surface areas of clays, especially in the case of smectites (Fig. 1). In addition to clays, silica gels, ion-exchange resins, activated alumina, zeolites, activated carbon and solid acids have all been used for denitrification at lower temperatures (Wang & Li, Reference Wang and Li2000; Feng, Reference Feng2004; Sano et al., Reference Sano, Choi, Korai and Mochida2004; Xie et al., Reference Xie, Favre-Reguillon, Wang, Fu and Lemaire2010; Hong & Tang, Reference Hong and Tang2015).
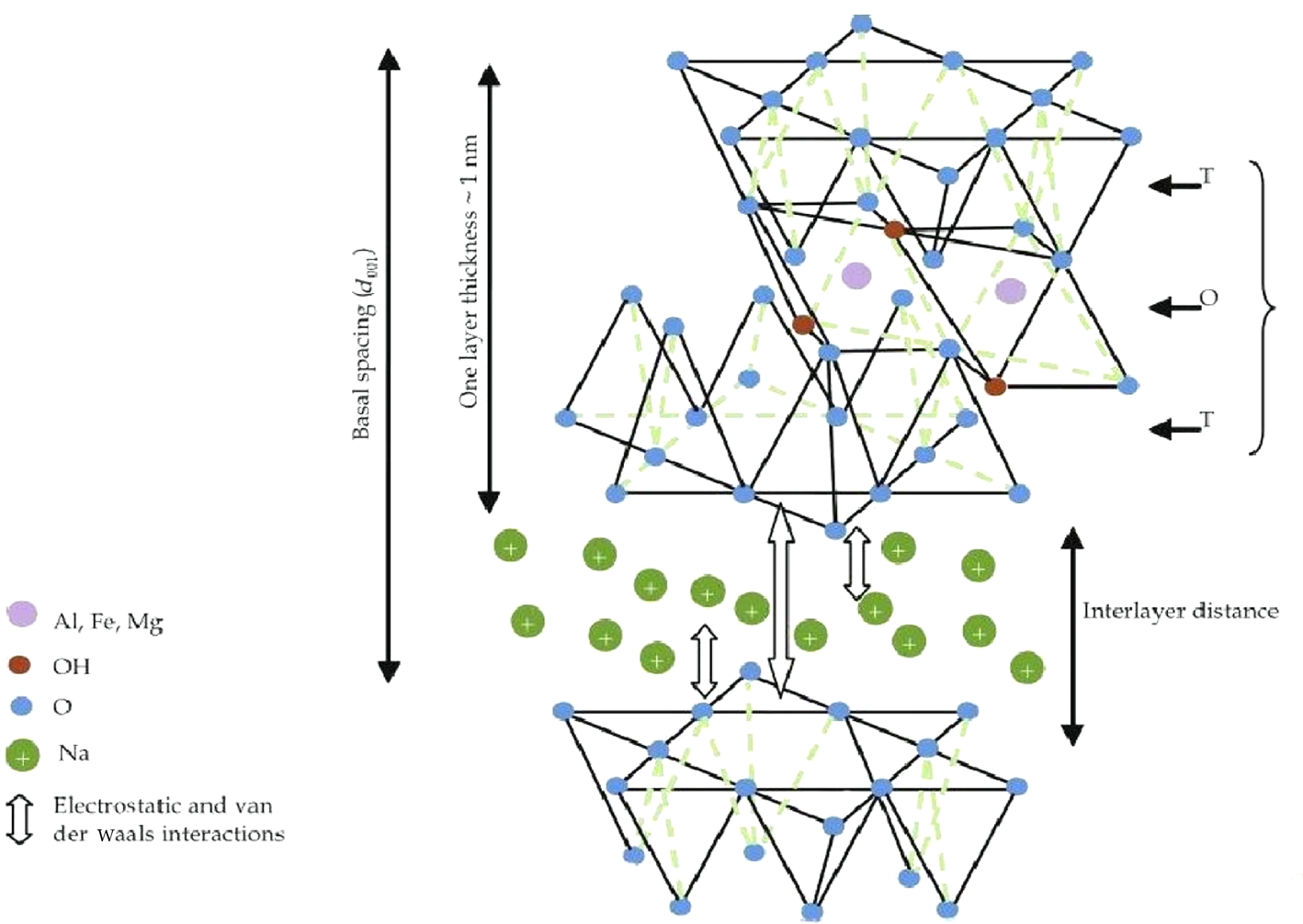
Fig. 1. The structure of smectite clay.
Clays have not been replaced by the emerging or conventional adsorbents because of their low cost and wide range of applications. Compared with liquid–liquid extraction, the technological process of adsorption and deamination is simpler and the reaction temperature and application conditions are less strict. In addition, the effect of Lewis acids (i.e. metal salts) on the removal of basic nitrides at low temperatures is also significant (Choi & Dines, Reference Choi and Dines1985; Feng, Reference Feng2004; Zhang et al., Reference Zhang, Xu, Qian and Liu2013).
The denitrification process of the feedstock oil used in this experiment is clay refining at 180°C, with a basic nitride removal rate of ~20–30%. At such a low denitrification rate, the amount of waste clay produced every year is considerable for large refineries, causing tremendous environmental impacts.
This work compares the denitrification efficiency of three bentonitic clays and explores possible factors affecting the process. The bentonites come from various areas and are used as carrier precursors. The physical and chemical properties of the clays were studied. Clay-A, which had best denitrogenation efficiency, was selected, modified with FeCl3 and used to remove basic nitride at 180°C. Our study aims to develop an effective basic nitride adsorbent and to evaluate its removal mechanism.
Experimental
Materials and methods
The raw oil is a vacuum distillation, second-line oil from Sinopec Shanghai Gaoqiao Petrochemical Co., Ltd. (China) with an alkaline nitride content of 56.22 ppm. The three bentonites used are labelled as Clay-A, Clay-B and Clay-C, and they consist mainly of montmorillonite. All bentonites are Ca-rich. Clay-A comes from central China, Clay-B from northern China and Clay-C from north-central China. The bentonites were activated by Baiyue Active Clay Co., Ltd, Anhui (China). The mineralogical and chemical compositions of the clays are shown in Fig. 2 and Table 1. FeCl3, Fe(NO3)3, C7H8 and glacial acetic acid were purchased from Titan Technology Co., Ltd (China).

Fig. 2. XRD traces for the carrier clays.
Table 1. Chemical compositions (wt.%) of the bentonite clays.

A total of 50 g of crude oil was added to a three-necked flask with a magnetic rotor and placed in a heating mantle before connecting the electric thermocouple and the N2 pipeline (Fig. 3). After opening the N2 valve and adjusting the gas flow, the magnetic rotor began stirring and the reaction temperature was set to 180°C. Adsorbent was added according to mass ratios of agent to oil of 1:50 (clay or modified clay) or 1:100 (pure compound). After 30 min of reaction, the oil was filtered and 10 g of residual crude was weighed for chemical titration.

Fig. 3. Schematic diagram of a lubricating oil denitrification unit. 1 = constant-temperature magnetic stirring and heating sleeve; 2 = thermocouple; 3 = condensing tube; 4 = magnetic rotor.
Clay modification
The three bentonite clays were dried at 150°C for 2 h and ground in a ball mill for 6 h at 300 rpm. Clay-A was further modified with FeCl3 as follows: three aliquots with masses of 0.99, 0.95 and 0.9 g were prepared and 0.01, 0.05 and 0.10 g FeCl3 were added, respectively. This was then dried at 120°C, roasted at 150°C for 2 h and ground to obtain 1 g of Clay-A modified by 1, 5 and 10 wt.% FeCl3, respectively.
Determination of the basic nitride content
The basic nitride content was determined via chemical titration using perchloric acid–glacial acetic acid as the titrant and methyl purple as an indicator. The end of titration was marked by the change of the residual oil from purple to blue or from yellow to light green. Measurements were taken in duplicate and the average value of the two results was taken as the final result of denitrification. The differences between the two measurements never exceeded 1 ppm.
N2 adsorption–desorption characterization
A clean and dry quartz tube was used to record the mass of the empty tube. In total, ~0.1 g of clay powder was loaded into the quartz tube, which was connected to a JWGB ZQ-200C specific surface analyser (Beijing Jingwei Gaobo Co., Ltd, China). The clay was outgassed at 150°C for 3 h in a vacuum and the specific surface area was determined in a liquid nitrogen environment.
Particle-size characterization
A small amount of clay powder was dispersed in deionized water and the particle-size distribution was analysed using an LA960 laser particle size analyser (Horiba Trading Co., Ltd, China).
Fourier-transform infrared spectroscopy
A total of 16.6 mg of dried clay powder was pressed into 13 mm discs under 10 MPa, placed in an in situ infrared cell at 10–5 Pa at 200°C for 2 h, then cooled to 80°C for 30 min and finally heated again at 200°C for 15 min to induce desorption. The Fourier-transform infrared (FTIR) spectra were obtained as the averages of 32 scans using an IS-10 FTIR spectrometer (Thermo Fisher Scientific, MA, USA).
X-ray diffraction characterization
The mineralogical compositions of the bentonite samples were determined using a Tonda 5000 X-ray diffractometer with Cu-Kα radiation (λ = 0.154 nm) at 40 kV, 30 mA, in the range 3–50°2θ, using a curved graphite monochromator. The scanning rate was 0.02°2θ.
Scanning electron microscopy
The morphology of the smectite particles was determined using scanning electron microscopy (SEM) with an FEI Nova NanoSEM 450 instrument (Thermo Fisher Scientific, MA, USA). Samples were deposited on conductive carbon scotch tape and covered with a gold film 20 nm thick. The SEM images were obtained in the secondary electron mode at a beam energy of 18 keV.
Results and discussion
Influence of the physical and chemical properties of the bentonite clays
Clay-A displayed a good ability to remove alkaline nitride; therefore, it was selected as the carrier clay for further modification (Fig. 4). Based on the difference in alkaline nitride removal capacity of the various clays, we investigated their specific surface data and particle-size distribution data.

Fig. 4. Alkaline nitride-removal abilities of the bentonite clays. Agent/oil ratio = 1:100, temperature = 180°C, reaction time = 30 min, N2 atmosphere.
There are no direct relationships between the denitrification capacity and the specific surface area, pore volume and pore diameter (Table 2). The adsorption and desorption curves of the three bentonites are all type IV curves, with H4-type hysteresis in the high-relative-pressure section, which is in accordance with the clay structure (Fig. 5). The type IV adsorption isotherm curve indicates initial monolayer adsorption on the mesopore walls followed by capillary condensation when the gas pressure is less than the liquid saturation pressure, forming a liquid phase-like state. After the capillary condensation fills the mesopore, due to the large pore size or strong interaction with the adsorbate the multi-molecular layer adsorption continues to occur and finally forms the adsorption saturation platform. Due to the capillary condensation on the mesopores, the adsorption and desorption curves do not coincide with each other at relative pressures >0.4, resulting in a hysteresis loop. The H4 hysteresis loop means that there is no obvious adsorption platform in the isotherm and that the pore structure of the material is irregular, which is the result of the mixing of pores of various sizes. The three clays displayed similar N2 adsorption–desorption isotherms (Fig. 5).

Fig. 5. Isothermal adsorption and desorption curves of the bentonite clays. STP = standard temperature and pressure.
Table 2. Specific surface data of the clays from various ore belts.

The particle-size data of the clays are listed in Table 3. Clay-A, with its mid-level particle size, has the greatest denitrification capacity (Table 3). Particle size does not affect directly the capacity of the bentonites to remove alkaline nitride.
Table 3. Particle sizes of the bentonite clays.

a The diameter of particles when the transmittance of particles reaches 50% in tests using a laser particle sizer.
b The arithmetic mean of the particle-size distribution.
c The geometric mean of the particle-size distribution.
In summary, the physical structure and properties of the clays have no direct effect on denitrification capacity; hence, the chemical properties were considered. Due to the existence of acid–base neutralization in the denitrification process, the acidities of the clays were investigated.
Both the total acidity and the Lewis acidity seem to improve the clays’ ability to remove alkaline nitride (Fig. 6 and Table 4). The relationship between Brønsted acidity and denitrification ability is not clear, however, and we cannot rule out the possibility that a lower Brønsted acidity may also affect denitrification. Previous work (Choi & Dines, Reference Choi and Dines1985; Qi et al., Reference Qi, Yan, Su, Qu and Dai1998; Zhang et al., Reference Zhang, Xu, Qian and Liu2013) has shown that there are several processes for denitrification using the acid solutions and metal salts as extractants and complexing agents, so the pure Brønsted acid and Lewis acid components were further investigated regarding their ability to remove alkaline nitride at high temperatures.

Fig. 6. Surface acidities of the bentonites.
Table 4. Surface acidities of the bentonites.

Influence of ions
Various Brønsted acids (including oxalic acid, citric acid, tartaric acid, ethylenediaminetetraacetic acid, phosphotungstic acid and phosphoric acid) and Lewis acids (including ferric chloride, ferrous chloride, zinc chloride, copper chloride, cuprous chloride and aluminium chloride) were used for the removal of basic nitrides, and the results are presented in Fig. 7.

Fig. 7. Removal rates of alkaline nitride by Brønsted acids (left) and Lewis acids (right). Agent/oil ratio = 1:200, temperature = 180°C, reaction time = 30 min, N2 atmosphere. EDTA = ethylenediaminetetraacetic acid; H3Cit = citric acid; PTA = phosphotungstic acid; TA = tartaric acid.
Under the reaction conditions used, oxalic acid and citric acid (Brønsted acids) are unstable and prone to vaporization or decomposition, while FeCl3 and FeCl2 both have excellent ability to remove alkaline nitride (which is difficult to decompose and separate) compared with Brønsted acids.
Previous work has shown that there is an obvious relationship between Lewis acid density and electronegativity: with increasing electronegativity, Lewis acid density increases. Brown & Skowron (Reference Brown and Skowron1990) summarized two effective correlations between Lewis acid density and electronegativity:


Equation 1 was proposed by Allen (Reference Allen1989). The electronegativity (χ) is calculated using the electron energy correlation constants of the s and p valence layers, and then the Lewis acid density (Sa) of the main-group elements is obtained. Equation 2 was put forward by Brown (1990) after observing the oxidation state (V) and coordination number (N t) of the ions in a compound. By using the above two formulae, the Sa value of the compound can be obtained and the Lewis acid strength can be compared.
The electronegativity of elements of the same group decreases with increasing atomic number, and the electronegativity of elements of the same period increases with increasing atomic number. For various oxidation states of the same atom, the greater the valence state, the stronger the electronegativity. In general, especially in the main-group elements, electronegativity is consistent with Lewis acid density, although some special cases were pointed out by Brown (1990). The transition elements have comparable electronegativity. Various scales and calculation methods have been proposed to characterize the Lewis acid density, which involve nuclear charges and atomic/ion radii.
The electronegativity of ions as proposed by Li & Xue (Reference Li and Xue2006) includes the sixfold coordination radius (ri), the limit ionization energy (Im) and the effective principal quantum number (n*) of ions, and R = 13.6 eV is the Rydberg constant. Im is related to the effective charge number and the effective principal quantum number. Based on Equation 1, the Lewis acidity of various ions can be calculated easily from Equation 3.

Based on the above calculation and analysis, it follows that the Lewis acid density of Fe3+ is greater than those of Cu2+ and Zn2+, which explains why FeCl3 shows the greatest denitrification effect in various Lewis acids, followed by FeCl2 (Zhang, Reference Zhang1982). Although AlCl3 is the most commonly used high-acidity compound in industry, it is not as effective as FeCl3 in denitrification reactions because it is easy deactivated and lost. In addition, the distribution of valence electrons in the external valence layer of Fe ions provides a more stable environment for binding the lone-pair electrons of basic nitrides, further explaining the excellent denitrification effect of FeCl3.
Based on the high removal rate of Fe3+, the denitrification ability of various iron salts was investigated. Sagues et al. (Reference Sagues, Davis and Johnson1983) noted that the solubility of chloride increases in the presence of basic nitrides, which may pose a potential risk to pipelines and equipment. Because the structure and properties of FeBr3 are similar to FeCl3 but it is slightly less corrosive, the denitrification ability of FeBr3 was also investigated. In addition, because H3PO4 also causes denitrification at high temperatures, the role of FePO4 was also considered. Furthermore, due to the principle of the denitrification and sulfur preservation in the processing of lubricating oil, Fe2(SO4)3 was also included in this investigation. Finally, Fe(NO3)3 was used as a supplement to verify the effect of acid ions and nitrogen-containing compounds on the denitrification efficiency of lubricating oil.
The denitrification abilities of FeCl3 and FeBr3 are strong, but the performance of FeCl3 is better at 180°C (Fig. 8). It follows that the factors affecting the denitrification effects of various iron salts are the acidity and basicity of the anions and the ion radius, and this is consistent with the influence of electronegativity mentioned previously and its contribution to Lewis acid density. The alkalinity of NO3– is strong, and the residual amount of basic nitride measured at the end of the reaction is greater than that of the raw material. While the ion radii of SO42– and PO43– are larger than those of Cl– and Br–, the electron cloud is widely distributed and the repulsion of nitride lone-pair electrons is strong, leading to a weak electronegativity, which affects the binding of Fe ions and nitrides. On the other hand, the atomic structure and properties of Cl– and Br– are similar, but the radius of Cl– is smaller than that of Br–, causing greater electronegativity and Lewis acid density in Cl–; hence, the binding force of nitrides to Fe ions is stronger and the possibility of dissociation at high temperatures is lower, yielding better denitrification. This result is in accordance with the work of Luo & Benson (Reference Luo and Benson1991), who showed that the Lewis acid density of Cl was significantly greater than that of Br.

Fig. 8. Denitrification effects of various Fe salts. Agent/oil ratio = 1:200, temperature = 180°C, reaction time = 30 min, N2 atmosphere.
Based on this, FeCl3 was loaded onto Clay-A as an active component and the denitrification effect was investigated. Compared with the carrier clay, the ability to remove alkaline nitride was improved after FeCl3 modification (Fig. 9). However, when increasing the FeCl3 loading beyond 1%, denitrification did not improve further. It is suggested that such a large Fe load caused aggregation of montmorillonite crystals. X-ray diffraction (XRD) traces of Clay-A treated with various amounts of FeCl3 are shown in Fig. 10.

Fig. 9. Denitrification effects of clays under various FeCl3 load concentrations. Agent/oil ratio = 1:100, temperature = 180°C, reaction time = 30 min, N2 atmosphere.

Fig. 10. XRD traces for the clays with various Fe loads.
At an FeCl3 load of 1%, no significant changes are observed in the bentonite, suggesting an improved dispersion effect with FeCl3. Hematite formed with increasing FeCl3 loading, and at 10% FeCl3 loading hematite became a major phase. It can be inferred that the dispersion effect is lower in this case than with a low Fe load, causing slow denitrification.
In order to verify the XRD results and the surface species aggregation and morphology changes caused by the increase in FeCl3 load, a SEM analysis was performed, and the results are shown in Fig. 11. When there is no FeCl3 load or at 1% FeCl3 loading, the morphology of the adsorbent tends to be similar and the montmorillonite particles tend to be better dispersed. At FeCl3 loads of 5% or 10%, the surface morphology of the adsorbent changed. Most of the surface of the carrier was covered by FeCl3, which affected the distribution of active species. This explains the fact that a large Fe load does not improve denitrification ability. The SEM images are in accordance with the XRD results.

Fig. 11. SEM images of (a) Clay-A, (b) 1% FeCl3/Clay-A, (c) 5% FeCl3/Clay-A and (d) 10% FeCl3/Clay-A.
The pyridine-FTIR spectra of Clay-A with 1% FeCl3 loading are shown in Fig. 12. Total acidity increases at 1% FeCl3 loading (Table 5). In addition, the acid value and chlorine content of the oil treated with clays loading with 1% FeCl3 did not exceed the maximum acceptable values at 180°C.

Fig. 12. Surface acidities of Clay-A (0%/1% FeCl3) as assessed from FTIR spectra.
Table 5. Acid content of Clay-A with 0%/1% FeCl3.

Denitrification mechanism
The pure FeCl3 and post-reaction precipitation were examined using FTIR spectroscopy with the KBr technique. New bands appeared in the 3000–2800 and 1500–1300 cm–1 regions of the complex product compared with FeCl3 (Fig. 13). The bands at 3070 and 3020 cm–1 are attributed to C–H stretching in pyridine and that at 3030 cm–1 is attributed to quinoline and aniline. By comparing the spectra before and after the reaction with the spectra of basic nitrides, a red shift can be seen, as the C–H band of basic nitrides shifts from 3100–3000 to 3000–2900 cm–1. It is suggested that when Fe3+ adsorbs basic nitrides, it coordinates with them, and this coordination would occur according to Equation 4:

where n and m refer to any number of the basic nitrides and Cl– that take part in the complexation reaction and R refers to the alkyl of the basic nitrides.

Fig. 13. FTIR spectra of pure FeCl3 and post-reaction precipitation.
Conclusion
A Chinese bentonite showing good denitrification performance was selected as a carrier for oil denitrification treatment and 1% FeCl3 was added to modify the clay. Under the reaction conditions, the denitrification rate increased from 33.6% to 43.4%. Through characterization and analysis of the clay, some conclusions can be inferred:
(1) The physical structure of the clay does not affect denitrification performance. Nevertheless, the acid content of the clay influences denitrification performance.
(2) Compared with Brønsted acids, Lewis acids are superior for removing alkaline nitride, and FeCl3 is efficient under the specific reaction conditions used in this study.
(3) The vibration bands of basic nitrides red-shifted from 3100–3000 to 3000–2900 cm–1, indicating a strong combination between basic nitrogen and Fe3+, with the binding mode being complex.
In a subsequent experiment, the specific species of basic nitrides in lubricating oil and the various adsorptions of specific nitrogen species by FeCl3 will be considered.
Financial support
This work was supported by the National Natural Science Foundation of China (No. 21808054).